- Department of Hand Surgery, China-Japan Union Hospital of Jilin University, Changchun, China
Peripheral nerve injury (PNI) is one of the most common neurological diseases. Recent studies on nerve cells have provided new ideas for the regeneration of peripheral nerves and treatment of physical trauma or degenerative disease-induced loss of sensory and motor neuron functions. Accumulating evidence suggested that magnetic fields might have a significant impact on the growth of nerve cells. Studies have investigated different magnetic field properties (static or pulsed magnetic field) and intensities, various magnetic nanoparticle-encapsulating cytokines based on superparamagnetism, magnetically functionalized nanofibers, and their relevant mechanisms and clinical applications. This review provides an overview of these aspects as well as their future developmental prospects in related fields.
1. Introduction
Peripheral nerve injury (PNI) is a major clinical concern, which is caused by the loss of structure or function of peripheral nerves. In developed countries, ~ 13–23 per 100,000 persons are affected by PNI every year (1). It is a common complication in trauma. The regeneration of the peripheral nerve should be improved to recover its function. The core difficulties in nerve regeneration are the directional prolongation of neurites and the proliferation of Schwann cells (SCs), which provide well-functional axons and myelin sheaths. Currently, the studies on peripheral nerve regeneration involve growth factors, nerve conduits, tissue engineering, and genetic engineering (2–5). Since the 1980's, researchers have been continuously studying the effects of magnetism on nerve cells. The magnetic field can induce the orientation of cellular growth. In 1965, Murayama et al. reported that sickled erythrocytes were oriented perpendicular to the magnetic field (6). This was the first study on this phenomenon. Since then, erythrocytes, collagen, fibroblasts, osteoblasts, human glioblastoma A172 cells, SCs, smooth muscle cells, and PC12 cells have been reported to be related to magnetic fields (6–13). In addition, nerve cells have a better growth potential in the magnetic field [16]. Based on the theoretical effects of magnetic fields on nerve cells, biomaterial interventions have shown promising results in cell cultures and animal studies, providing contact guidance for extending neurites or a sustained release of various drugs and growth factors. Moreover, researchers further investigated the magnetic stimulation of peripheral nerves to obtain a better sense and function. This review summarizes the recent studies on the effects of magnetism on peripheral nerves and proposes future developmental directions in this field.
2. PNI and regeneration
PNI is a common clinical issue worldwide, and its prognosis varies with the degree of injury. Unlike the central nerve, the peripheral nerve has a limited regenerative capacity after being injured. After PNI, Wallerian degeneration occurs; the cell bodies of neurons swell, dissolving the chromatin and making Nissl's body disappear, which makes the cell body relatively eosinophilic, shifting the nucleus to the periphery. Moreover, SCs and macrophages phagocytize the myelin and axons, thereby degenerating the myelin sheaths at the distal portion of the nerve injury site. In addition to clearing myelin debris, macrophages and SCs also produce cytokines, which enhance axon growth. After clearing debris, regeneration begins at the proximal portion and continues toward the distal stump. New axonal sprouts generate from the Ranvier nodes (14, 15).
Different cells participate in repairing the injured nerves. SCs are arranged longitudinally to form the bands of Büngner, guiding the direction of regeneration and providing a microenvironment, which promotes regeneration. The tips of the regenerated axons, called growth cones, are composed of flat sheets of cellular matrices with finger-like protrusions (filopodia). Actin polypeptides, which can contract to produce axonal elongation, are in filopodia. The growth cones release proteases, which dissolve the matrix on its path, thereby clearing the way for regeneration. The recovery of nerve function depends on the extension of regenerated axons from the injured site to the target organ at the distant portion (14).
3. Theoretical study based on magnetism and peripheral nerve regeneration
3.1. Properties of magnetic fields and superparamagnetism
Magnetic properties are generated by electrons, spinning around the nuclei of atoms and their axis (16). The magnetic field intensity of a magnetic material is related to its atomic structure and temperature. In some atoms, the magnetic dipoles, arising from the spinning of electrons, do not cancel each other out, thereby creating a permanent dipole. The permanent dipole aligns with the external magnetic field. The magnetism of superparamagnetic materials disappears with the disappearance of the external magnetic field. This provides a basis for strengthening the magnetic field intensity and reducing the adverse effect of a magnetic field (17).
3.2. Growth of nerve cells in a magnetic field
Static magnetic fields (SMFs) or pulsed magnetic fields (PMFs) are usually used in the studies of nerve cells. PMFs are safe and efficient to promote nerve regeneration (18–20). SMFs have constant intensity and direction and a frequency of 0 Hz. Recently, studies have been conducted on the effects of SMFs on nerves at the cellular level. Numerous studies showed that the effects of exposure to SMFs on cellular proliferation varied depending on the cell type (21, 22). Moreover, most studies, investigating the effects of SMFs on cell cycle distribution, showed that there was no statistical difference between the exposed and control group (23). There are two necessary pathways for the regeneration of peripheral nerves: the growth of neurites and myelin sheaths. Mann et al. provided new ideas for the correlations between these two enhanced pathways (24). They reported that a slight nuclear magnetic resonance therapy (NMRT) could induce an increase in SCs proliferation; however, its effect on repair-associated genes was not statistically significant. Furthermore, NMRT could not only enhance the neuronal maturation of individual neurons but also encouraged neurite outgrowth. Interestingly, they also showed that NMRT could induce the secretion of neurotrophic and neuritogenic factors in SCs, leading to the survival of dorsal root ganglia (DRG) neurons and neurite outgrowth.
These studies suggested that the differences in growth direction in response to magnetic field direction depended on the cell type. Macias made two coils of individual copper sheets folded into a square coil to establish a PMF (25). DRG showed a growth tendency parallel to the electric field (perpendicular to the magnetic field). Interestingly, Eguchi showed that SCs and collagens exhibited different growth directions under strong SMFs (8-T). The directions of the SCs and collagen groups were parallel and vertical to that of the magnetic field, respectively. Moreover, SCs oriented in the direction vertical to the magnetic field in a mixture of Schwann cells and collagen under 2 h of magnetic field exposure. DRG showed the same growth tendency as that of collagens, thereby providing theoretical support for the use of a magnetic field to guide peripheral nerve regeneration. Other studies demonstrated that fibrin (26, 27), PC12 cells (12), erythrocytes (28), and osteoblasts (9, 29) aligned parallel to the magnetic field. This property of the magnetic field might be significant in selecting different ways to guide peripheral nerve regeneration.
A strong magnetic field has a destructive effect on cells and can cause genetic mutation and DNA damage (30, 31). Liu et al. reported that the high-intensity magnetic field might be unfavorable for the growth of Schwann cells. When PMF was set to a frequency of 50 Hz, different from 5.0 or 10.0 mT (T = tesla), 0.5, 1.0, or 2.0 mT each was safe for the growth of Schwann cells (32). Interestingly, Eguchi reported that SCs and collagen exhibited normal growth under strong SMFs (8-T) (11). The reasons for these dramatic differences have not been explored yet, and it was speculated that the effects of magnetic field intensity on cellular growth were related to the type of magnetic field. SCs could better withstand SMF as compared to PMF.
3.3. Magnetic nanoparticles
A major challenge in peripheral nerve regeneration is the local and sustained delivery of bioactive factors. Studies on MNPs showed that the intervention of an external magnetic field was a type of conventional means. Due to good biocompatibility, MNPs can also be used alone as drug-loading tools to promote peripheral nerve regeneration (33). Magnetic liposomes could enhance neurite outgrowth with a nerve growth factor (NGF) through phosphorylated extracellular regulated protein kinases (ERK) 1/2, which simultaneously upregulated the β-tubulin and integrin β1 (34). Hu et al. showed an increased extension of spiral ganglion neurons (SGNs) in MNPs and MNPs + magnetic field groups as compared to those in the control group (2). Mitrea et al. used oral chitosan-functionalized MNPs to promote neural regeneration in the PNI experimental model and obtained satisfactory results (35). The mechanism for this phenomenon might be the release of Fe ions from iron oxide NPs inside the cells, promoting neurite outgrowth. Numerous approaches used MNPs to assist in drug or gene delivery, including liposome or micelle encapsulation, polymer coatings, and direct surface functionalization (16). Other applications of MNPs include the directional migration of cells induced by external magnetic fields (2, 36–39). In a review, Gilbert divided MNP-mediated cellular manipulations into two categories: magnetic cell guidance and magnetic cell transplantation (16). In essence, both these methods are based on the combination of MNPs and target cells induced by external magnetic fields.
To solve the problem of instability, aggregations, and cellular toxicity of MNPs, Qin et al. synthesized a novel nanomedicine composed of NGF-functionalized Au-coated MNPs. They showed that both the static and rotation magnetic groups could significantly increase the number of differentiated cells and neurites, exhibiting longer average neurite length and clearer directionality, as compared to the no magnet group (36). Liu et al. designed a type of fluorescent–magnetic bifunctional superparamagnetic iron oxide nanoparticles (SPIONs) to control the phenotypic stability of repaired SCs (37). The SMF-induced SPIONs group showed a significantly higher mRNA expression level of brain-derived neurotrophic factor (BDNF), glial cell-derived neurotrophic factor (GDNF), oligodendrocyte transcription factor 1 (Olig1), and vascular endothelial growth factor (VEGF) (qRT-PCR and ELISA). Western blot analysis showed that the expression levels of Beclin1 and lc3b in the experimental group were higher than those in the control group, while the expression level of p62 protein was lower, suggesting the activation of autophagy in Schwann cells by the magnetic stimulation SPIONs. Moreover, for their effects on elongation and branching, there was a statistically significant increase in the expression levels of immune-related cytokines and transcription factors associated with repair phenotypes in the SPION + MF groups. In vitro experiments also verified the effects of SPIONs on promoting peripheral nerve regeneration. Hu et al. reported that the combination of SGNs with poly-L-lysine-coated SPIONs could promote the extension of neurites and growth along the direction of a magnetic field (2). Huang et al. reported that the number of SCs with ChABC/PEI-SPIONs, which migrated into the astrocyte region, was 11.6- and 4.6-fold higher than that of control groups under the driven effect of a directional magnetic field (40). Raffa et al. observed that PC12 cells also exhibited more neurite extension and orientation under the guidance of MNPs (39).
3.4. Biomaterials based on magnetic fibers
Due to the advantageous features of nanofibers and MNPs, numerous researchers have combined MNPs with biodegradable nanofibers to produce magnetic nanofiber scaffolds. Nanofibers have the characteristics of a large surface-to-mass ratio, high porosity, and superior mechanical performance (41–43). Based on electrospinning (ES), there are three techniques for obtaining iron oxide-loaded composite fibers: the introduction of pre-synthesized SPIONs in a polymer solution before ES (used in biodegradable polymers mostly), mixing a precursor before a post-ES process to yield the SPIONs within the fibers, and in situ synthesis techniques (44, 45). There are two major drawbacks in the classical precipitation of NPs on the surface of biomaterials, including difficulty in controlling the morphology and aggregation of NPs and the incompatibility of SPIONs formation with a wide range of biopolymers (44). Silanization, polymer brush coating, and grafting have been used for ameliorating the dispersibility of NPs in polymeric matrices (44). Sodium citrate, polyacid, or oleic acid has also been applied for the dispersion of iron oxide NPs. Nottelet et al. combined thiolyne photoaddition with free ligand exchange to anchor SPIONs on the surface of nanofibers, which maintained the initial fiber morphology and avoided the unwanted aggregation of MNPs.
A variety of materials have been used in the preparation of magnetic nanofibers, such as chitosan/poly (vinyl alcohol) (PVA), poly (ε-caprolactone) (PCL), hydroxyapatite (HA), magnetic poly (L-lactic acid) (PLLA), poly (D, L-lactic acid) (PDLLA), and poly (glycolide-co-L-lactide) (PGLA) (46–50). Due to the tunability, porosity, hydrophilicity, capacity for the incorporation of biological factors, and the polymeric nature of hydrogels, they are increasingly used in bone or nerve regeneration, cancer therapy, and tissue engineering (51). Gilbert et al. designed a method to inject the small conduits of aligned fibers within a hydrogel to reduce fiber tangling (52). They observed that SPION could increase the elongation of neurites by 30%. In addition, they also concluded that SPION content in the electrospinning fibers could be set between 2 and 6% by weight to balance the magnetization and fiber diameter, alignment, and/or density. Gilbert et al. also synthesized aligned control PLLA and SPION-grafted PLLA electro-spun fibers to induce DRG growth. They set up three different types of magnetic fields to study the induction of nerve regeneration by magnetic nanofibers under different magnetic fields: SMF, alternating magnetic field, and linearly moving magnetic field. They observed that neurites could extend 24 and 30% farther on SPION-grafted fibers as compared to that of untethered SPIONs on PLLA fibers under the static or alternating magnetic field. Moreover, 40 and 27% longer neurites were observed in the alternating and linearly moving magnetic fields as compared to that in the SMF (53).
In addition to inducing nerve regeneration under a paramagnetic action, the magnetic field can also be used to form aligned columnar structures in biological scaffolds, especially systematically tuning the mechanical properties of hydrogel scaffolds (54–56). Schmidt et al. used polyimide, magnetic alginate microparticles, and glycidyl methacrylate hyaluronic acid to invent a magnetically aligned regenerative tissue-engineered electronic nerve interface (MARTEENI) (57). This new technology combined TEENI technology with magnetically templated scaffolds to overcome the challenges of prosthetic-limb neural-interfacing technology, such as stiffness mismatch, low axonal population sampling, and long-term signal decay. After 6–8 weeks of exposure, MARTEENI groups showed similar results in the expression levels of extracellular matrix (ECM) proteins and axonal density as compared to the autograft groups. Under the induction of an external magnetic field, magnetic nanofibers can accelerate drug release. Ming-Wei Chang et al. reported that magnetic hollow fibers could induce greater drug release as compared to the non-treated samples under AMF (~40 kHz) (58). This might be due to the magnetocaloric effect caused by superparamagnetism. This kind of nanofiber might have a good prospect in drug delivery with precise positioning.
3.5. Mechanism of magnetic effects on nerve cells
Since the 1970's, increasing evidence has confirmed the neuron-promoting effects of SMF and alternating magnetic fields. The mechanism of the magnetic field, including both external or micromagnetic fields based on superparamagnetism, which affects peripheral nerves, is unclear. A comprehensive understanding of the mechanism at the cellular level might help in developing strategies to use magnet-mediated therapy for PNI. This part of the review highlights the mechanism of stimulating the effects of a magnetic field on cells (Figure 1).
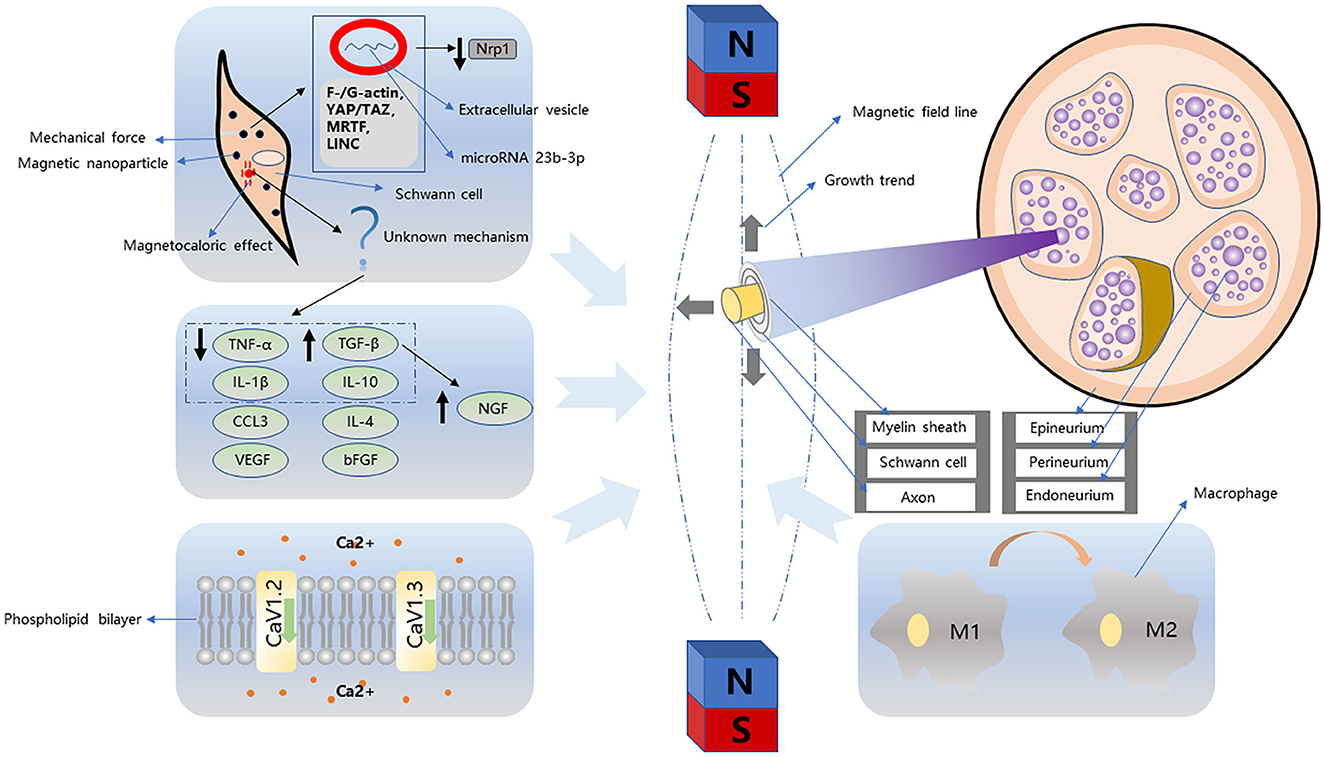
Figure 1. Magnetism-related nerve regeneration strategies can be divided into simple magnetic fields and magnetic nanoparticles. The growth trend of neurites and Schwann cells in the simple magnetic fields is different. Schwann cells tend to grow parallel to the magnetic field, while neurites tend to be vertical. In addition, this process is accompanied by macrophage transformation, cytokine regulation, and changes in ion channels. Strategies based on magnetic nanoparticles generally combine particles with Schwann cells. In this process, Schwann cells are subject to mechanical action and magnetocaloric effect. In the process of mechanical stimulation, Schwann cells are directionally proliferated in the magnetic field by F-/G-actin, YAP/TAZ, MRTF, LINC pathway, and the downregulation of NRP1 induced by microRNA 23b-3p proliferation.
One of the mechanisms of the magnetic field on cells is its effect on the molecular structure of excitable membranes, modifying the function of embedded ion-specific channels (59). Strong magnetic fields can alter the preferred orientation of different diamagnetic anisotropic organic molecules. Low magnetic field intensity relies on the molecular structure of excitable membranes. Researchers have focused on the changes in ion channels under a magnetic field. The voltage-gated channels, including potassium, sodium, and calcium ion channels, are affected by magnetic field exposure, thereby making neurons highly sensitive to magnetic field exposure (60–62). Numerous studies have investigated the internal flow of calcium ions (3, 63). In previous studies, Aldinucci simultaneously exposed human lymphocytes to 4.75 T SMFs and 0.7 mT PMF at 500 MHz for 1 h. The combined SMF and PMF groups could increase the Ca2+ influx (64). Balassa et al. reported that the calcium ion channels might play significant roles in synaptic functions under magnetic field stimulation (65). Finberg et al. reported that SMFs could mediate the Ca2+ influx through L-type voltage-gated calcium channels (VGCCs), providing a neuroprotective activity related to their anti-apoptotic capacity. They reported that the cellular apoptosis of primary cortical neurons, which were exposed to SMF (50 G), decreased, showing a lower expression level of pro-apoptotic markers, including cleaved caspase-3, cleaved poly ADP ribose polymerase-1, the phospho-histone H2A variant (Ser139), and active caspase-9. Moreover, the expression levels of Cav1.2 and Cav1.3 channels were significantly enhanced (63). Currently, these protective effects on these cells are only reflected in the SMF, while the strong PMF accelerates cellular apoptosis. Prasad et al. reported that the moderate-intensity SMF (0.3 T) could induce the oligodendrocytes precursor cells to increase the intracellular Ca2+ influx by increasing the expression levels of L-type channel subunits—CaV1.2 and CaV1.3 (3). Numerous studies showed that the changes in ion channels were an important part of this process.
Studies on MNPs in various magnetic fields have shown that they have great potential for nerve regeneration. After the binding of MNPs to target cells, the magnetic force acting on the target cells becomes the most intuitive mechanism. Schwann cells, which are the commonly used target cells, are essential for the rapid saltatory propagation of action potential. Studies have focused on the effects of mechanical stimulation on Schwann cells (66). Recent studies showed that Schwann cells could create “stimuli” for themselves even in the absence of external mechanical stimulation (67, 68). They secrete basal lamina, an essential component of the SC's ECM, to enhance mechanical resistance. Mechanical stimulation might have a positive impact on SCs based on mechano-sensors and mechano-transducers (66). The stimulation can activate ECM, cell adhesion molecules, and some mechano-transduction pathways (69) and can enhance the expression levels of β1 integrins (70, 71). Moreover, low-level mechanical stimulation can promote the proliferation of SCs accompanied by demyelination and apoptosis (72–74). Xia et al. reported that miR-23b-3p from the extracellular vesicle of SCs might be a key factor of mechanical stimulation, promoting peripheral nerve regeneration (75). Meanwhile, force generation is downstream of many signaling cascades activated by neurotrophic factors, such as netrin-1 and NGF (76, 77). Raffa et al. quantified the correlations between the magnitude of mechanical force and nerve elongation (78, 79). This provided a theoretical basis for the quantitative design of MNPs to promote axonal regeneration.
Another possible mechanism is the magnetocaloric effects of MNPs in an external magnetic field, promoting nerve regeneration. This effect has been applied in bioengineering for a long time. Lin et al. completed and applied the localized heating of magnetic nanofibers to inactivate tumor cells (80). Although there is a lack of studies on the correlation of the magnetocaloric effect with nerve regeneration under a magnetic field, the correlation between temperature and neurite growth has been confirmed. He et al. reported that suitable temperature increased the serum levels of TGF-β and IL-10 and decreased the serum levels of TNF-α and IL-1β (81). This result is the same as the cytokine changes of nerve cells under the magnetic field. Numerous studies proved that the increase in temperature could positively affect the growth of neurites, and the temperature of 37–42°C might be the most suitable temperature for nerve growth (82).
The effects of the magnetic field on peripheral nerves are also reflected in growth factors and inflammatory factors. Zhang et al. reported that the low-intensity PMF could enhance the expression levels of myelin basic protein (MBP), myelin oligodendrocyte glycoprotein expressions (MOG), and transforming growth factor (TGF) (TGF-β1, TGF-βR1, and TGF-βR2) in the central nervous system (83). The TGF-β inhibitor could reduce the expression levels of NGF in a mouse model, which suggested that the stimulation of a magnetic field might promote the release of NGF (4). Mert et al. reported that the low-frequency PMF (1, 3, 5, 7Hz) not only increased compound action potential (CAP) amplitude and sciatic nerve conduction velocity (SNCV) but also reduced the expression levels of chemokines, such as CXCL1 and CCL3, which prevented the infiltration of immune cells and migration of neural progenitors (84). Moreover, PMF treatment could increase the expression levels of bFGF and decrease that of VEGF in the sciatic nerve. Interestingly, VEGF and bFGF indicated different results in diabetic mouse models, showing decreased expression levels (85). The mRNA expression levels of BDNF, GDNF, and VEGF were higher in the magnetic field-induced Schwann cells than those in the control group, while those of NT-3 were similar (86). There was no reasonable explanation for this phenomenon. It was speculated that this might be related to the differences in the growth directions of Schwann cells and neurites in the magnetic field.
During peripheral nerve regeneration, macrophages gradually convert from the pro-inflammatory (M1) phenotype to the anti-inflammatory (M2) phenotype (87–90). They are also associated with cellular proliferation and differentiation as well as the release of growth factors (91). Dai et al. reported that the combination of MNPs and alternating magnetic fields could promote macrophage to M2 polarization (92). In addition, they also suggested that this regulation might be directly related to the internalization of MNPs involved in activating the expression of interleukin 10 (IL-10), which might be amplified by the external magnetic field.
Brief exposure to SMF at 100 mT for 15 min led to a marked but transient potentiation of binding of a radiolabeled probe for activator protein-1 (AP1) in immature cultured rat hippocampal neurons. It caused a high expression level of growth-associated protein-43 and increased AP1 DNA binding through the expression of Fra-2, c-jun, and jun-D proteins. A study by Hirai provided a basis for finding the signaling pathway of the magnetic stimulatory effects on neurons (93). Liu et al. studied the mechanism of remyelination using in vitro and in vivo experiments on MNPs bound to SCs under SMFs. They suggested that Raf-MEK-ERK1/2, Rac1-MKK7-JNK-c-Jun, and TORC1-c-Jun pathways might be related to peripheral nerve regeneration under superparamagnetism. Notably, although there was a statistically significant increase for regeneration-related protein in the SPION + MF group as compared to the pure magnetic field group, it was not possible to determine whether this effect was due to superparamagnetism or mechanical stimulation (37).
Numerous high-quality studies are still required to establish a complete system for the mechanistic effects of magnetism on peripheral nerves, which should include all the aforementioned experimental phenomena.
4. Clinical applications
With the continuous theoretical studies on peripheral nerve regeneration using a magnetic field, relevant clinical applications are also being developed. Due to its muscle contractions and sensory afferents, repetitive peripheral magnetic stimulation (rPMS) is a non-invasive treatment for the nervous and musculoskeletal system (94) and has been widely used in many fields, such as reducing spasticity and improving the motor control of paretic limbs (95–97).
There is no conclusion about the underlying mechanism of rPMS. Researchers showed that the cortical plastic effects might be a key factor (98). The activation of the frontoparietal loops and an increase in corticomotor excitability are also important effects after magnetic stimulation (96). Liu et al. reported that the peripheral nerve electrical stimulation for 1 h could increase corticomotor excitability and hand dexterity improvement in patients with motor impairment after stroke (99). Other studies have shown similar results (100, 101).
A randomized controlled trial of 46 samples showed that the repetitive magnetic stimulation of the median nerve with 2,400 pulses (20 Hz over 10 min) could increase the peak motor evoked potential (MEP) amplitudes and RC slope in the contralateral hemisphere (98). Furthermore, the improvement in the Purdue Pegboard Test (PPT) score was significant after 24 h as compared to the baseline (*P = 0.003) and immediately after rPMS (*P = 0.012). Savulescu et al. reported that rPMS in association with physiokinesiotherapy (PKT) groups showed more improved results in the pain score and electromyography (EMG) analysis than in monotherapy groups (102).
rPMS has therapeutic effects on stroke-induced neurological and muscular sequelae. Jiang et al. reported that rPMS groups showed a better arm function and muscle strength for grip and elbow flexion and an extension of patients suffering from an early subacute stroke with severe upper extremity impairment than conventional physiotherapy groups (103). Zschorlich et al. reported that a 5-Hz rPMS could reduce the tendon reflex amplitude, which reduced muscle stiffness and increased mobility (104). Although rPMS involves the direct effect of magnetic field and peripheral nerve, there are few clinical studies on rPMS and peripheral nerve injury. The aforementioned research suggests that rPMS may play a role in promoting peripheral nerve regeneration. We need more high-quality studies to verify the efficacy and specific parameters of rPMS.
Magnetic fields are also applied in chemotherapy-induced peripheral neuropathy (CIPN), which is performed by axonal degeneration (vinca alkaloids) and demyelination (platinum compounds) (105, 106). Rick et al. reported that the 3-month magnetic field treatment could significantly improve NCV and subjectively perceived neurotoxicity (107). Moreover, there was no statistically significant difference in the pain detection end scores.
The International Commission on Non-Ionizing Radiation Protection (lower than 300 Hz for the general public and 400 Hz for occupational exposure) and the Institute of Electrical and Electronics Engineers International Committee on Electromagnetic Safety (lower than 750 Hz) have set limits on electromagnetic field thresholds for protection against stimulation and thermal effects (108–110). Moreover, the threshold of electromagnetic field strength is conservative due to a lack of correlation between internal and external field strengths and nerve activation (108). Hirata et al. used a multi-scale computation to study the threshold of peripheral magnetic stimulation based on a human anatomical model (108). They showed a margin factor of 4–6 and 10–24 times between internal and external protection limits of the international standards.
It is worth noting that the preliminary or explorative studies on the nature of rPMS have reported single cases, case series, or different stimulation protocols (111–115). Sollmann et al. made a checklist, including eight subject-related items, such as age, gender, handedness, or footedness; 16 methodological items, such as coil location, coil type, pulse duration, and shape; and 11 stimulation protocol items, such as stimulation frequency, stimulation intensity, and duty cycle, to guide the research direction of rPMS and contribute to new interventional or exploratory rPMS studies (116).
5. Conclusion and future prospects
This review discusses magnetic field properties and intensities, MNP-encapsulating various cytokines based on superparamagnetism, magnetically functionalized nanofibers, relevant mechanisms, and some clinical applications. This review attempted to completely and systematically analyze the latest studies on these topics.
At the same time, there are numerous problems in this field, which should be solved by researchers in their future studies. First, a suitable magnetic field mode and methods to be used are needed to be formulated. Gleich et al. reported that the cornered FO8 coil was more effective, which could reduce the stimulation voltage, current, and energy of the stimulation device (117). Abe et al. also used a newly developed stimulator to reduce the pain and discomfort of magnetic stimulation (118). Heiland et al. reported that the magnetization transfer ratio in the peripheral nerve tissue altered with age (119). This indicated that the mode setting of peripheral nerve magnetic stimulation therapy should be adjusted based on the age of patients.
In addition, the relevant mechanism requires further exploration. The molecular mechanisms of these problems are still unknown. Using experiments based on MNPs-Schwann cells under SMFs, Liu et al. showed that Raf-MEK-ERK1/2, Rac1-MKK7-JNK-c-Jun, and TORC1-c-Jun pathways might be related to peripheral nerve regeneration; however, there is no evidence determining whether this effect was due to superparamagnetism or mechanical stimulation. Moreover, the direction of cell growth was correlated with the cell type under different magnetic fields. According to previous studies, Schwann cells tended to grow parallel to the direction of the magnetic field, while neurites grew perpendicular to the magnetic field. This difference might limit the potential of axon and myelin sheath growth under a magnetic field. Future studies should focus on finding a more reasonable magnetic field placement mode, strength, and time to obtain the best effects with the smallest damage.
The effects of magnetism on peripheral nerves have always been a research hotspot. A suitable application of magnetic field or magnetic biomaterials can shorten the regeneration time of peripheral nerves, enhance the orientation of nerves, and promote the release of growth factors. In future, more high-quality studies are warranted, which could further clarify the mechanism of magnetic action and formulate guidelines for magnetic therapy.
Author contributions
All authors were involved in the conception and choice of approach for the expert consensus and also contributed findings from their knowledge and experience to the expert consensus, drafted the manuscript, critically revised the manuscript for important intellectual content, and approved the final version of the manuscript.
Funding
This study was supported by the Research Project of the Education Department of Jilin Province (No. JJKH20211187KJ) and the Industrial Technology Research and Development of the Development and Reform Commission of Jilin Province (No. 2021C042-4).
Acknowledgments
The authors would like to acknowledge Xiang Li (College of Chemistry, Jilin University, Changchun, China) for providing support related to materials science, and Shusen Cui (China-Japan Union Hospital of Jilin University, Changchun, China) for medical writing assistance with the preparation of this article.
Conflict of interest
The authors declare that the research was conducted in the absence of any commercial or financial relationships that could be construed as a potential conflict of interest.
Publisher's note
All claims expressed in this article are solely those of the authors and do not necessarily represent those of their affiliated organizations, or those of the publisher, the editors and the reviewers. Any product that may be evaluated in this article, or claim that may be made by its manufacturer, is not guaranteed or endorsed by the publisher.
References
1. Li R, Liu Z, Pan Y, Chen L, Zhang Z, Lu L. Peripheral nerve injuries treatment: a systematic review. Cell Biochem Biophys. (2014) 68:449–54. doi: 10.1007/s12013-013-9742-1
2. Hu Y, Li D, Wei H, Zhou S, Chen W, Yan X, et al. Neurite extension and orientation of spiral ganglion neurons can be directed by superparamagnetic iron oxide nanoparticles in a magnetic field. Int J Nanomedicine. (2021) 16:4515–26. doi: 10.2147/IJN.S313673
3. Prasad A, Teh DBL, Blasiak A, Chai C, Wu Y, Gharibani PM, et al. Static magnetic field stimulation enhances oligodendrocyte differentiation and secretion of neurotrophic factors. Sci Rep. (2017) 7:6743. doi: 10.1038/s41598-017-06331-8
4. Yokozeki Y, Uchida K, Kawakubo A, Nakawaki M, Okubo T, Miyagi M, et al. TGF-β regulates nerve growth factor expression in a mouse intervertebral disc injury model. BMC Musculoskeletal Disord. (2021) 22:634. doi: 10.1186/s12891-021-04509-w
5. Yang M, Jian L, Fan W, Chen X, Zou H, Huang Y, et al. Axon regeneration after optic nerve injury in rats can be improved via PirB knockdown in the retina. Cell Biosci. (2021) 11:158. doi: 10.1186/s13578-021-00670-w
6. Murayama M. Orientation of sickled erythrocytes in a magnetic field. Nature. (1965) 206:420–2. doi: 10.1038/206420a0
7. Emura R, Takeuchi T, Nakaoka Y, Higashi T. Analysis of anisotropic diamagnetic susceptibility of a bull sperm. Bioelectromagnetics. (2003) 24:347–55. doi: 10.1002/bem.10109
8. Guido S, Tranquillo RT. A methodology for the systematic and quantitative study of cell contact guidance in oriented collagen gels. Correlation of fibroblast orientation and gel birefringence. J Cell Sci. (1993) 105:317–31. doi: 10.1242/jcs.105.2.317
9. Kotani, Hiroko, Iwasaka, Masakazu, Ueno, Shoogo, et al. Magnetic orientation of collagen and bone mixture. J Appl Physics. (2000) 87:6191. doi: 10.1063/1.372652
10. Hirose H, Nakahara T, Miyakoshi J. Orientation of human glioblastoma cells embedded in type I collagen, caused by exposure to a 10 T static magnetic field. Neurosci Lett. (2003) 338:88–90. doi: 10.1016/S0304-3940(02)01363-0
11. Eguchi Y, Ogiue-Ikeda M, Ueno S. Control of orientation of rat Schwann cells using an 8-T static magnetic field. Neurosci Lett. (2003) 351:130–2. doi: 10.1016/S0304-3940(03)00719-5
12. Kim S, Im WS, Kang L, Lee ST, Chu K, Kim BI. The application of magnets directs the orientation of neurite outgrowth in cultured human neuronal cells. J Neurosci Methods. (2008) 174:91–6. doi: 10.1016/j.jneumeth.2008.07.005
13. Torbet J, Ronzière MC. Magnetic alignment of collagen during self-assembly. Biochem J. (1984) 219:1057–9. doi: 10.1042/bj2191057
14. Schmidt CE, Leach JB. Neural tissue engineering: strategies for repair and regeneration. Annu Rev Biomed Eng. (2003) 5:293–347. doi: 10.1146/annurev.bioeng.5.011303.120731
15. Stoll G, Griffin JW, Li CY, Trapp BD. Wallerian degeneration in the peripheral nervous system: participation of both Schwann cells and macrophages in myelin degradation. J Neurocytol. (1989) 18:671–83. doi: 10.1007/BF01187086
16. Funnell JL, Balouch B, Gilbert RJ. Magnetic composite biomaterials for neural regeneration. Front Bioeng Biotechnol. (2019) 7:179. doi: 10.3389/fbioe.2019.00179
17. D'Agata F, Ruffinatti FA, Boschi S, Stura I, Rainero I, Abollino O, et al. Magnetic nanoparticles in the central nervous system: targeting principles, applications and safety issues. Molecules. (2017) 23:9. doi: 10.3390/molecules23010009
18. Ito H, Bassett CAL. Effect of weak, pulsing electromagnetic fields on neural regeneration in the rat. Clin Orthop Relat Res. (1983) 181:283–90. doi: 10.1097/00003086-198312000-00044
19. Kanje M, Rusovan A, Sisken B, Lundborg G. Pretreatment of rats with pulsed electromagnetic fields enhances regeneration of the sciatic nerve. Bioelectromagnetics. (1993) 14:353–9. doi: 10.1002/bem.2250140407
20. Pedro J, Pérez-Caballer A, Dominguez J, Collía F, Blanco J, Salvado M. Pulsed electromagnetic fields induce peripheral nerve regeneration and endplate enzymatic changes. Bioelectromagnetics. (2005) 26:20–7. doi: 10.1002/bem.20049
21. Raylman RR, Clavo AC, Wahl RL. Exposure to strong static magnetic field slows the growth of human cancer cells in vitro. Bioelectromagnetics. (1996) 17:358–63. doi: 10.1002/(SICI)1521-186X(1996)17:5 < 358::AID-BEM2>3.0.CO;2-2
22. Buemi M, Marino D, Pasquale GD, Floccari F, Senatore M, Aloisi C, et al. Cell proliferation/cell death balance in renal cell cultures after exposure to a static magnetic field. Nephron. (2001) 87:269–73. doi: 10.1159/000045925
23. Schiffer IB, Schreiber WG, Graf R, Schreiber EM, Jung D, Rose DM, et al. No influence of magnetic fields on cell cycle progression using conditions relevant for patients during MRI. Bioelectromagnetics. (2003) 24:241–50. doi: 10.1002/bem.10097
24. Mann A, Steinecker-Frohnwieser B, Naghilou A, Millesi F, Supper P, Semmler L, et al. Nuclear magnetic resonance treatment accelerates the regeneration of dorsal root ganglion neurons. Front Cell Neurosci. (2022) 16:859545. doi: 10.3389/fncel.2022.859545
25. Macias MY, Battocletti JH, Sutton CH, Pintar FA, Maiman DJ. Directed and enhanced neurite growth with pulsed magnetic field stimulation. Bioelectromagnetics. (2015) 21:272–86. doi: 10.1002/(SICI)1521-186X(200005)21:4<272::AID-BEM4>3.3.CO;2-X
26. Torbet J, Freyssinet JM, Hudry-Clergeon G. Oriented fibrin gels formed by polymerization in strong magnetic fields. Nature. (1981) 289:91–3. doi: 10.1038/289091a0
27. Heid S, Unterweger H, Tietze R, Friedrich RP, Weigel B, Cicha I, et al. Synthesis and characterization of tissue plasminogen activator-functionalized superparamagnetic iron oxide nanoparticles for targeted fibrin clot dissolution. Int J Mol Sci. (2017) 18:1837. doi: 10.3390/ijms18091837
28. Higashi T, Yamagishi A, Takeuchi T, Kawaguchi N, Sagawa S, Onishi S, et al. Orientation of erythrocytes in a strong static magnetic field. Blood. (1993) 82:1328–34. doi: 10.1182/blood.V82.4.1328.1328
29. Kotani H, Kawaguchi H, Shimoaka T, Iwasaka M, Ueno S, Ozawa H, et al. Strong static magnetic field stimulates bone formation to a definite orientation in vitro and in vivo. J Bone Min Res. (2002) 17:1814–21. doi: 10.1359/jbmr.2002.17.10.1814
30. Ikehata M, Koana T, Suzuki Y, Shimizu H, Nakagawa M. Mutagenicity and co-mutagenicity of static magnetic fields detected by bacterial mutation assay. Mutat Res. (1999) 427:147. doi: 10.1016/S0027-5107(99)00087-1
31. ZmyLony M, Palus J, Jajte J, Dziubaltowska E, Rajkowska E. DNA damage in rat lymphocytes treated in vitro with iron cations and exposed to 7 mT magnetic fields (static or 50 Hz). Mutat Res. (2000) 453:89–96. doi: 10.1016/S0027-5107(00)00094-4
32. Liu L, Liu Z, Huang L, Sun Z, Ma T, Zhu S, et al. Pulsed magnetic field promotes proliferation and neurotrophic genes expression in Schwann cells in vitro. Int J Clin Exp Pathol. (2015) 8:2343–53.
33. Giannaccini M, Calatayud MP, Poggetti A, Corbianco S, Novelli M, Paoli M, et al. Magnetic nanoparticles for efficient delivery of growth factors: stimulation of peripheral nerve regeneration. Adv Healthc Mater. (2017) 6. doi: 10.1002/adhm.201601429
34. Kim JA, Lee N, Kim BH, Rhee WJ, Yoon S, Hyeon T, et al. Enhancement of neurite outgrowth in PC12 cells by iron oxide nanoparticles. Biomaterials. (2011) 32:2871–7. doi: 10.1016/j.biomaterials.2011.01.019
35. Pop NL, Nan A, Urda-Cimpean AE, Florea A, Toma VA, Moldovan R, et al. Chitosan functionalized magnetic nanoparticles to provide neural regeneration and recovery after experimental model induced peripheral nerve injury. Biomolecules. (2021) 11:676. doi: 10.3390/biom11050676
36. Yuan M, Wang Y, Qin Y-X. Promoting neuroregeneration by applying dynamic magnetic fields to a novel nanomedicine: superparamagnetic iron oxide (SPIO)-gold nanoparticles bounded with nerve growth factor (NGF). Nanomedicine. (2018) 14:1337–47. doi: 10.1016/j.nano.2018.03.004
37. Liu T, Wang Y, Lu L, Liu Y. SPIONs mediated magnetic actuation promotes nerve regeneration by inducing and maintaining repair-supportive phenotypes in Schwann cells. J Nanobiotechnology. (2022) 20:159. doi: 10.1186/s12951-022-01337-5
38. Wang Y, Du S, Liu T, Ren J, Zhang J, Xu H, et al. Schwann cell migration through magnetic actuation mediated by fluorescent-magnetic bifunctional FeO·rhodamine 6G@polydopamine superparticles. ACS Chem Neurosci. (2020) 11:1359–70. doi: 10.1021/acschemneuro.0c00116
39. Riggio C, Calatayud MP, Giannaccini M, Sanz B, Torres TE, Fernández-Pacheco R, et al. The orientation of the neuronal growth process can be directed via magnetic nanoparticles under an applied magnetic field. Nanomedicine. (2014) 10:1549–58. doi: 10.1016/j.nano.2013.12.008
40. Gao J, Xia B, Li S, Huang L, Ma T, Shi X, et al. Magnetic field promotes migration of schwann cells with chondroitinase ABC (ChABC)-loaded superparamagnetic nanoparticles across astrocyte boundary in vitro. Int J Nanomedicine. (2020) 15:315–32. doi: 10.2147/IJN.S227328
41. Frenot A, Chronakis IS. Polymer nanofibers assembled by electrospinning. Curr Opin Colloid Interface Sci. (2003) 8:64–75. doi: 10.1016/S1359-0294(03)00004-9
42. Kim JS. Improved mechanical properties of composites using ultrafine electrospun fibers. Univ Akron. (1997) 20:124–31. doi: 10.1002/pc.10340
43. Lee H-J, Lee SJ, Uthaman S, Thomas RG, Hyun H, Jeong YY, et al. Biomedical applications of magnetically functionalized organic/inorganic hybrid nanofibers. Int J Mol Sci. (2015) 16:13661–77. doi: 10.3390/ijms160613661
44. Awada H, Al Samad A, Laurencin D, Gilbert R, Dumail X, El Jundi A, et al. Controlled anchoring of iron oxide nanoparticles on polymeric nanofibers: easy access to Core@Shell organic-inorganic nanocomposites for magneto-scaffolds. ACS Appl Mater Interfaces. (2019) 11:9519–29. doi: 10.1021/acsami.8b19099
45. Mortimer CJ, Wright CJ. The fabrication of iron oxide nanoparticle-nanofiber composites by electrospinning and their applications in tissue engineering. Biotechnol J. (2017) 12. doi: 10.1002/biot.20160069
46. Gao D, Jiang J-J, Gu S-H, Lu J-Z, Xu L. Morphological detection and functional assessment of regenerated nerve after neural prosthesis with a PGLA nerve conduit. Sci Rep. (2017) 7:46403. doi: 10.1038/srep46403
47. Singh RK, Patel KD, Lee JH, Lee E-J, Kim J-H, Kim T-H, et al. Potential of magnetic nanofiber scaffolds with mechanical and biological properties applicable for bone regeneration. PLoS ONE. (2014) 9:e91584. doi: 10.1371/journal.pone.0091584
48. Wei Y, Zhang X, Song Y, Han B, Hu X, Wang X, et al. Magnetic biodegradable Fe3O4/CS/PVA nanofibrous membranes for bone regeneration. Biomed Mater. (2011) 6:055008. doi: 10.1088/1748-6041/6/5/055008
49. Zeng XB, Hu H, Xie LQ, Lan F, Jiang W, Wu Y, et al. Magnetic responsive hydroxyapatite composite scaffolds construction for bone defect reparation. Int J Nanomedicine. (2012) 7:3365–78. doi: 10.2147/IJN.S32264
50. Shan D, Shi Y, Duan S, Wei Y, Cai Q, Yang X. Electrospun magnetic poly(L-lactide) (PLLA) nanofibers by incorporating PLLA-stabilized Fe3O4 nanoparticles. Mater Sci Eng C Mater Biol Appl. (2013) 33:3498–505. doi: 10.1016/j.msec.2013.04.040
51. Annabi N, Tamayol A, Uquillas JA, Akbari M, Bertassoni LE, Cha C, et al. 25th anniversary article: rational design and applications of hydrogels in regenerative medicine. Adv Mater. (2014) 26:85–123. doi: 10.1002/adma.201303233
52. Johnson CDL, Ganguly D, Zuidema JM, Cardinal TJ, Ziemba AM, Kearns KR, et al. Injectable, magnetically orienting electrospun fiber conduits for neuron Guidance. ACS Appl Mater Interfaces. (2019) 11:356–72. doi: 10.1021/acsami.8b18344
53. Funnell JL, Ziemba AM, Nowak JF, Awada H, Prokopiou N, Samuel J, et al. Assessing the combination of magnetic field stimulation, iron oxide nanoparticles, and aligned electrospun fibers for promoting neurite outgrowth from dorsal root ganglia in vitro. Acta Biomater. (2021) 131:302–13. doi: 10.1016/j.actbio.2021.06.049
54. Lacko CS, Singh I, Wall MA, Garcia AR, Porvasnik SL, Rinaldi C, et al. Magnetic particle templating of hydrogels: engineering naturally derived hydrogel scaffolds with 3D aligned microarchitecture for nerve repair. J Neural Eng. (2020) 17:016057. doi: 10.1088/1741-2552/ab4a22
55. Kasper M, Deister C, Beck F, Schmidt CE. Bench-to-bedside lessons learned: commercialization of an acellular nerve graft. Adv Healthc Mater. (2020) 9:e2000174. doi: 10.1002/adhm.202000174
56. Kuliasha CA, Judy JW. In vitro reactive-accelerated-aging (RAA) assessment of tissue-engineered electronic nerve interfaces (TEENI). Annu Int Conf IEEE Eng Med Biol Soc. (2018) 2018:5061–4. doi: 10.1109/EMBC.2018.8513458
57. Kasper M, Ellenbogen B, Hardy R, Cydis M, Mojica-Santiago J, Afridi A, et al. Development of a magnetically aligned regenerative tissue-engineered electronic nerve interface for peripheral nerve applications. Biomaterials. (2021) 279:121212. doi: 10.1016/j.biomaterials.2021.121212
58. Wang B, Zheng H, Chang M-W, Ahmad Z, Li J-S. Hollow polycaprolactone composite fibers for controlled magnetic responsive antifungal drug release. Colloids Surf B Biointerfaces. (2016) 145:757–67. doi: 10.1016/j.colsurfb.2016.05.092
59. Rosen AD. Mechanism of action of moderate-intensity static magnetic fields on biological systems. Cell Biochem Biophys. (2003) 39:163–73. doi: 10.1385/CBB:39:2:163
60. Mathie A, Kennard LE, Veale EL. Neuronal ion channels and their sensitivity to extremely low frequency weak electric field effects. Radiat Prot Dosimetry. (2003) 106:311–6. doi: 10.1093/oxfordjournals.rpd.a006365
61. Marchionni I, Paffi A, Pellegrino M, Liberti M, Apollonio F, Abeti R, et al. Comparison between low-level 50 Hz and 900 MHz electromagnetic stimulation on single channel ionic currents and on firing frequency in dorsal root ganglion isolated neurons. Biochim Biophys Acta. (2006) 1758:597–605. doi: 10.1016/j.bbamem.2006.03.014
62. Saunders RD, Jefferys JGR. A neurobiological basis for ELF guidelines. Health Phys. (2007) 92:596–603. doi: 10.1097/01.HP.0000257856.83294.3e
63. Ben Yakir-Blumkin M, Loboda Y, Schächter L, Finberg JPM. Neuroprotective effect of weak static magnetic fields in primary neuronal cultures. Neuroscience. (2014) 278:313–26. doi: 10.1016/j.neuroscience.2014.08.029
64. Aldinucci C, Garcia JB, Palmi M, Sgaragli G, Benocci A, Meini A, et al. The effect of exposure to high flux density static and pulsed magnetic fields on lymphocyte function. Bioelectromagnetics. (2003) 24:373–9. doi: 10.1002/bem.10111
65. Balassa T, Varró P, Elek S, Drozdovszky O, Szemerszky R, Világi I, et al. Changes in synaptic efficacy in rat brain slices following extremely low-frequency magnetic field exposure at embryonic and early postnatal age. Int J Dev Neurosci. (2013) 31:724–30. doi: 10.1016/j.ijdevneu.2013.08.004
66. Belin S, Zuloaga KL, Poitelon Y. Influence of mechanical stimuli on Schwann cell biology. Front Cell Neurosci. (2017) 11:347. doi: 10.3389/fncel.2017.00347
67. Feltri ML, Poitelon Y, Previtali SC. How Schwann cells sort axons: new concepts. Neuroscientist. (2016) 22:252–65. doi: 10.1177/1073858415572361
68. Monk KR, Feltri ML, Taveggia C. New insights on Schwann cell development. Glia. (2015) 63:1376–93. doi: 10.1002/glia.22852
69. Poitelon Y, Bogni S, Matafora V, Della-Flora Nunes G, Hurley E, Ghidinelli M, et al. Spatial mapping of juxtacrine axo-glial interactions identifies novel molecules in peripheral myelination. Nat Commun. (2015) 6:8303. doi: 10.1038/ncomms9303
70. Friedland JC, Lee MH, Boettiger D. Mechanically activated integrin switch controls alpha5beta1 function. Science. (2009) 323:642–4. doi: 10.1126/science.1168441
71. Kong F, García AJ, Mould AP, Humphries MJ, Zhu C. Demonstration of catch bonds between an integrin and its ligand. J Cell Biol. (2009) 185:1275–84. doi: 10.1083/jcb.200810002
72. Gupta R, Steward O. Chronic nerve compression induces concurrent apoptosis and proliferation of Schwann cells. J Comp Neurol. (2003) 461:174–86. doi: 10.1002/cne.10692
73. Gupta R, Truong L, Bear D, Chafik D, Modafferi E, Hung CT. Shear stress alters the expression of myelin-associated glycoprotein (MAG) and myelin basic protein (MBP) in Schwann cells. J Orthopaed Res. (2005) 23:1232–9. doi: 10.1016/j.orthres.2004.12.010
74. Salzer JL, Bunge RP. Studies of Schwann cell proliferation. I An analysis in tissue culture of proliferation during development, Wallerian degeneration, and direct injury. J Cell Biol. (1980) 84:739–52. doi: 10.1083/jcb.84.3.739
75. Xia B, Gao J, Li S, Huang L, Zhu L, Ma T, et al. Mechanical stimulation of Schwann cells promote peripheral nerve regeneration via extracellular vesicle-mediated transfer of microRNA 23b-3p. Theranostics. (2020) 10:8974–95. doi: 10.7150/thno.44912
76. Toriyama M, Kozawa S, Sakumura Y, Inagaki N. Conversion of a signal into forces for axon outgrowth through Pak1-mediated shootin1 phosphorylation. Curr Biol. (2013) 23:529–34. doi: 10.1016/j.cub.2013.02.017
77. Turney SG, Ahmed M, Chandrasekar I, Wysolmerski RB, Goeckeler ZM, Rioux RM, et al. Nerve growth factor stimulates axon outgrowth through negative regulation of growth cone actomyosin restraint of microtubule advance. Mol Biol Cell. (2016) 27:500–17. doi: 10.1091/mbc.e15-09-0636
78. Raffa V, Falcone F, De Vincentiis S, Falconieri A, Calatayud MP, Goya GF, et al. Piconewton mechanical forces promote neurite growth. Biophys J. (2018) 115:2026–33. doi: 10.1016/j.bpj.2018.10.009
79. De Vincentiis S, Falconieri A, Mainardi M, Cappello V, Scribano V, Bizzarri R, et al. Extremely low forces induce extreme axon growth. J Neurosci. (2020) 40:4997–5007. doi: 10.1523/JNEUROSCI.3075-19.2020
80. Lin T-C, Lin F-H, Lin J-C. In vitro feasibility study of the use of a magnetic electrospun chitosan nanofiber composite for hyperthermia treatment of tumor cells. Acta Biomater. (2012) 8:2704–11. doi: 10.1016/j.actbio.2012.03.045
81. He L-P, Liu P-Z, Wen Y-M, Wu J. Effect of temperature maintenance by forced-air warming blankets of different temperatures on changes in inflammatory factors in children undergoing congenital hip dislocation surgery. Chin Med J. (2020) 133:1768–73. doi: 10.1097/CM9.0000000000000846
82. Cancalon P. Influence of temperature on various mechanisms associated with neuronal growth and nerve regeneration. Prog Neurobiol. (1985) 25:27–92. doi: 10.1016/0301-0082(85)90022-X
83. Wang Z, Baharani A, Wei Z, Truong D, Bi X, Wang F, et al. Low field magnetic stimulation promotes myelin repair and cognitive recovery in chronic cuprizone mouse model. Clin Exp Pharmacol Physiol. (2021) 48:1090–102. doi: 10.1111/1440-1681.13490
84. Mert T, Metin TO, Sahin E, Yaman S, Sahin M. Neuroprotective and anti-neuropathic actions of pulsed magnetic fields with low frequencies in rats with chronic peripheral neuropathic pain. Brain Res Bull. (2021) 177:273–81. doi: 10.1016/j.brainresbull.2021.10.012
85. Mert T, Sahin E, Yaman S, Sahin M. Pulsed magnetic field treatment ameliorates the progression of peripheral neuropathy by modulating the neuronal oxidative stress, apoptosis and angiogenesis in a rat model of experimental diabetes. Arch Physiol Biochem. (2020) 128:1658–65. doi: 10.1080/13813455.2020.1788098
86. Liu L, Liu Z, Huang L, Sun Z, Luo Z. Pulsed magnetic field promotes proliferation and neurotrophic genes expression in Schwann cells in vitro. Int J Clin Exp Pathol. (2015) 8:2343.
87. Ferrante CJ, Leibovich SJ. Regulation of macrophage polarization and wound healing. Adv Wound Care. (2012) 1:10–6. doi: 10.1089/wound.2011.0307
88. Novak ML, Koh TJ. Phenotypic transitions of macrophages orchestrate tissue repair. Am J Pathol. (2013) 183:1352–63. doi: 10.1016/j.ajpath.2013.06.034
89. Chen P, Piao X, Bonaldo P. Role of macrophages in Wallerian degeneration and axonal regeneration after peripheral nerve injury. Acta Neuropathol. (2015) 130:605–18. doi: 10.1007/s00401-015-1482-4
90. Gensel JC, Zhang B. Macrophage activation and its role in repair and pathology after spinal cord injury. Brain Res. (2015) 1619:1–11. doi: 10.1016/j.brainres.2014.12.045
91. Mokarram N, Merchant A, Mukhatyar V, Patel G, Bellamkonda RV. Effect of modulating macrophage phenotype on peripheral nerve repair. Biomaterials. (2012) 33:8793–801. doi: 10.1016/j.biomaterials.2012.08.050
92. Guo W, Wu X, Wei W, Wang Y, Dai H. Mesoporous hollow FeO nanoparticles regulate the behavior of neuro-associated cells through induction of macrophage polarization in an alternating magnetic field. J Mater Chem B. (2022) 10:5633–43. doi: 10.1039/D2TB00527A
93. Hirai T, Nakamichi N, Yoneda Y. Activator protein-1 complex expressed by magnetism in cultured rat hippocampal neurons. Biochem Biophys Res Commun. (2002) 292:200–7. doi: 10.1006/bbrc.2002.6618
94. Beaulieu LD, Schneider C. Repetitive peripheral magnetic stimulation to reduce pain or improve sensorimotor impairments: a literature review on parameters of application and afferents recruitment. Neurophysiol Clin. (2015) 45:223–37. doi: 10.1016/j.neucli.2015.08.002
95. Struppler A, Havel P, Müller-Barna P. Facilitation of skilled finger movements by repetitive peripheral magnetic stimulation (RPMS) - a new approach in central paresis. NeuroRehabilitation. (2003) 18:69–82. doi: 10.3233/NRE-2003-18108
96. Struppler A, Binkofski F, Angerer B, Bernhardt M, Spiegel S, Drzezga A, et al. A fronto-parietal network is mediating improvement of motor function related to repetitive peripheral magnetic stimulation: a PET-H2O15 study. Neuroimage. (2007) 36 Suppl 2:T174–T86. doi: 10.1016/j.neuroimage.2007.03.033
97. Beaulieu L-D, Massé-Alarie H, Brouwer B, Schneider C. Noninvasive neurostimulation in chronic stroke: a double-blind randomized sham-controlled testing of clinical and corticomotor effects. Top Stroke Rehabil. (2015) 22:8–17. doi: 10.1179/1074935714Z.0000000032
98. Jia Y, Liu X, Wei J, Li D, Wang C, Wang X, et al. Modulation of the corticomotor excitability by repetitive peripheral magnetic stimulation on the median nerve in healthy subjects. Front Neural Circuits. (2021) 15:616084. doi: 10.3389/fncir.2021.616084
99. Liu H, Au-Yeung SSY. Corticomotor excitability effects of peripheral nerve electrical stimulation to the paretic arm in stroke. Am J Phys Med Rehabil. (2017) 96:687–93. doi: 10.1097/PHM.0000000000000748
100. Guarda SNFD, Conforto AB. Effects of somatosensory stimulation on corticomotor excitability in patients with unilateral cerebellar infarcts and healthy subjects - preliminary results. Cerebell Ataxias. (2014) 1:16. doi: 10.1186/s40673-014-0016-5
101. Chen C-C, Chuang Y-F, Yang H-C, Hsu M-J, Huang Y-Z, Chang Y-J. Neuromuscular electrical stimulation of the median nerve facilitates low motor cortex excitability in patients with spinocerebellar ataxia. J Electromyo Kinesiol. (2015) 25:143–50. doi: 10.1016/j.jelekin.2014.10.009
102. Savulescu SE, Berteanu M, Filipescu I, Beiu C, Mihai M-M, Popa LG, et al. Repetitive peripheral magnetic stimulation (rPMS) in subjects with lumbar radiculopathy: an electromyography-guided prospective, randomized study. In Vivo. (2021) 35:623–7. doi: 10.21873/invivo.12300
103. Jiang Y-F, Zhang D, Zhang J, Hai H, Zhao Y-Y, Ma Y-W, et al. Randomized controlled trial of repetitive peripheral magnetic stimulation applied in early subacute stroke: effects on severe upper-limb impairment. Clin Rehabil. (2022) 36:693–702. doi: 10.1177/02692155211072189
104. Zschorlich VR, Hillebrecht M, Tanjour T, Qi F, Behrendt F, Kirschstein T, et al. Repetitive peripheral magnetic nerve stimulation (rPMS) as adjuvant therapy reduces skeletal muscle reflex activity. Front Neurol. (2019) 10:930. doi: 10.3389/fneur.2019.00930
105. Sahenk Z, Brady ST, Mendell JR. Studies on the pathogenesis of vincristine-induced neuropathy. Muscle Nerve. (1987) 10:80–4. doi: 10.1002/mus.880100115
106. Shemesh OA, Spira ME. Paclitaxel induces axonal microtubules polar reconfiguration and impaired organelle transport: implications for the pathogenesis of paclitaxel-induced polyneuropathy. Acta Neuropathol. (2010) 119:235–48. doi: 10.1007/s00401-009-0586-0
107. Rick O, Hehn UV, Mikus E, Dertinger H, Geiger G. Magnetic field therapy in patients with cytostatics-induced polyneuropathy: a prospective randomized placebo-controlled phase-III study. Bioelectromagnetics. (2018) 38:85–94. doi: 10.1002/bem.22005
108. Suzuki Y, Gomez-Tames J, Diao Y, Hirata A. Evaluation of peripheral electrostimulation thresholds in human model for uniform magnetic field exposure. Int J Environ Res Public Health. (2021) 19:390. doi: 10.3390/ijerph19010390
109. Guidelines for limiting exposure to time-varying electric magnetic and electromagnetic fields (up to 300 GHz). International commission on non-ionizing radiation protection. Health Phys. (1998) 74:494–522.
110. Ibey BL, Roth CC, Ledwig PB, Payne JA, Amato AL, Dalzell DR, et al. Cellular effects of acute exposure to high peak power microwave systems: morphology and toxicology. Bioelectromagnetics. (2016) 37:141–51. doi: 10.1002/bem.21962
111. Flamand VH, Schneider C. Noninvasive and painless magnetic stimulation of nerves improved brain motor function and mobility in a cerebral palsy case. Arch Phys Med Rehabil. (2014) 95:1984–90. doi: 10.1016/j.apmr.2014.05.014
112. Kinoshita S, Ikeda K, Hama M, Suzuki S, Abo M. Repetitive peripheral magnetic stimulation combined with intensive physical therapy for gait disturbance after hemorrhagic stroke: an open-label case series. Int J Rehabil Res. (2020) 43:235–9. doi: 10.1097/MRR.0000000000000416
113. Leung A, Fallah A, Shukla S. Transcutaneous magnetic stimulation (TMS) in alleviating post-traumatic peripheral neuropathic pain States: a case series. Pain Med. (2014) 15:1196–9. doi: 10.1111/pme.12426
114. Momosaki R, Abo M, Watanabe S, Kakuda W, Yamada N, Kinoshita S. Repetitive peripheral magnetic stimulation with intensive swallowing rehabilitation for poststroke dysphagia: an open-label case series. Neuromodulation. (2015) 18:630–4. doi: 10.1111/ner.12308
115. Elena SS, Lavinia G, Florina P, Luminita D, Mihai AB. Peripheral repetitive magnetic stimulation: a novel approach for hand rehabilitation in carpal tunnel syndrome - a pilot study. Int J Soc Sci Human. (2017) 6:604–7. doi: 10.7763/IJSSH.2016.V6.718
116. Schneider C, Zangrandi A, Sollmann N, Bonfert MV, Beaulieu L-D. Checklist on the quality of the repetitive peripheral magnetic stimulation (rPMS) methods in research: an international delphi study. Front Neurol. (2022) 13:852848. doi: 10.3389/fneur.2022.852848
117. Braun P, Rapp J, Hemmert W, Gleich B. Coil efficiency for inductive peripheral nerve stimulation. IEEE Trans Neural Syst Rehabil Eng. (2022) 30:2137–45. doi: 10.1109/TNSRE.2022.3192761
118. Abe G, Oyama H, Liao Z, Honda K, Yashima K, Asao A, et al. Difference in pain and discomfort of comparable wrist movements induced by magnetic or electrical stimulation for peripheral nerves in the dorsal forearm. Med Devices. (2020) 13:439–47. doi: 10.2147/MDER.S271258
Keywords: magnetic nanoparticles, magnetical nanofibers, peripheral nerve regeneration, magnetic field, repetitive peripheral magnetic stimulation
Citation: Fan Z, Wen X, Ding X, Wang Q, Wang S and Yu W (2023) Advances in biotechnology and clinical therapy in the field of peripheral nerve regeneration based on magnetism. Front. Neurol. 14:1079757. doi: 10.3389/fneur.2023.1079757
Received: 25 October 2022; Accepted: 07 February 2023;
Published: 10 March 2023.
Edited by:
Lei Xu, Fudan University, ChinaReviewed by:
Giovanna Squintani, Azienda Ospedaliera Universitaria Integrata Verona, ItalyBo Cui, Xuanwu Hospital, Capital Medical University, China
Copyright © 2023 Fan, Wen, Ding, Wang, Wang and Yu. This is an open-access article distributed under the terms of the Creative Commons Attribution License (CC BY). The use, distribution or reproduction in other forums is permitted, provided the original author(s) and the copyright owner(s) are credited and that the original publication in this journal is cited, in accordance with accepted academic practice. No use, distribution or reproduction is permitted which does not comply with these terms.
*Correspondence: Wei Yu, yuweiwei@jlu.edu.cn