Epidermal Growth Factor Pathway in the Age-Related Decline of Oligodendrocyte Regeneration
- 1Department of Neuroscience, Institute of Human Anatomy, University of Padua, Padua, Italy
- 2Department of Neurology, Medical Faculty, Heinrich-Heine-University, Düsseldorf, Germany
- 3School of Pharmacy and Biomedical Science, University of Portsmouth, Portsmouth, United Kingdom
Oligodendrocytes (OLs) are specialized glial cells that myelinate CNS axons. OLs are generated throughout life from oligodendrocyte progenitor cells (OPCs) via a series of tightly controlled differentiation steps. Life-long myelination is essential for learning and to replace myelin lost in age-related pathologies such as Alzheimer’s disease (AD) as well as white matter pathologies such as multiple sclerosis (MS). Notably, there is considerable myelin loss in the aging brain, which is accelerated in AD and underpins the failure of remyelination in secondary progressive MS. An important factor in age-related myelin loss is a marked decrease in the regenerative capacity of OPCs. In this review, we will contextualize recent advances in the key role of Epidermal Growth Factor (EGF) signaling in regulating multiple biological pathways in oligodendroglia that are dysregulated in aging.
Introduction
Brain aging is characterized by a slowing down of sensory, cognitive and behavioral processes (Harada et al., 2013). Notably, brain imaging studies in humans have demonstrated shrinkage of white matter that precedes overt loss of neurons and appears to be accelerated in dementia (Bartzokis et al., 2012; Maniega et al., 2015; Cox et al., 2016). White matter is comprised of myelinated axons which are thin protrusions of the neurons that transmit electrical signals between the different parts of the central nervous system (CNS). Myelin is a lipid-rich insulating layer that is wrapped around axons in concentrical lamellae by terminally differentiated glial cells called oligodendrocytes (OLs). Myelin increases the propagation speed of electrical signaling along the length of an axon by saltatory conduction. Moreover, myelin has numerous emerging roles that includes, providing metabolic support (Fünfschilling et al., 2007; Philips and Rothstein, 2017), memory consolidation (Pan et al., 2020; Steadman et al., 2020), task-associated learning experiences (Kato et al., 2020; Wang et al., 2020), and reviewed by (Pan and Chan, 2021), whilst myelin loss renders axons more vulnerable to damage (Smith, 2006). Once developmental myelination is complete, myelin remodeling continues throughout life via a reservoir of oligodendrocyte progenitors (OPCs) which are the main proliferating pool of cells in the adult CNS and possess the stem-cell-like feature of self-renewal (Nishiyama et al., 2021). The life-long generation of OLs from OPCs is essential to produce new myelin required to insulate new brain connections formed in response to new life experiences and to replace myelin lost through natural “wear-tear” or pathology (Rivera et al., 2016). However, the regenerative power of OPCs declines with age leading to impaired oligodendrogenesis and myelin remodeling, and an overall gradual loss of major CNS functions such as spatial learning and memory (Pan et al., 2020; Steadman et al., 2020; Wang et al., 2020). The age-related impairments in OPC differentiation have been discussed in a number of recent reviews (for example, Rivera et al., 2021a; Butt et al., 2019). Moreover, in many age-related neuropathologies such as AD or secondary progressive MS, due to a number of reasons that include and are not limited to the inflammatory environment, excess inhibitory myelin debris, lack of appropriate trophic support, etc, OPC differentiation drastically fails and contributes to the loss in cognitive function (Neumann et al., 2019; Wang et al., 2020; Coelho et al., 2021; Rivera et al., 2021a,b). Currently, the development of treatments to halt these changes is hampered by gaps in fundamental scientific knowledge. Developmental studies propose a positive role of epidermal growth factor (EGF) acting via its main receptor, EGFR, as a key regulator of cell survival, proliferation, migration and differentiation which are disrupted in aging (Figure 1; Herbst, 2004; Gonzalez-Perez et al., 2009; Galvez-Contreras et al., 2013; Yang et al., 2017). To the best of our knowledge, functional studies of EGF signaling in the context of OL differentiation during later stages of adulthood have yet to be performed. Nevertheless, these are exciting future avenues in the field as a potential therapeutic target in OL pathologies and aging.
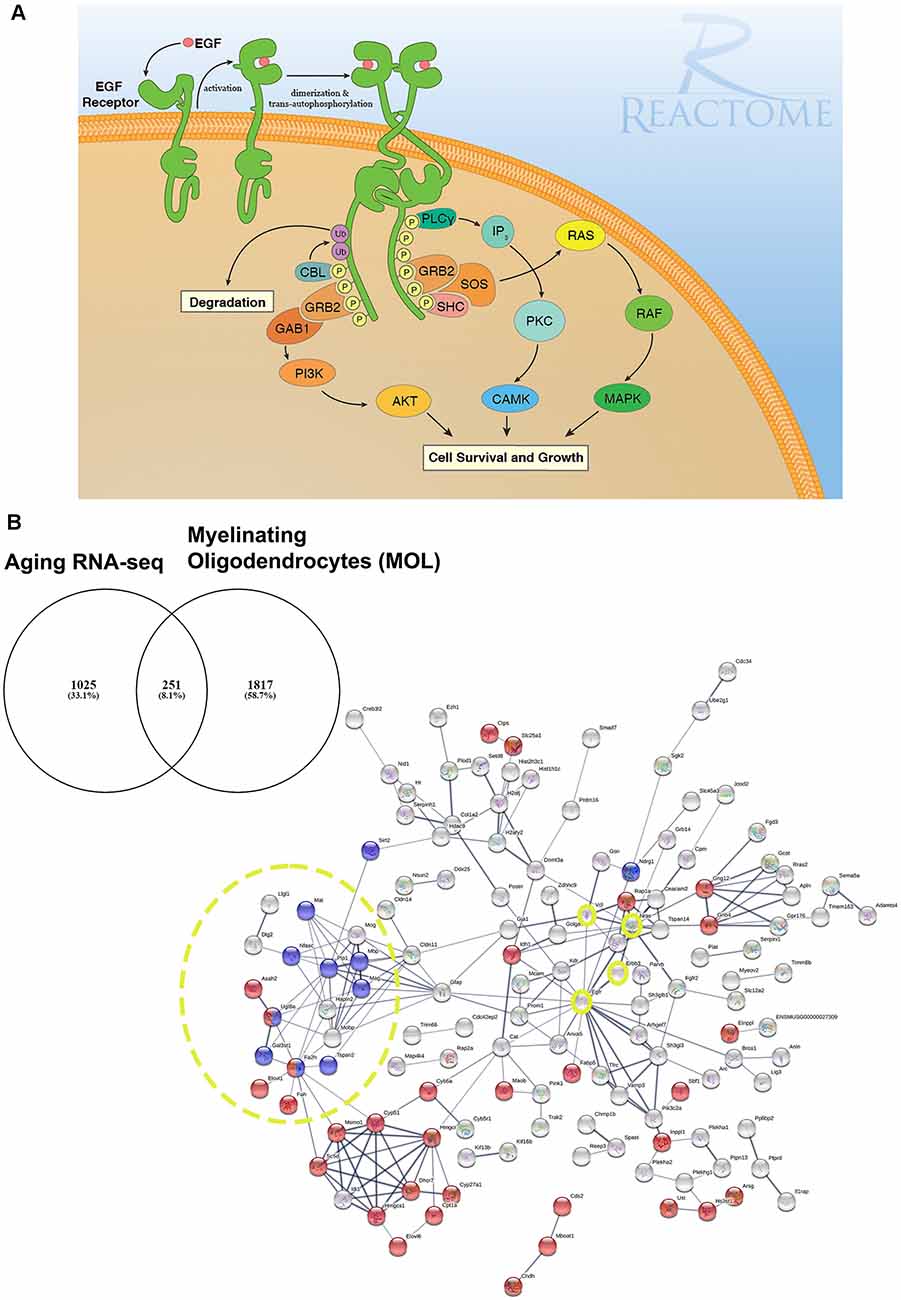
Figure 1. EGF receptor signaling and resolving its dysregulation in aged oligodendroglial via protein-protein network analysis. (A) EGFR is a member of ERBB receptors that belong to the superfamily of Receptor Tyrosine Kinases (RTKs). The binding of ligands to EGFR induces conformational changes resulting in the receptor homo- or heterodimerization at the cell surface. Dimerization of the extracellular regions of EGFR cascades results in further conformational change at the cytoplasmic region of the receptor, leading to the activation of the catalytic domain. EGFR dimers trans-autophosphorylate on tyrosine residues in the cytoplasmic tail becoming binding sites for the recruitment of intracellular modulator for downstream signaling cascades. Recruitment of complexes containing GRB2 and SOS1 directly through GRB2 or indirectly through SHC1 promotes the activation of RAS/RAF/MAP kinase signaling. The binding of GRB2 and GAB1 to phosphorylated EGFR leads to the activation of PI3K/AKT signaling cascade. Finally, PLCγcan be recruited to the phosphorylated EGFR which, in turn, activates IP3/PKC signaling. Image generated from REACTOME “Signaling by EGFR” (https://reactome.org/PathwayBrowser/#/R-HSA-177929). (B) RNA-seq transcriptome analysis of the aging murine brain was compared to a database of genes expressed by myelinating OLs (MOL) and 251 genes were identified as significantly altered in aging (Rivera et al., 2021b). (B) Functional protein-protein network analysis identified EGFR as centrally connected with ERBB3, NRAS, VCL, GSN, CLDN11, and the myelination node (yellow circles). Red nodes represent genes associated with Metabolism (p < 0.000034) and blue nodes represent genes associated with Myelination (p < 5.55e-07). PPI enrichment p-value < : 2.44e-15. Adapted from Rivera et al. (2021b).
Oligodendrocyte and Myelin Disruption in The Aging Brain
Age-related loss of brain connectivity underlies cognitive decline, with a “last in, first out” pattern, whereby white matter tracts associated with cognition are the “last” to be fully myelinated and the first to be lost in aging (Davis et al., 2009; Bartzokis et al., 2012; Gozdas et al., 2020). This process is the result of brain architectural complexity described as heterochronicity and spatial heterogeneity intrinsic in white matter tracts. In addition, it suggests that the latest tracts to develop are the most vulnerable to the deleterious effects of aging (Cox et al., 2016; Kochunov et al., 2016). Post-mortem diffusion magnetic resonance imaging (dMRI) studies indicate ontogenetic differences between early-myelinating projection and posterior callosal fibers in aging (Sexton et al., 2014; Cox et al., 2016; Slater et al., 2019). Although the precise causes of WM shrinkage are currently unresolved, they include metabolic disruption of oligodendroglia, OPC senescence and loss of extracellular trophic factors that support OPCs and OLs which can contribute to the functional decline of brain function including deficits in spatial memory and learning (Rivera et al., 2016; 2021b; Neumann et al., 2019; Kato et al., 2020; Pan et al., 2020; Steadman et al., 2020; Willis et al., 2020). Several studies in both humans and rodents have demonstrated marked changes in the transcriptome of OLs and myelination processes (Soreq et al., 2017; Neumann et al., 2019; Rivera et al., 2021b). Moreover, alterations in OPC densities have been reported in brain aging (Soreq et al., 2017; Rivera et al., 2021b). The age-related disruption of indispensable signaling pathway components hinders myelin remodeling and repair, and ultimately adds to the cumulative loss of myelin, which is aggravated in pathology. Recently, we have demonstrated that the critical OPC protein GPR17 is downregulated in the aged murine brain, together with myelin-related transcripts such as MBP, PLP1, CNP, and UGT8A (Rivera et al., 2021b). Our transcriptomic analysis identified a central role for age-related changes in EGFR signaling in oligodendroglia, consistent with its recognized importance in OLregeneration and myelin repair (Aguirre et al., 2007; Hayakawa-Yano et al., 2007; Ivkovic et al., 2008).
Unraveling Novel Roles of EGFR Signaling in Aged Oligodendroglia
In our network analyses (Figure 1B), we identified EGFR association with myelination via its interaction with ERBB3 which is required for OL maturation (Schmucker et al., 2003; Makinodan et al., 2012). ERBB3 is coupled to the Ras family member NRAS which has intrinsic GTPase activity and is involved in the control of cell proliferation, regulating microtubule stability and actin polymerization (Fotiadou et al., 2007), and is implicated in cancer pathways (Bronte et al., 2015). Interestingly, NRAS has recently been reported to be elevated in expression in the aging OPC proteome (de la Fuente et al., 2020). NRAS interacts with VCL (vinculin) and CLDN11 (claudin-11) to regulate OL morphogenesis/myelin growth (Nawaz et al., 2015), or the formation of tight junctions (TJs) with ECM-integrin interactions, respectively (Gow et al., 1999; Bronstein et al., 2000; Tiwari-Woodruff et al., 2001). Notably, EGFRs are mechanosensitive (Tschumperlin, 2004; Müller-Deubert et al., 2017), transduced by vinculin (including Talin and similar linker proteins) to regulate the anchoring of the actin cytoskeleton to the ECM through integrin, leading to cytoskeleton regulation and cellular spreading (Rübsam et al., 2017). Our analysis predicted the interaction of vinculin on with the actin cytoskeleton via gelsolin (GSN), which is enriched in OLs (Tanaka and Sobue, 1994; Zhang et al., 2014) and is required for myelination (Liu et al., 2003; Zuchero et al., 2015). Intriguingly, vinculin and gelsolin are focal adhesion proteins important for the association of cell-cell and cell-matrix junctions and are critical for controlling cell spreading, cytoskeletal mechanics, and lamellipodia formation (Ciobanasu et al., 2014; Elosegui-Artola et al., 2016; Argentati et al., 2019; Merkel et al., 2019; Muñoz-Lasso et al., 2020). Moreover, the interaction of EGFR and vinculin with CLDN11 is consistent with the evidence that they mediate cell/integrin/ECM interactions (Hagen, 2017). Recent in vivo experiments in which CLDN11 was deleted in OLs have shown dysregulation of myelin with subsequent aberrant behavioral changes due to increased latency of signals (Maheras et al., 2018). The ECM plays a pivotal role in OL differentiation (Lourenço and Grãos, 2016) and increased stiffness of the ECM is related to age-related deterioration of OPC function (Segel et al., 2019). Our data implicate for the first time the EGFR-VINCULIN-GELSOLIN-CLDN11 network as key to age-related changes in oligodendroglial ECM interactions.
EGFR and Their Roles in Oligodendroglia
Overview of EGFR Ligands and Receptors in the CNS
The EGFR, also known as ERBB1 or HER-1, and its family of ligands are widely expressed across the CNS. EGFR, together with ERBB2, ERBB3, ERBB4 belong to the receptor tyrosine kinases (RTKs) superfamily (reviewed extensively elsewhere, for example, Novak et al., 2001; Fu et al., 2003; Galvez-Contreras et al., 2013). Canonical ligands include: epidermal growth factor (EGF), transforming growth factor-α (TGFα), Heparin-binding EGF (HB-EGF) B-cellulin (BTC) and low-affinity binding ligands such as neuregulins (NRG 1–4) amphiregulin (AR) and epiregulin (EPR; Figure 1A; Knudsen et al., 2014; Singh et al., 2016). In young adult mice, bulk transcriptomic analysis could resolve their detection across multiple cell types where most of these are expressed by the vasculature, choroid plexus (TGFα), or astrocytes (HB-EGF; Azim et al., 2018). It remains to be determined which of these are altered during aging and are aspects which will be addressed in follow-up studies using the same procedures done in older mice.
The major downstream effectors of EGFR signaling are described in Figure 1A illustrating the RAS/RAF/ERK1-2/STAT3-5 and the PI3K/AKT protein complexes are fundamental regulators of many OL biological processes aside from the EGFR signaling pathway (Ishii et al., 2014, 2019; Azim et al., 2017; Sanz-Rodriguez et al., 2018; Rivera et al., 2021b). The precise interaction of these kinases to the newly identified EGFR-VINCULIN-GELSOLIN-CLDN11 network remains to be resolved.
EGFR Signaling in Oligodendroglia and Myelination
Recent transcriptomic studies have shed further light on the expression of EGFR and ERRB2–4 in developmental and adult human OL lineage cells demonstrating elevated expression in OPC compared to other CNS cell types (Zhang et al., 2014; Jäkel et al., 2019). In vivo gain- and loss-of-function studies underlined the critical importance of EGFR in OLs (Aguirre et al., 2007, 2010). Specifically, overexpression of EGFR enhanced the densities and maturation state of myelinating oligodendrocytes (MOL; Aguirre et al., 2007), which may be owed to sustained AKT phosphorylation in post-mitotic immature OLs (Flores et al., 2000, 2008) via the Src homology 2-containing phosphotyrosine phosphatase (SHP2) which integrates EGFR to AKT signaling (Agazie and Hayman, 2003; Zhu et al., 2010; Nocita et al., 2019). Similarly, the Grb2 associated binder 1 (GAB1) is another modulator of PI3K/AKT signaling capable of directing oligodendrogenesis via EGFR (Holgado-Madruga et al., 1996; Hayakawa-Yano et al., 2007). In vitro studies indicated that EGF interacts with PDGF-AA and FGF to direct early glial progenitors toward the OL fate, suggesting the combinatorial role for these two pathways towards oligodendrogenesis (Yang et al., 2017). Intriguingly, OPCs cultured with EGF in the absence of PDGF-AA are driven to differentiate into MOLs, suggesting a dual role of EGF in the control of oligodendrogenesis and myelination, depending on the activation of other signaling pathways (Yang et al., 2017). Similarly, intraventricular administration of EGF promotes subventricular progenitors to differentiate into OPCs and MOL in vivo (Gonzalez-Perez et al., 2009; Galvez-Contreras et al., 2013). Furthermore, overexpression of human-EGFR (hEGFR) in CNP+ OLs leads to an increase in OL densities and remyelination of the corpus callosum following a lesion (Aguirre et al., 2007). However, persistent overexpression of EGFR in OPCs leads to their dramatic enhancement in densities and white matter hyperplasia, although differentiation appears to be hindered (Hayakawa-Yano et al., 2007; Ivkovic et al., 2008; Gonzalez-Perez et al., 2009). Finally, intranasal administration of exogenous heparin-binding EGF (HB-EGF) in a neonatal hypoxia murine model reduced apoptosis of MOL preserving axonal myelination (Scafidi et al., 2014). These results suggest that the control of oligodendrogenesis and myelination requires temporal and cell-specific interplay of EGFR with other unknown signaling pathways. OL lineage cells also express ERBB2–4 which are required depending on the maturation stage (Flores et al., 2000; Park et al., 2001; Yarden and Sliwkowski, 2001; Kim et al., 2003; Schmucker et al., 2003; Makinodan et al., 2012). For example, ERBB2 regulates OL proliferation and differentiation, while ERBB3 and ERBB4 are necessary for maturation and myelination (Park et al., 2001; Schmucker et al., 2003; Roy et al., 2007; Joubert et al., 2010; Makinodan et al., 2012). However, overexpression or excessive activation of ERBBs in defined stages of OLs can lead to inflammation, demyelination, and cell death (Hu et al., 2021). Taken together, EGFR/ERBB signaling has key roles in the regulation of defined stages of OLs and white matter formation in the CNS.
EGFR as A Potential Therapeutic Strategy in Age-Related White Matter Loss
Studies on preclinical murine animal models and human post-mortem tissue have demonstrated downregulation of EGFR in the aging brain (Hiramatsu et al., 1988; Werner et al., 1988). Moreover, EGFR signaling is disturbed in aging and gain and loss of function experiments in vivo posit the idea of the presence of undiscovered factors in the aging CNS that limit its efficacy in positively regulating biological processes required for proliferation and differentiation of NSCs (Cochard et al., 2021). To further investigate this, we have interrogated the transcriptome of the aging murine brain (Rivera et al., 2021b) with genes associated with Alzheimer’s Disease (AD) and Multiple Sclerosis (MS) using the DISGENET database (Figure 2; Pinero et al., 2015). These analyses identified 60 AD-associated genes that are altered in aged MOLs, with an apparent EGFR-ANXA5-GSN-APOD axis interconnected with a myelin gene hub; ANXA5 (annexin 5) is involved in pathogenesis through autophagy mechanisms (Iannaccone et al., 2015; Xi et al., 2020), and APOD (apolipoprotein D) is a secreted glycoprotein involved in lipid transport that is linked to AD, MS and other neuroinflammatory diseases (Reindl et al., 2001; Li et al., 2015). In the same way, analysis of oligodendroglial genes altered in aging and associated with MS revealed 34 highly correlated genes with a conserved EGFR-VCL-GSN-APOD network associated with myelin genes. In MS, APOD levels are decreased in sclerotic plaques and elevated during remyelination (Navarro et al., 2018). Hence, identifying small molecules that target these networks has promising therapeutic potential for rejuvenating OPCs in aging contexts. A novel approach is to use genomics and chemical informatics data, most notably the connectivity map (CMAP) and the Library of Integrated Network-based Cellular Signatures (LINCS), which enables the identification of small molecules that counteract disease-specific transcriptional profiles (Azim et al., 2017; Rivera et al., 2022a). In this way, we recently identified the PI3K-Akt inhibitor LY294002 as a potent driver of OPC rejuvenation and myelin repair in vivo (Rivera et al., 2022a, b). Notably, transcriptional profiling and signaling pathway activity assays identified EGFR signaling as a target of LY294002 in OPCs and GO analysis of LY294002-responsive oligodendroglial genes indicated a central role for Rhoa at the core of ERBB3 signaling, which regulates oligodendrogenesis via RAF-MAPK and PI3K/Akt (Rivera et al., 2022a). These studies demonstrate that transcript-specific targeting using pharmacogenomics approaches streamlines the identification of drugs to target EGFR signaling, and can be readily adapted to probe genes and transcriptional networks of interest for driving rejuvenation and myelin repair.
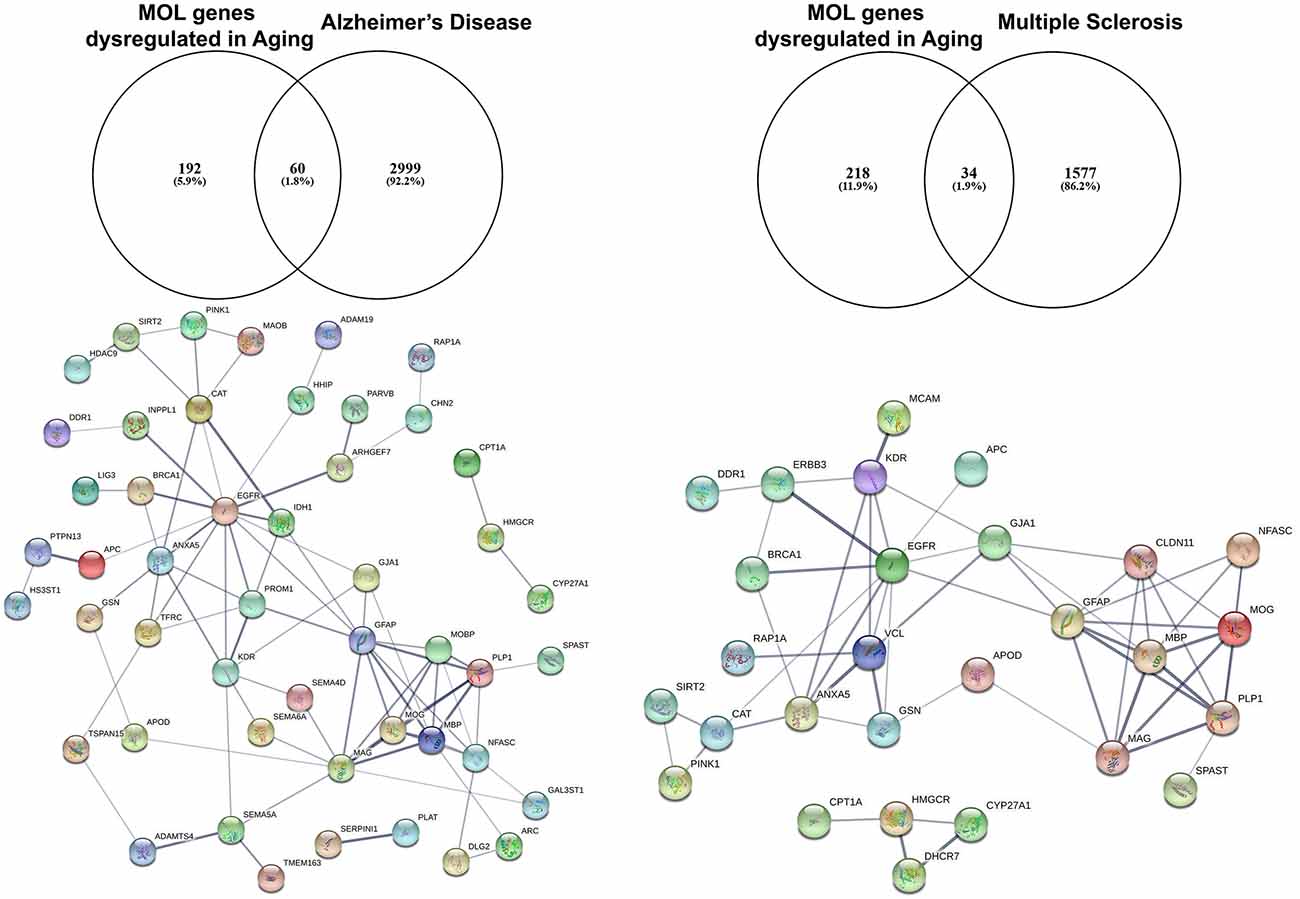
Figure 2. Identification of altered EGFR signaling in aged myelinating oligodendrocytes are further associated as altered genes in Alzheimer’s disease and multiple sclerosis. The aging myelinating OLtranscriptome was interrogated to identify novel associations within disease-specific databases for Alzheimer’s disease and multiple sclerosis. Functional protein–protein prediction analysis identified EGFR at the core of the networks for Alzheimer’s disease and multiple sclerosis (PPI enrichment p < 0.0001).
Conclusion
In summary, EGFR signaling and its subsequent signaling cascade depends on the combination of ERBB receptors activated. EGFR signaling is central for the OPC self-renewal and their differentiation into MOLs. In the aged brain, there is a decline in the regenerative capacity of OPCs and this is highly correlated with changes in EGFR signaling pathways. Moreover, we have recently shown that targeting PI3K/AKT signaling, which is a key downstream mechanism of EGFR, promotes OPC regeneration and remyelination in an aging context (Rivera et al., 2022a, b). These data support a key role for EGFR as a potential therapeutic target for rejuvenating OPCs and promoting repair in pathologies with age-related contexts, including MS and AD.
Author Contributions
AR: conceptualization, formal analysis, investigation, methodology, writing—original draft, writing—review and editing. KA: formal analysis, investigation, writing—review and editing. VM and AP: supervision, writing—review and editing. AB and RD: conceptualization, formal analysis, funding acquisition, project administration, resources, supervision, visualization, writing—original draft, writing—review and editing.
Funding
This work was supported by grants from the Biotechnology and Biological Sciences Research Council (BBSRC, UK) (BB/M029379/1), Medical Research Council (MRC, UK) (MR/P025811/1), MSCA Seal of Excellence @ UNIPD and NVIDIA Hardware Grant, German Research Council (AZ/115/1-1; AZ/115/1-3), and Swiss National Funds (P300PA_171224).
Conflict of Interest
AR and AB are shareholders of Gliagenesis LTD. The remaining authors declare that the research was conducted in the absence of any commercial or financial relationships that could be construed as a potential conflict of interest.
Publisher’s Note
All claims expressed in this article are solely those of the authors and do not necessarily represent those of their affiliated organizations, or those of the publisher, the editors and the reviewers. Any product that may be evaluated in this article, or claim that may be made by its manufacturer, is not guaranteed or endorsed by the publisher.
References
Agazie, Y. M., and Hayman, M. J. (2003). Molecular mechanism for a role of SHP2 in epidermal growth factor receptor signaling. Mol. Cell Biol. 23, 7875–7886. doi: 10.1128/MCB.23.21.7875-7886.2003
Aguirre, A., Dupree, J. L., Mangin, J. M., and Gallo, V. (2007). A functional role for EGFR signaling in myelination and remyelination. Nat. Neurosci. 10, 990–1002. doi: 10.1038/nn1938
Aguirre, A., Maria Rubio, E., and Vittorio, G. (2010). Notch and EGFR pathway interaction regulates neural stem cell number and self-renewal. Nature 467, 323–327. doi: 10.1038/nature09347
Argentati, C., Morena, F., Tortorella, I., Bazzucchi, M., Porcellati, S., Emiliani, C., et al. (2019). Insight into mechanobiology: how stem cells feel mechanical forces and orchestrate biological functions. Int. J. Mol. Sci. 20:5337. doi: 10.3390/ijms20215337
Azim, K., Angonin, D., Marcy, G., Pieropan, F., Rivera, A., Donega, V., et al. (2017). Pharmacogenomic identification of small molecules for lineage specific manipulation of subventricular zone germinal activity. PLoS Biol. 15:e2000698. doi: 10.1371/journal.pbio.2000698
Azim, K., Rainer, A., Martina, C., Julio, V., Janusz Jadasz, J., and Patrick, K. (2018). Transcriptional profiling of ligand expression in cell specific populations of the adult mouse forebrain that regulates neurogenesis. Front. Neurosci. 12:220. doi: 10.3389/fnins.2018.00220
Bartzokis, G., Po Lu, H., Panthea, H., Alexander, C., Grace Lee, J., Greta, K., et al. (2012). Multimodal magnetic resonance imaging assessment of white matter aging trajectories over the lifespan of healthy individuals. Biol. Psychiatry 72, 1026–1034. doi: 10.1016/j.biopsych.2012.07.010
Bronstein, J. M., Tiwari-Woodruff, S., Buznikov, A. G., and Stevens, D. B. (2000). Involvement of OSP/claudin-11 in oligodendrocyte membrane interactions, role in biology and disease. J. Neurosci. Res. 59, 706–711. doi: 10.1002/(SICI)1097-4547(20000315)59:6<706::AID-JNR2>3.0.CO;2-D
Bronte, G., Silvestris, N., Castiglia, M., Galvano, A., Passiglia, F., Sortino, G., et al. (2015). New findings on primary and acquired resistance to anti-EGFR therapy in metastatic colorectal cancer: do all roads lead to RAS. Oncotarget 6, 24780–24796. doi: 10.18632/oncotarget.4959
Butt, A. M., De La Rocha, I. C., and Rivera, A. (2019). Oligodendroglial cells in Alzheimer’s disease. Adv. Exp. Med. Biol. 1175, 325–333. doi: 10.1007/978-981-13-9913-8_12
Ciobanasu, C., Faivre, B., and Le Clainche, C. (2014). Actomyosin-dependent formation of the mechanosensitive talin-vinculin complex reinforces actin anchoring. Nat. Commun. 5:3095. doi: 10.1038/ncomms4095
Cochard, L. M., Louis-Charles, L., Sandra, E., Joppé, F. P., Anne, A., and Karl Fernandes, J. L. (2021). Manipulation of EGFR-induced signaling for the recruitment of quiescent neural stem cells in the adult mouse forebrain. Front. Neurosci. 15:621076. doi: 10.3389/fnins.2021.621076
Coelho, A., Henrique Fernandes, M., Ricardo, M., Moreira, P. S., Marques, P., José Soares, M., et al. (2021). Signatures of white-matter microstructure degradation during aging and its association with cognitive status. Sci. Rep. 11:4517. doi: 10.1038/s41598-021-83983-7
Cox, S. R., Ritchie, S. J., Tucker-Drob, E. M., Liewald, D. C., Hagenaars, S. P., Davies, G., et al. (2016). Ageing and brain white matter structure in 3,513 UK Biobank participants. Nat. Commun. 7:13629. doi: 10.1038/ncomms13629
Davis, S. W., Dennis, N. A., Buchler, N. G., White, L. E., Madden, D. J., and Cabeza, R. (2009). Assessing the effects of age on long white matter tracts using diffusion tensor tractography. Neuroimage 46, 530–541. doi: 10.1016/j.neuroimage.2009.01.068
de la Fuente, A. G., Queiroz, R. M. L., Ghosh, T., McMurran, C. E., Cubillos, J. F., Bergles, D. E., et al. (2020). Changes in the oligodendrocyte progenitor cell proteome with ageing. Mol. Cell. Proteomics 19, 1281–1302. doi: 10.1074/mcp.RA120.002102
Elosegui-Artola, A., Oria, R., Chen, Y., Kosmalska, A., Pérez-González, C., Castro, N., et al. (2016). Mechanical regulation of a molecular clutch defines force transmission and transduction in response to matrix rigidity. Nat. Cell Biol. 18, 540–548. doi: 10.1038/ncb3336
Flores, A. I., Mallon, B. S., Matsui, T., Ogawa, W., Rosenzweig, A., Okamoto, T., et al. (2000). Akt-mediated survival of oligodendrocytes induced by neuregulins. J. Neurosci. 20, 7622–7630. doi: 10.1523/JNEUROSCI.20-20-07622.2000
Flores, A. I., Narayanan, S. P., Morse, E. N., Shick, H. E., Yin, X., Kidd, G., et al. (2008). Constitutively active Akt induces enhanced myelination in the CNS. J. Neurosci. 28, 7174–7183. doi: 10.1523/JNEUROSCI.0150-08.2008
Fotiadou, P. P., Takahashi, C., Rajabi, H. N., and Ewen, M. E. (2007). Wild-type NRas and KRas perform distinct functions during transformation. Mol. Cell. Biol. 27, 6742–6755. doi: 10.1128/MCB.00234-07
Fu, L., Abu-Khalil, A., Morrison, R. S., Geschwind, D. H., and Kornblum, H. I. (2003). Expression patterns of epidermal growth factor receptor and fibroblast growth factor receptor 1 mRNA in fetal human brain. J. Comp. Neurol. 462, 265–273. doi: 10.1002/cne.10727
Fünfschilling, U., Saher, G., Xiao, L., Möbius, W., and Nave, K.-A. (2007). Survival of adult neurons lacking cholesterol synthesis in vivo. BMC Neurosci. 8:1. doi: 10.1186/1471-2202-8-1
Galvez-Contreras, A., Quinones-Hinojosa, A., and Gonzalez-Perez, O. (2013). The role of EGFR and ErbB family related proteins in the oligodendrocyte specification in germinal niches of the adult mammalian brain. Front. Cell. Neurosci. 7:258. doi: 10.3389/fncel.2013.00258
Gonzalez-Perez, O., Romero-Rodriguez, R., Soriano-Navarro, M., Garcia-Verdugo, J. M., and Alvarez-Buylla, A. (2009). Epidermal growth factor induces the progeny of subventricular zone type B cells to migrate and differentiate into oligodendrocytes. Stem Cells 27, 2032–2043. doi: 10.1002/stem.119
Gow, A., Southwood, C. M., Li, J. S., Pariali, M., Riordan, G. P., Brodie, S. E., et al. (1999). CNS myelin and sertoli cell tight junction strands are absent in Osp/claudin-11 null mice. Cell 99, 649–659. doi: 10.1016/s0092-8674(00)81553-6
Gozdas, E., Fingerhut, H., Chromik, L. C., O’Hara, R., Reiss, A. L., and Hadi Hosseini, S. M. (2020). Focal white matter disruptions along the cingulum tract explain cognitive decline in amnestic mild cognitive impairment (aMCI). Sci. Rep. 10:10213. doi: 10.1038/s41598-020-66796-y
Hagen, S. J. (2017). Non-canonical functions of claudin proteins: beyond the regulation of cell-cell adhesions. Tissue Barriers 5:e1327839. doi: 10.1080/21688370.2017.1327839
Harada, C. N., Natelson Love, M. C., and Triebel, K. L. (2013). Normal cognitive aging. Clin. Geriat. Med. 29, 737–752. doi: 10.1016/j.cger.2013.07.002
Hayakawa-Yano, Y., Nishida, K., Fukami, S., Gotoh, Y., Hirano, T., Nakagawa, T., et al. (2007). Epidermal growth factor signaling mediated by grb2 associated binder1 is required for the spatiotemporally regulated proliferation of olig2-expressing progenitors in the embryonic spinal cord. Stem Cells 25, 1410–1422. doi: 10.1634/stemcells.2006-0584
Herbst, R. S. (2004). Review of epidermal growth factor receptor biology. Int. J. Radiat. Oncol. Biol. Phys. 59, 21–26. doi: 10.1016/j.ijrobp.2003.11.041
Hiramatsu, M., Kashimata, M., Sato, A., Murayama, M., and Minami, N. (1988). Influence of age on epidermal growth factor receptor level in the rat brain. Experientia 44, 23–25. doi: 10.1007/BF01960230
Holgado-Madruga, M., Emlet, D. R., Moscatello, D. K., Godwin, A. K., and Wong, A. J. (1996). A Grb2-associated docking protein in EGF- and insulin-receptor signalling. Nature 379, 560–564. doi: 10.1038/379560a0
Hu, X., Xiao, G., He, L., Niu, X., Li, H., Lou, T., et al. (2021). Sustained ErbB activation causes demyelination and hypomyelination by driving necroptosis of mature oligodendrocytes and apoptosis of oligodendrocyte precursor cells. J. Neurosci. 41, 9872–9890. doi: 10.1523/JNEUROSCI.2922-20.2021
Iannaccone, A., Giorgianni, F., New, D. D., Hollingsworth, T. J., Umfress, A., Alhatem, A. H., et al. (2015). Circulating autoantibodies in age-related macular degeneration recognize human macular tissue antigens implicated in autophagy, immunomodulation and protection from oxidative stress and apoptosis. PLoS One 10:e0145323. doi: 10.1371/journal.pone.0145323
Ishii, A., Furusho, M., Dupree, J. L., and Bansal, R. (2014). Role of ERK1/2 MAPK signaling in the maintenance of myelin and axonal integrity in the adult CNS. J. Neurosci. 34, 16031–16045. doi: 10.1523/JNEUROSCI.3360-14.2014
Ishii, A., Furusho, M., Macklin, W., and Bansal, R. (2019). Independent and cooperative roles of the Mek/ERK1/2-MAPK and PI3K/Akt/mTOR pathways during developmental myelination and in adulthood. Glia 67, 1277–1295. doi: 10.1002/glia.23602
Ivkovic, S., Canoll, P., and Goldman, J. E. (2008). Constitutive EGFR signaling in oligodendrocyte progenitors leads to diffuse hyperplasia in postnatal white matter. J. Neurosci. 28, 914–922. doi: 10.1523/JNEUROSCI.4327-07.2008
Jäkel, S., Agirre, E., Falcão, A. M., Bruggen, D. V., Lee, K. W., Knuesel, I., et al. (2019). Altered human oligodendrocyte heterogeneity in multiple sclerosis. Nature 566, 543–547. doi: 10.1038/s41586-019-0903-2
Joubert, L., Foucault, I., Sagot, Y., Bernasconi, L., Duval, F., Alliod, C., et al. (2010). Chemical inducers and transcriptional markers of oligodendrocyte differentiation. J. Neurosci. Res. 88, 2546–2557. doi: 10.1002/jnr.22434
Kato, D., Wake, H., Lee, P. R., Tachibana, Y., Ono, R., Sugio, S., et al. (2020). Motor learning requires myelination to reduce asynchrony and spontaneity in neural activity. Glia 68, 193–210. doi: 10.1002/glia.23713
Kim, J. Y., Sun, Q., Oglesbee, M., and Yoon, S. O. (2003). The role of ErbB2 signaling in the onset of terminal differentiation of oligodendrocytes in vivo. J. Neurosci. 23, 5561–5571. doi: 10.1523/JNEUROSCI.23-13-05561.2003
Knudsen, S. L., Mac, A. S., Henriksen, L., van Deurs, B., and Grøvdal, L. M. (2014). EGFR signaling patterns are regulated by its different ligands. Growth Factors 32, 155–163. doi: 10.3109/08977194.2014.952410
Kochunov, P., Ganjgahi, H., Winkler, A., Kelly, S., Shukla, D. K., Du, X., et al. (2016). Heterochronicity of white matter development and aging explains regional patient control differences in schizophrenia. Hum. Brain Mapp. 37, 4673–4688. doi: 10.1002/hbm.23336
Li, H., Ruberu, K., Muñoz, S. S., Jenner, A. M., Spiro, A., Zhao, H., et al. (2015). Apolipoprotein D modulates amyloid pathology in APP/PS1 Alzheimers disease mice. Neurobiol. Aging 36, 1820–1833. doi: 10.1016/j.neurobiolaging.2015.02.010
Liu, A., Muggironi, M., Marin-Husstege, M., and Casaccia-Bonnefil, P. (2003). Oligodendrocyte process outgrowth in vitro is modulated by epigenetic regulation of cytoskeletal severing proteins. Glia 44, 264–274. doi: 10.1002/glia.10290
Lourenço, T., and Grãos, M. (2016). Modulation of oligodendrocyte differentiation by mechanotransduction. Front. Cell. Neurosci. 10:277. doi: 10.3389/fncel.2016.00277
Maheras, K. J., Peppi, M., Ghoddoussi, F., Galloway, M. P., Perrine, S. A., and Gow, A. (2018). Absence of claudin 11 in CNS myelin perturbs behavior and neurotransmitter levels in mice. Sci. Rep. 8:3798. doi: 10.1038/s41598-018-22047-9
Makinodan, M., Rosen, K. M., Ito, S., and Corfas, G. (2012). A critical period for social experience-dependent oligodendrocyte maturation and myelination. Science 337, 1357–1360. doi: 10.1126/science.1220845
Maniega, S. M., Valdes Hernandez, M. C., Clayden, J. D., Royle, N. A., Murray, C., Morris, Z., et al. (2015). White matter hyperintensities and normal-appearing white matter integrity in the aging brain. Neurobiol. Aging 36, 909–918. doi: 10.1016/j.neurobiolaging.2014.07.048
Merkel, C. D., Li, Y., Raza, Q., Stolz, D. B., and Kwiatkowski, A. V. (2019). Vinculin anchors contractile actin to the cardiomyocyte adherens junction. Mol. Biol. Cell 30, 2639–2650. doi: 10.1091/mbc.E19-04-0216
Müller-Deubert, S., Seefried, L., Krug, M., Jakob, F., and Ebert, R. (2017). Epidermal growth factor as a mechanosensitizer in human bone marrow stromal cells. Stem Cell Res. 24, 69–76. doi: 10.1016/j.scr.2017.08.012
Muñoz-Lasso, D. C., Romá-Mateo, C., Pallardó, F. V., and Gonzalez-Cabo, P. (2020). Much more than a scaffold: cytoskeletal proteins in neurological disorders. Cells 9:358. doi: 10.3390/cells9020358
Navarro, A., Rioseras, B., del Valle, E., Martínez-Pinilla, E., Astudillo, A., and Tolivia, J. (2018). Expression pattern of myelin-related apolipoprotein D in human multiple sclerosis lesions. Front. Aging Neurosci. 10:254. doi: 10.3389/fnagi.2018.00254
Nawaz, S., Sánchez, P., Schmitt, S., Snaidero, N., Mitkovski, M., Velte, C., et al. (2015). Actin filament turnover drives leading edge growth during myelin sheath formation in the central nervous system. Dev. Cell 34, 139–151. doi: 10.1016/j.devcel.2015.05.013
Neumann, B., Baror, R., Zhao, C., Segel, M., Dietmann, S., Rawji, K. S., et al. (2019). Metformin restores CNS remyelination capacity by rejuvenating aged stem cells. Cell Stem Cell 25, 473–485.e8. doi: 10.1016/j.stem.2019.08.015
Nishiyama, A., Shimizu, T., Sherafat, A., and Richardson, W. D. (2021). Life-long oligodendrocyte development and plasticity. Semin. Cell Dev. Biol. 116, 25–37. doi: 10.1016/j.semcdb.2021.02.004
Nocita, E., Giovane, A. D., Tiberi, M., Boccuni, L., Fiorelli, D., Sposato, C., et al. (2019). EGFR/ErbB inhibition promotes OPC maturation up to axon engagement by co-regulating PIP2 and MBP. Cells 8:844. doi: 10.3390/cells8080844
Novak, U., Walker, F., and Kaye, A. (2001). Expression of EGFR-family proteins in the brain: role in development, health and disease. J. Clin. Neurosci. 8, 106–111. doi: 10.1054/jocn.2000.0799
Pan, S., and Chan, J. R. (2021). Clinical applications of myelin plasticity for remyelinating therapies in multiple sclerosis. Ann. Neurol. 90, 558–567. doi: 10.1002/ana.26196
Pan, S., Mayoral, S. R., Sun Choi, H., Chan, J. R., and Kheirbek, M. A. (2020). Preservation of a remote fear memory requires new myelin formation. Nat. Neurosci. 23, 487–499. doi: 10.1038/s41593-019-0582-1
Park, S. K., Miller, R., Krane, I., and Vartanian, T. (2001). The erbB2 gene is required for the development of terminally differentiated spinal cord oligodendrocytes. J. Cell Biol. 154, 1245–1258. doi: 10.1083/jcb.200104025
Philips, T., and Rothstein, J. D. (2017). Oligodendroglia: metabolic supporters of neurons. J. Clin. Invest. 127, 3271–3280. doi: 10.1172/JCI90610
Pinero, J., Queralt-Rosinach, N., Bravo, A., Deu-Pons, J., Bauer-Mehren, A., Baron, M., et al. (2015). DisGeNET: a discovery platform for the dynamical exploration of human diseases and their genes. Database (Oxford) 2015:bav028. doi: 10.1093/database/bav028
Reindl, M., Knipping, G., Wicher, I., Dilitz, E., Egg, R., Deisenhammer, F., et al. (2001). Increased intrathecal production of apolipoprotein D in multiple sclerosis. J. Neuroimmunol. 119, 327–332. doi: 10.1016/s0165-5728(01)00378-2
Rivera, A. D., Chacon-De-La-Rocha, I., Pieropan, F., Papanikolau, M., Azim, K., and Butt, A. M. (2021a). Keeping the ageing brain wired: a role for purine signalling in regulating cellular metabolism in oligodendrocyte progenitors. Pflügers Arch. 473, 775–783. doi: 10.1007/s00424-021-02544-z
Rivera, A. D., Pieropan, F., Chacon-De-La-Rocha, I., Lecca, D., Abbracchio, M. P., Azim, K., et al. (2021b). Functional genomic analyses highlight a shift in Gpr17-regulated cellular processes in oligodendrocyte progenitor cells and underlying myelin dysregulation in the aged mouse cerebrum. Aging Cell 20:e13335. doi: 10.1111/acel.13335
Rivera, A. D., Pieropan, F., Williams, G., Calzolari, F., Butt, A. M., and Azim, K. (2022a). Drug connectivity mapping and functional analysis reveal therapeutic small molecules that differentially modulate myelination. Biomed. Pharmacother. 145:112436. doi: 10.1016/j.biopha.2021.112436
Rivera, A. D., Butt, A. M., and Azim, K. (2022b). Resolving the age-related decline in central nervous system myelin turnover and drug discovery for oligodendroglial rejuvenation. Neural Regen. Res. in press.
Rivera, A., Vanzuli, I., Arellano, J. J., and Butt, A. (2016). Decreased regenerative capacity of oligodendrocyte progenitor cells (NG2-Glia) in the ageing brain: a vicious cycle of synaptic dysfunction, myelin loss and neuronal disruption? Curr. Alzheimer Res. 13, 413–418. doi: 10.2174/1567205013666151116125518
Roy, K., Murtie, J. C., El-Khodor, B. F., Edgar, N. S., Sardi, P., Hooks, B. M., et al. (2007). Loss of erbB signaling in oligodendrocytes alters myelin and dopaminergic function, a potential mechanism for neuropsychiatric disorders. Proc. Natl. Acad. Sci. U S A 104, 8131–8136. doi: 10.1073/pnas.0702157104
Rübsam, M., Mertz, A. F., Kubo, A., Marg, S., Jüngst, C., Goranci-Buzhala, G., et al. (2017). E-cadherin integrates mechanotransduction and EGFR signaling to control junctional tissue polarization and tight junction positioning. Nat. Commun. 8:1250. doi: 10.1038/s41467-017-01170-7
Sanz-Rodriguez, M., Gruart, A., Escudero-Ramirez, J., de Castro, F., Delgado-García, J. M., Wandosell, F., et al. (2018). R-Ras1 and R-Ras2 are essential for oligodendrocyte differentiation and survival for correct myelination in the central nervous system. J. Neurosci. 38, 5096–5110. doi: 10.1523/JNEUROSCI.3364-17.2018
Scafidi, J., Hammond, T. R., Scafidi, S., Ritter, J., Jablonska, B., Roncal, M., et al. (2014). Intranasal epidermal growth factor treatment rescues neonatal brain injury. Nature 506, 230–234. doi: 10.1038/nature12880
Schmucker, J., Ader, M., Brockschnieder, D., Brodarac, A., Bartsch, U., and Riethmacher, D. (2003). erbB3 is dispensable for oligodendrocyte development in vitro and in vivo. Glia 44, 67–75. doi: 10.1002/glia.10275
Segel, M., Neumann, B., Hill, M. F. E., Weber, I. P., Viscomi, C., Zhao, C., et al. (2019). Niche stiffness underlies the ageing of central nervous system progenitor cells. Nature 573, 130–134. doi: 10.1038/s41586-019-1484-9
Sexton, C. E., Walhovd, K. B., Storsve, A. B., Tamnes, C. K., Westlye, L. T., Johansen-Berg, H., et al. (2014). Accelerated changes in white matter microstructure during aging: a longitudinal diffusion tensor imaging study. J. Neurosci. 34, 15425–15436. doi: 10.1523/JNEUROSCI.0203-14.2014
Singh, B., Carpenter, G., and Coffey, R. J. (2016). EGF receptor ligands: recent advances. F1000Res. 5(F1000 Faculty Rev):2270. doi: 10.12688/f1000research.9025.1
Slater, D. A., Melie-Garcia, L., Preisig, M., Kherif, F., Lutti, A., and Draganski, B. (2019). Evolution of white matter tract microstructure across the life span. Hum. Brain Mapp. 40, 2252–2268. doi: 10.1002/hbm.24522
Smith, K. J. (2006). Axonal protection in multiple sclerosis—a particular need during remyelination?. Brain 129, 3147–3149. doi: 10.1093/brain/awl323
Soreq, L., Rose, J., Soreq, E., Hardy, J., Trabzuni, D., Cookson, M. R., et al. (2017). Major shifts in glial regional identity are a transcriptional hallmark of human brain aging. Cell Rep. 18, 557–570. doi: 10.1016/j.celrep.2016.12.011
Steadman, P. E., Xia, F., Ahmed, M., Mocle, A. J., Penning, A. R. A., Geraghty, A. C., et al. (2020). Disruption of oligodendrogenesis impairs memory consolidation in adult mice. Neuron 105, 150–164.e6. doi: 10.1016/j.neuron.2019.10.013
Tanaka, J., and Sobue, K. (1994). Localization and characterization of gelsolin in nervous tissues: gelsolin is specifically enriched in myelin-forming cells. J. Neurosci. 14, 1038–1052. doi: 10.1523/JNEUROSCI.14-03-01038.1994
Tiwari-Woodruff, S. K., Buznikov, A. G., Vu, T. Q., Micevych, P. E., Chen, K., Kornblum, H. I., et al. (2001). OSP/claudin-11 forms a complex with a novel member of the tetraspanin super family and beta1 integrin and regulates proliferation and migration of oligodendrocytes. J. Cell Biol. 153, 295–305. doi: 10.1083/jcb.153.2.295
Tschumperlin, D. J. (2004). EGFR autocrine signaling in a compliant interstitial space: mechanotransduction from the outside in. Cell Cycle 3, 996–997. doi: 10.4161/cc.3.8.1062
Wang, F., Ren, S. Y., Chen, J. F., Liu, K., Li, R. X., Li, Z. F., et al. (2020). Myelin degeneration and diminished myelin renewal contribute to age-related deficits in memory. Nat. Neurosci. 23, 481–486. doi: 10.1038/s41593-020-0588-8
Werner, M. H., Nanney, L. B., Stoscheck, C. M., and King, L. E. (1988). Localization of immunoreactive epidermal growth factor receptors in human nervous system. J. Histochem. Cytochem. 36, 81–86. doi: 10.1177/36.1.3275713
Willis, C. M., Nicaise, A. M., Bongarzone, E. R., Givogri, M., Reiter, C. R., Heintz, O., et al. (2020). Astrocyte support for oligodendrocyte differentiation can be conveyed via extracellular vesicles but diminishes with age. Sci. Rep. 10:828. doi: 10.1038/s41598-020-57663-x
Xi, Y., Ju, R., and Wang, Y. (2020). Roles of annexin A protein family in autophagy regulation and therapy. Biomed. Pharmacother. 130:110591. doi: 10.1016/j.biopha.2020.110591
Yang, J., Cheng, X., Qi, J., Xie, B., Zhao, X., Zheng, K., et al. (2017). EGF enhances oligodendrogenesis from glial progenitor cells. Front. Mol. Neurosci. 10:106. doi: 10.3389/fnmol.2017.00106
Yarden, Y., and Sliwkowski, M. X. (2001). Untangling the ErbB signalling network. Nat. Rev. Mol. Cell Biol. 2, 127–137. doi: 10.1038/35052073
Zhang, Y., Chen, K., Sloan, S. A., Bennett, M. L., Scholze, A. R., OKeeffe, S., et al. (2014). An RNA-sequencing transcriptome and splicing database of glia, neurons and vascular cells of the cerebral cortex. J. Neurosci. 34, 11929–11947. doi: 10.1523/JNEUROSCI.1860-14.2014
Zhu, Y., Park, J., Hu, X., Zheng, K., Li, H., Cao, Q., et al. (2010). Control of oligodendrocyte generation and proliferation by Shp2 protein tyrosine phosphatase. Glia 58, 1407–1414. doi: 10.1002/glia.21016
Keywords: EGF, EGFR, ErbB, oligodendrocyte, myelin, aging, white matter
Citation: Rivera AD, Azim K, Macchi V, Porzionato A, Butt AM and De Caro R (2022) Epidermal Growth Factor Pathway in the Age-Related Decline of Oligodendrocyte Regeneration. Front. Cell. Neurosci. 16:838007. doi: 10.3389/fncel.2022.838007
Received: 17 December 2021; Accepted: 23 February 2022;
Published: 17 March 2022.
Edited by:
Jason R. Plemel, University of Alberta, CanadaCopyright © 2022 Rivera, Azim, Macchi, Porzionato, Butt and De Caro. This is an open-access article distributed under the terms of the Creative Commons Attribution License (CC BY). The use, distribution or reproduction in other forums is permitted, provided the original author(s) and the copyright owner(s) are credited and that the original publication in this journal is cited, in accordance with accepted academic practice. No use, distribution or reproduction is permitted which does not comply with these terms.
*Correspondence: Andrea D. Rivera, andrea.rivera@unipd.it
† These authors have contributed equally to this work