Hizikia fusiforme functional oil (HFFO) prevents neuroinflammation and memory deficits evoked by lipopolysaccharide/aluminum trichloride in zebrafish
- 1Guangdong Provincial Key Laboratory of Aquatic Product Processing and Safety, Guangdong Provincial Engineering Laboratory for Marine Biological Products, Guangdong Provincial Engineering Technology Research Center of Seafood, Key Laboratory of Advanced Processing of Aquatic Product of Guangdong Higher Education Institution, Shenzhen Institute of Guangdong Ocean University, Zhanjiang Municipal Key Laboratory of Marine Drugs and Nutrition for Brain Health, Research Institute for Marine Drugs and Nutrition, College of Food Science and Technology, Guangdong Ocean University, Zhanjian, China
- 2Collaborative Innovation Center of Seafood Deep Processing, Dalian Polytechnic University, Dalian, China
Background: Oxidative stress, cholinergic deficiency, and neuroinflammation are hallmarks of most neurodegenerative disorders (NDs). Lipids play an important role in brain development and proper functioning. Marine-derived lipids have shown good memory-improving potentials, especially those from fish and microalgae. The cultivated macroalga Hizikia fusiforme is healthy food and shows benefits to memory, but the study is rare on the brain healthy value of its oil. Previously, we had reported that the Hizikia fusiforme functional oil (HFFO) contains arachidonic acid, 11,14,17-eicosatrienoic acid, phytol, and other molecules displaying in vitro acetylcholinesterase inhibitory and nitroxide scavenging activity; however, the in vivo effect remains unclear. In this study, we further investigated its potential effects against lipopolysaccharides (LPS)- or aluminum trichloride (AlCl3)-induced memory deficiency in zebrafish and its drug-related properties in silica.
Methods: We established memory deficit models in zebrafish by intraperitoneal (i.p.) injection of lipopolysaccharides (LPS) (75 ng) or aluminum trichloride (AlCl3) (21 μg), and assessed their behaviors in the T-maze test. The interleukin-1β (IL-1β), tumor necrosis factor-α (TNF-α), acetylcholine (ACh), and malondialdehyde (MDA) levels were measured 24 h after the LPS/AlCl3 injection as markers of inflammation, cholinergic activity, and oxidative stress. Furthermore, the interaction of two main components, 11,14,17-eicosatrienoic acid and phytol, was investigated by molecular docking, with the important anti-inflammatory targets nuclear factor kappa B (NF-κB) and cyclooxygenase 2 (COX-2). Specifically, the absorption, distribution, metabolism, excretion, and toxicity (ADMET) and drug-likeness properties of HFFO were studied by ADMETlab.
Results: The results showed that HFFO reduced cognitive deficits in zebrafish T-maze induced by LPS/AlCl3. While the LPS/AlCl3 treatment increased MDA content, lowered ACh levels in the zebrafish brain, and elevated levels of central and peripheral proinflammatory cytokines, these effects were reversed by 100 mg/kg HFFO except for MDA. Moreover, 11,14,17-eicosatrienoic acid and phytol showed a good affinity with NF-κB, COX-2, and HFFO exhibited acceptable drug-likeness and ADMET profiles in general.
Conclusion: Collectively, this study's findings suggest HFFO as a potent neuroprotectant, potentially valuable for the prevention of memory impairment caused by cholinergic deficiency and neuroinflammation.
Introduction
Neurodegenerative disorders (NDs) are chronic, progressive, and severely debilitating neurological illnesses caused by the loss of neurons in the brain and spinal cord (Whitehouse et al., 1982). With the aging of the population and the deterioration of the environment, the threat of NDs to human beings is becoming increasingly serious, among which the most representative is Alzheimer's disease (AD). Existing studies have shown that NDs are closely related to neurodegeneration and neuronal damage caused by neuroinflammation in the brain (Uttara et al., 2009; Kumar et al., 2016; Ransohoff, 2016). However, current clinical drugs for the prevention and treatment of NDs have limited efficacy, such as donepezil, which can only partially relieve symptoms but not reverse or prevent their development (Bachurin et al., 2017). Thus, daily nutritional interventions such as healthy lipid intake are increasingly viewed as important supplementary approaches to drugs for these chronic neurodegenerative diseases in a specific population (Petersson and Philippou, 2016; McGrattan et al., 2019; Livingston et al., 2020).
Marine organisms live in a water environment with low temperatures, featuring them with a rich level of polyunsaturated fatty acids (PUFAs) synthesized by themselves or accumulated from their food chains. Many PUFAs possess anti-neuroinflammatory effects and show potential for preventing or treating NDs. For example, docosahexaenoic acid (DHA) or its metabolites can inhibit acetylcholinesterase (AChE), promote neuronal survival/growth and synaptogenesis, increase cortical brain-derived neurotrophic factor (BDNTF), inhibit oxidative-stress-induced caspase-3 activation and IL-1-stimulated expression of COX-2, and protect liposaccharide (LPS)-induced mouse model of acute neuroinflammation (Barbosa et al., 2014). Eicosapentaenoic acid (EPA) ethyl ester was reported to lower interleukin-1β (IL-1β) and increase peroxisome proliferator-activated receptor (PPAR)γ in Aβ i.c.v. aged mice and EPA was also found to be the active principle of a microalgae Nannochloropsis oceanica, demonstrating anti-inflammatory, antioxidative, and anti-amyloidogenesis activities in a mouse model of LPS-induced AD (Trépanier et al., 2016; Choi et al., 2017). Currently, the main commercial sources for marine PUFAs are fish and microalgae; however, macroalgae should not be neglected considering their high proportion of PUFAs in their total lipids and neuroprotective isoprenoids like fucoxanthin coexisting in lipids (Barbalace et al., 2019; Catanesi et al., 2021).
Brown alga Hizikia fusiforme (also named “Hijiki” or Sargassum fusiforme), belonging to the class Phaeophyceae, order Fucales, and family Sargassaceae (Meinita et al., 2021), is a kind of natural healthy food that can be used as both medicine and food. Its medicinal effects are clearly recorded in traditional Chinese medicinal works, including Shennong Materia Medica Classic (dated 200 AD) and Compendium of Materia Medica (published in the late 16th century). As a delicious food, it is now popular in China, Korea, Japan, the United Kingdom, and North America and has been effectively cultivated in China and Korea (Liu et al., 2020; Meinita et al., 2021). In recent years, it has attracted more attention from natural medicine research (Liu et al., 2020; Meinita et al., 2021). Researchers have found a lot of active ingredients in it that have the potential to improve learning and memory (Hu et al., 2016; Bogie et al., 2019). Sargassum fusiforme polysaccharides (SFPS) can inhibit the apoptosis of central nerve cells, increase the activities of total antioxidant capacity (T-AOC) and superoxide dismutase (SOD) in serum, and reduce the content of malonaldehyde (MDA), thus improving the serum antioxidant ability of mice and enhancing the learning and memory ability of mice (Liu et al., 2018). In addition, SFPS significantly inhibited the production of TNF-α and other inflammatory molecules, and it can significantly reduce reactive oxygen species (ROS), cell death, and NO levels in a dose-dependent manner in LPS-induced zebrafish (Wang et al., 2021). Sargassum fusiforme polyphenols (SFP) can reduce brain damage and delay the occurrence of AD mainly through their antioxidant effect (Hu et al., 2016). In addition, the Hizikia fusiforme fucoxanthin showed strong scavenging ability on 1, 1-diphenyl-2- picrylhydrazyl (DPPH), OH−, O2, and H2O2 (Kuang and Zhang, 2014). But the study is rare on the brain-health value of its oil. Previously, we reported that the Hizikia fusiforme functional oil (HFFO) contained arachidonic acid (ARA, one of the two main PUFAs in the brain) (20.54%), 11,14,17-eicosatrienoic acid (ETrA) (19.53%), phytol (6.55%) (Bazinet and Layé, 2014), and other molecules displayed in vitro acetylcholinesterase inhibitory and nitroxide scavenging activity (Yang et al., 2020). However, the in vivo effect remains unclear.
Bacterial LPS or AlCl3 can cause AD-like pathology, aggravating neuroinflammation, oxidative stress, and acetylcholinesterase (AChE) activity in the brain of rodents (Tyagi et al., 2010; Mathiyazahan et al., 2015; Ahmad Rather et al., 2019; Huat et al., 2019). They also affect adult zebrafish via similar mechanisms or cause-related responses (Senger et al., 2011; Gonçalves et al., 2012). Here, we develop LPS/AlCl3-induced neuroinflammatory zebrafish model to assess the potential effects of HFFO on memory and cognitive impairment in vivo and its drug-related properties in silico. The putative neuroprotective activity of HFFO in zebrafish was also probed by evaluating MDA and ACh levels in the brains and by analyzing the levels of the IL-1β and TNF-α in both brain and peripheral tissues.
Materials and methods
Animals
Wild-type AB zebrafish of 6–8 months old (adult, 1:1 male to female) were purchased from Shanghai Jianyu Aquarium (Shanghai, China) and acclimatized for at least 2 weeks in a 50-L tank in the aquatic facility of Guangdong Ocean University Shenzhen Research Institute. The fish were raised according to standard conditions (the illumination condition is a 14-h light/10-h dark cycle and the ambient temperature is 25 ± 2°C) (Westerfield, 2007) and fed with Artemis larvae at 9 am and 2 pm every day.
Chemicals
Hizikia fusiforme essential oil (HFFO) was prepared by our research group (Yang et al., 2020). The main ingredients of HFFO are arachidonic acid, 11,14,17-eicosatrienoic acid, palmitic acid, and phytol. The BCA protein, Ach and MDA assay kits, fish IL-1β enzyme-linked immunosorbent assay (ELISA) kit, and fish TNF-α ELISA kit were purchased from Nanjing Jiancheng Bioengineering Institute (Nanjing, China). Eugenol was purchased from Huaxia Reagent (Chengdu, China) and LPS from Shanghai Ryon Biological Technology Co. Ltd. (Shanghai, China). All the chemicals involved in this study are analytical grade.
Animal model development and T-maze behavioral testing
To perform the experiments based on the LPS model, 50 zebrafish (3.0 ± 0.4 cm in length) were randomly divided into a control group, an LPS model, and three treatment groups (n = 10 per group). The treatment groups received 50, 100, or 200 mg/kg HFFO with food containing HFFO for 2 weeks. The control and LPS groups were fed equal amounts of normal food. After 2 weeks, the LPS and HFFO groups were anesthetized and injected intraperitoneally (i.p.) with LPS solution (0.015 mg/ml, 5 μl) using a 10-μl gas phase injection needle 0.5 mm in the outer diameter. The control group was injected with the same amount of saline. Memory testing was performed in the T-maze 24 h later. Before the injection of LPS/saline, the fish were previously trained in the T-maze for 4 days to record their latency of entering the enriched chamber (EC) zones for the first time.
The experiments on the AlCl3 model were developed similar to the LPS model and involved a 20-day feeding with normal or HFFO-containing food, followed by an i.p. injection of the AlCl3 solution (4.2 mg/ml, 5 μl, pH = 5.0 ± 0.2). The control group was injected with the same amount of saline and tested in the T-maze 24 h later.
In this study, we performed a zebrafish cognitive test using an aquatic T-maze (Tyagi et al., 2010). Apowersoft (Apowersoft Co. Ltd., Hong Kong, China) was used to record videos from Microsoft LifeCam Studio 1080p HD cameras. Offline video analyses were performed by the Supersys software (Xinruan, Shanghai, China), assessing the latency of zebrafish entering the enriched chamber (EC) zone for the first time.
Molecular biomarker assays
After 24 h of behavioral testing, the fish were euthanized to prepare the brain and peripheral tissue homogenates (Nie et al., 2022). Zebrafish brain sample supernatants were used to estimate tissue biomarker changes, i.e., Ach and MDA levels. In addition, Il-1 β and TNF-α levels in the supernatant of the brain and peripheral tissues of zebrafish were determined according to the ELISA kits' instructions (Rishitha and Muthuraman, 2018).
Statistical analysis
Statistical analysis was performed by one-way analysis of variance (ANOVA, factor: group) followed by a post hoc Dunnett for significant AVOVA data. The results are expressed as the mean ± SD. P-value was set at <0.05 for all tests.
Molecular docking analysis
This study employed AutoDock Vina (Trott and Olson, 2010) for the in silico protein–ligand docking analysis. Protein three-dimensional (3D) structures (PDB format) of NF-κB (PDB: 1VKX) and COX-2 (PDB: 5IKT) were retrieved from Protein Databank (PDB) (http://www.rcsb.org). Chemical 3D conformer of the compounds (phytol and eicosatrienoic acid) was obtained from the PubChem database (http://www.ncbi.nlm.nih.gov/pccompound). Molecular docking simulations were performed using AutoDock Vina using default parameters. The protein–ligand interactions were investigated in the PyMOL (https://www.PyMOL.org).
In silico prediction of ADMET and drug-likeness properties of HFFO
We used ADMETlab 2.0 (https://admetmesh.scbdd.com/pub/) to assess HFFO pharmacokinetic characteristics and toxicity; the online platform can help researchers predict the absorption, distribution, metabolism, excretion, and toxicity (ADMET) and drug-likeness properties of compounds (Guo et al., 2021).
Results
HFFO improved LPS/AlCl3-induced memory impairment
Overall, zebrafish treated with LPS/AlCl3 showed impaired spatial memory in the T-maze test; there were significant treatment effects for zebrafish cognitive performance by HFFO treatment (F(4, 45) = 141.9, P < 0.001 in Figure 1A; F(4, 45) = 80.63, P < 0.001 in Figure 1B).
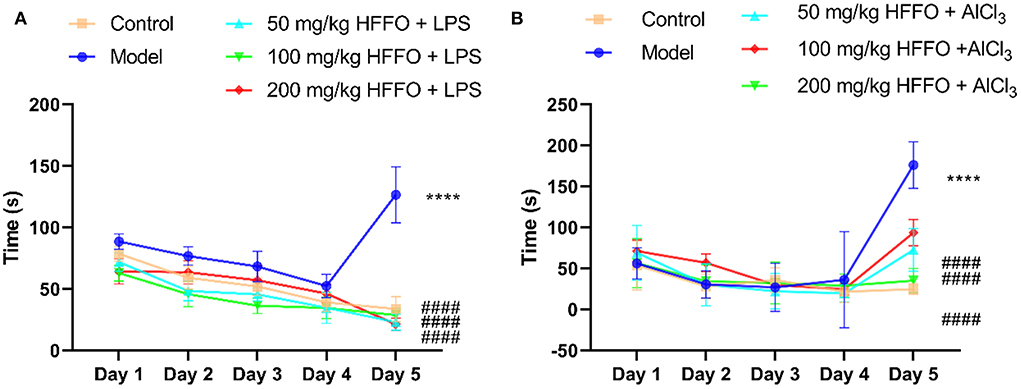
Figure 1. The latency (s) of first entry into the enriched chamber (EC) zone of the T-maze test. LPS model (A), AlCl3 model (B). n = 10, *p < 0.05, **p < 0.01, ***p < 0.005, ****p < 0.001, vs. the control group; #p < 0.05, ##p < 0.01, ###p < 0.005, ####p < 0.001, vs. the model group.
Subsequent post hoc testing revealed that the latency of first entry into the EC zone increased for the model groups. The swimming tracks also clearly showed the reduced preference of the model group fish to the EC zone (Figure 2) following the LPS/AlCl3 injections. In contrast, pretreatment with low to high doses of HFFO prevented these effects of LPS/AlCl3.
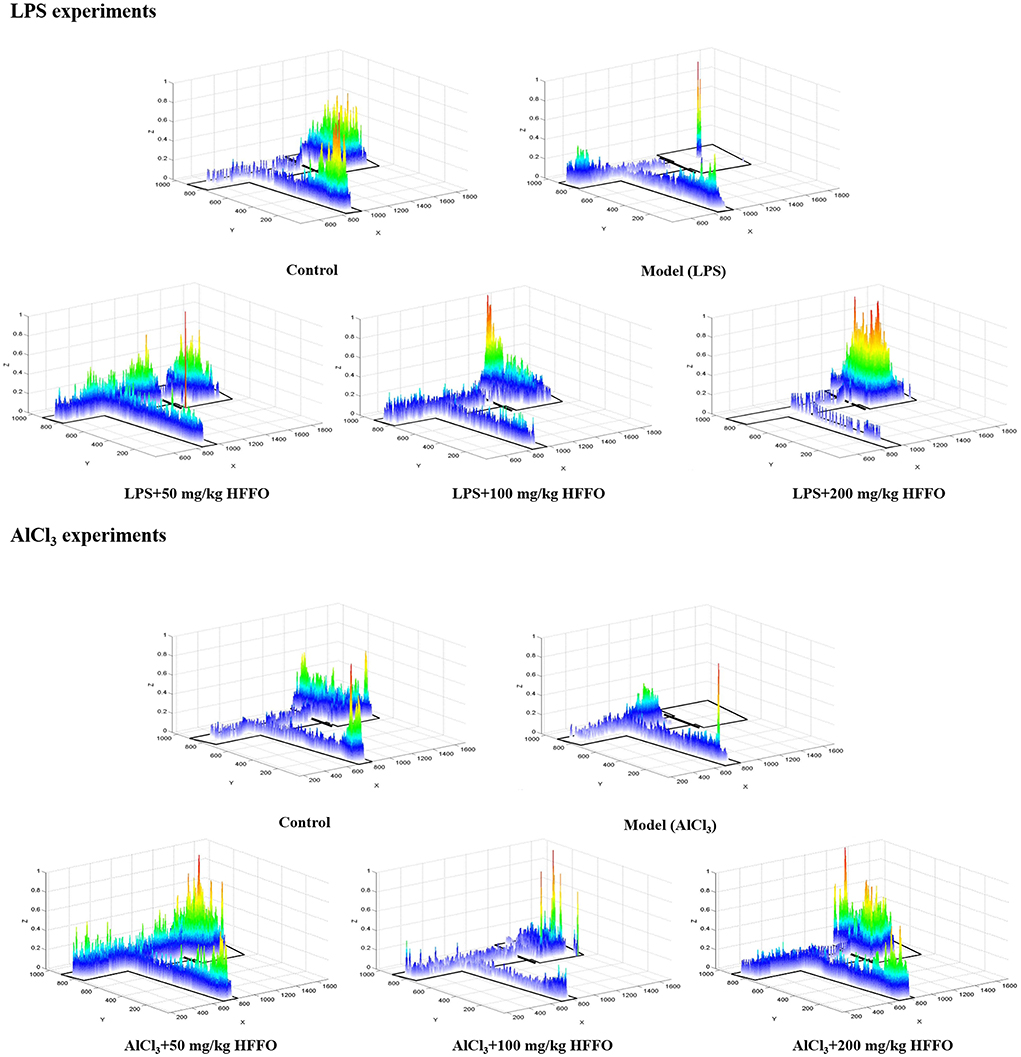
Figure 2. Heatmaps of zebrafish activity in the T-maze on the fifth day. The X-axis and Y-axis in the figure represent the motion trajectory of zebrafish, while the Z-axis represents the residence time of zebrafish. The higher the Z-axis, the longer the residence time of zebrafish at a certain point.
Effect of HFFO against LPS/AlCl3 on proinflammatory cytokines
In addition to overt behavioral effects, the treatment with LPS/AlCl3 promoted the release of inflammatory cytokine IL-1β from the brain and peripheral tissues (Figures 3A–D), and HFFO supplementation reduced LPS-induced IL-1β significantly at all and part of the doses in the brain and peripheral tissues, respectively (F(4, 10) = 32.93, P < 0.001 in Figure 3A; F(4, 10) = 7.160, P < 0.01 in Figure 3C). As for AlCl3-caused IL-1β increase, HFFO showed a reduction in peripheral tissue at the high dose while no inhibition in the brain at the tested doses (F(4, 10) = 15.69, P < 0.001 in Figure 3B; F(4, 10) = 19.28, P < 0.001 in Figure 3D).
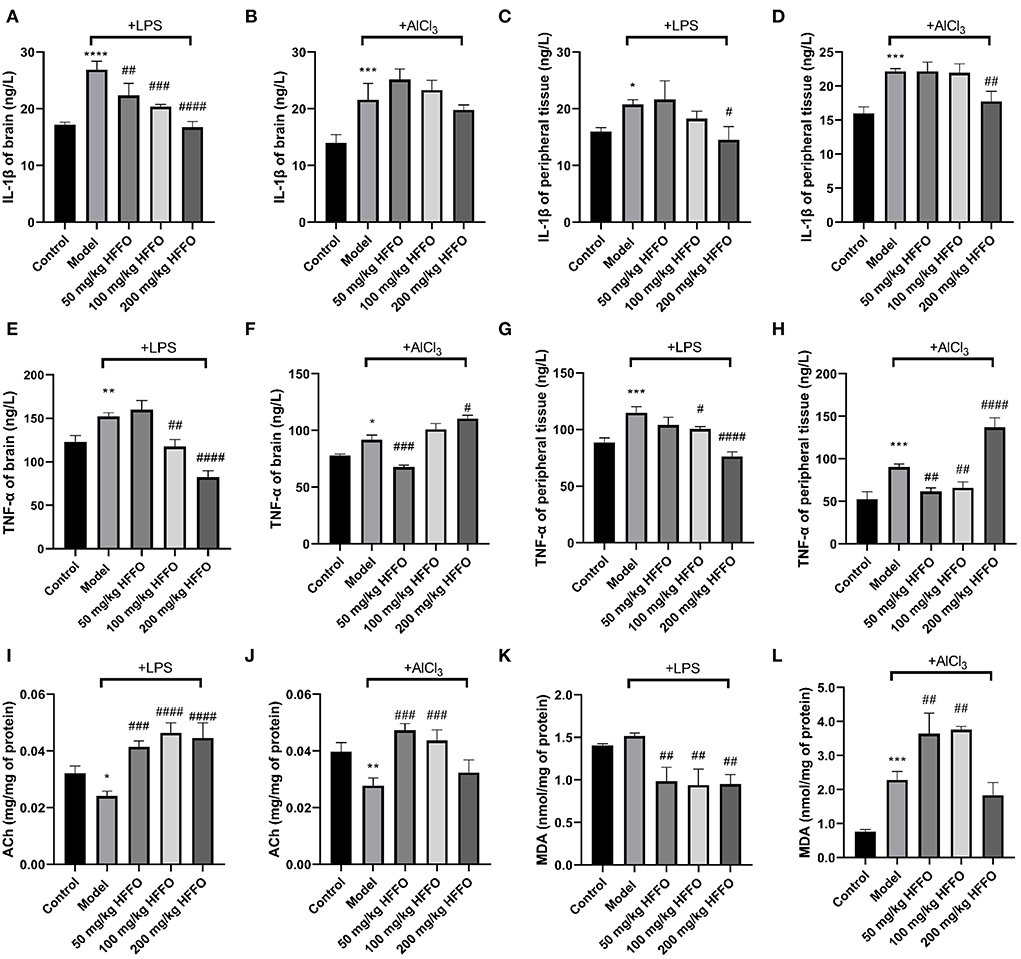
Figure 3. IL-1β (A–D) and TNF-α (E–H) content in zebrafish brain and peripheral tissue, and ACh (I,J) and MDA (K,L) levels in zebrafish brain tissue. n = 3, *p < 0.05, **p < 0.01, ***p < 0.005, ****p < 0.001, vs. the control group; #p < 0.05, ##p < 0.01, ###p < 0.005, ####p < 0.001, vs. the model group.
Besides, the treatment with LPS/AlCl3 also promoted the release of TNF-α from brain and peripheral tissues, and HFFO supplementation inhibited these effects at part of the doses (F(4, 10) = 46.81, P < 0.001 in Figure 3E; F(4, 10) = 84.67, P < 0.001 in Figure 3F; F(4, 10) = 30.44, P < 0.001 in Figure 3G; F(4, 10) = 62.92, P < 0.001 in Figure 3H).
Effect of HFFO against LPS/AlCl3 on ACh and MDA
Furthermore, paralleling their cognitive deficits in the T-maze, zebrafish treated with LPS/AlCl3 decreased brain ACh content (i.e., exhibited higher brain AChE activity), whereas almost all the doses of HFFO significantly reversed this phenomenon (F (4, 10) = 23.93, P < 0.001 in Figure 3I; F (4, 10) = 17.16, P < 0.001 in Figure 3J). This is consistent with the fact that excessive AChE activity is closely related to memory deficits (Giacobini et al., 2002).
Because oxidative stress plays a key role in neuronal injury and apoptosis (Mcbean et al., 2016), the levels of MDA (a lipid peroxidation degradation indicator) were also analyzed to assess the oxidant–antioxidant balance in the zebrafish brain, to parallel their behavioral analyses. In the LPS model, HFFO significantly reduced MDA levels in the brain of zebrafish. However, in the AlCl3 model, low and medium doses of HFFO significantly increased MDA levels (F (4, 10) = 15.40, P < 0.001 in Figure 3K; F (4, 10) = 42.12, P < 0.001 in Figure 3L).
Molecular docking and interaction analysis
Previous studies have suggested that phytol and eicosatrienoic acid may exert anti-inflammatory effects by interacting with nuclear factor kappa B (NF-κB) (Tak and Firestein, 2001; Silva et al., 2014; Chen et al., 2015) and cyclooxygenase 2 (COX-2) (Islam et al., 2020; Baker et al., 2021). In our study, eicosatrienoic acid and phytol are two major components of the HFFO. To explore the potential interactions and gain insights into the mechanism of phytol with NF-κB and COX-2, and eicosatrienoic acid with NF-κB and COX-2, molecular docking studies were conducted. Phytol displayed considerable binding affinity to NF-κB and COX-2 at −6.1 and −7.2 kcal/mol (Table 1), respectively. The interaction forces between phytol and potential targets (NF-κB and COX-2) mainly include polar (hydrogen bonds) interactions and non-polar interactions (Figures 4A,B). Specifically, phytol forms a hydrogen bond with NF-κB at Ile439 (length of 2.4 Å) and non-polar interactions with NF-κB at Tyr357, Val412, Val358, etc. (Figure 4A). Phytol forms a hydrogen bond with COX-2 at Ser353 (length of 2.1 Å) and non-polar interactions with COX-2 at Tyr355, Gly354, His90, etc. (Figure 4B). In terms of eicosatrienoic acid, it also showed considerable binding affinity to NF-κB and COX-2 at −6.1 and −6.8 kcal/mol (Table 1), respectively. The interaction forces were similar to those of the phytol with NF-κB and COX-2 (Figures 4C,flreffig4D). In summary, these results show that phytol and eicosatrienoic acid bind to the analyzed targets with high affinity and form clearly defined specific interactions with them.
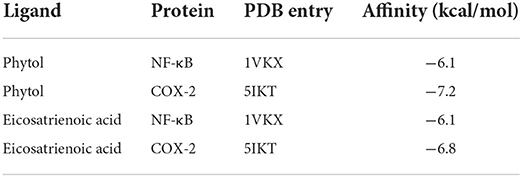
Table 1. Molecular docking affinity (kcal/mol) of phytol and eicosatrienoic acid with NF-κB and COX-2, respectively.
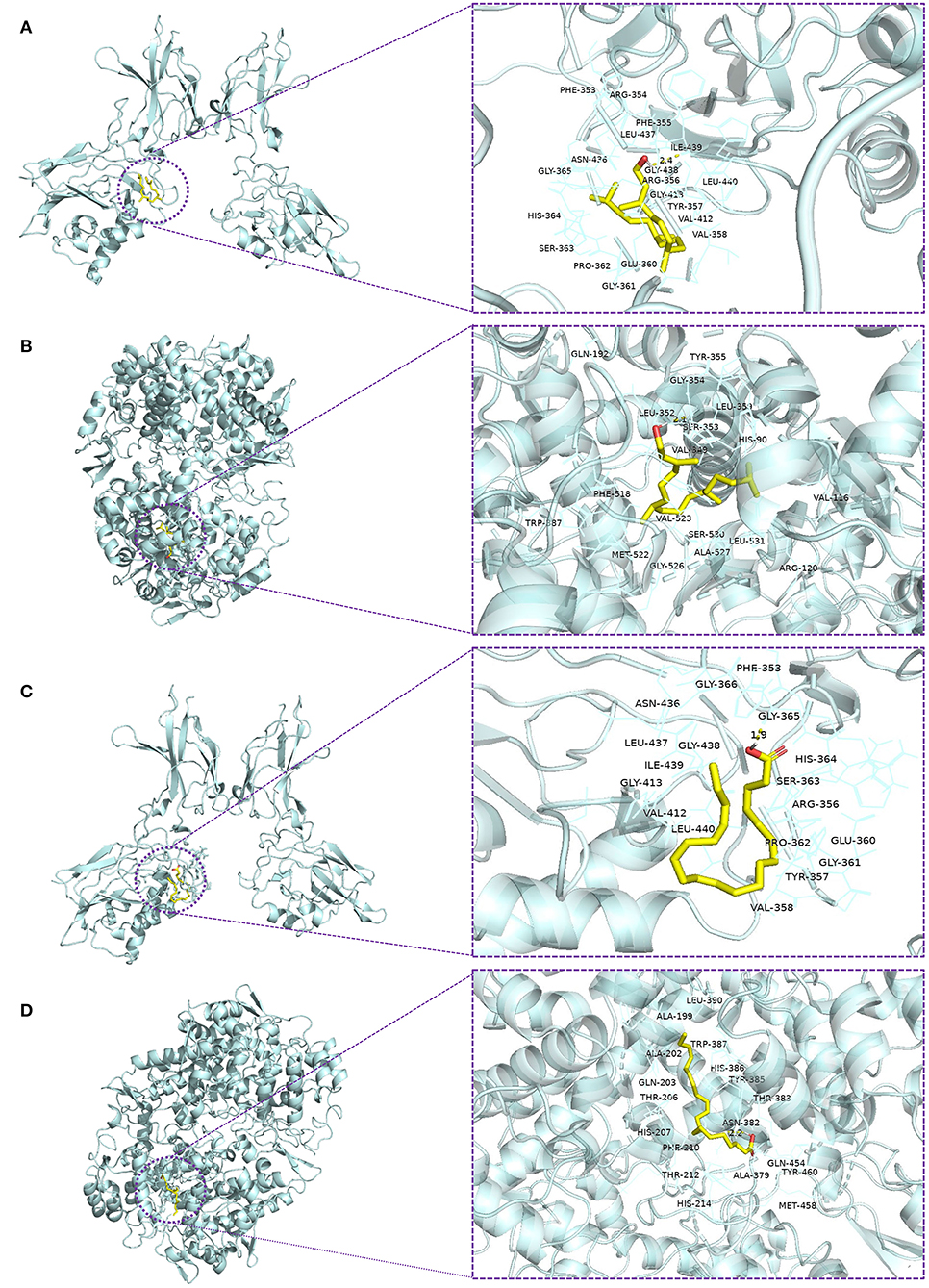
Figure 4. Molecular docking of ligands with their potential targets. Docking interaction 3D view of phytol with NF-κB (A) and COX-2 (B), and eicosatrienoic acid with NF-κB (C) and COX-2 (D). Residues are within the distance of 4Å, and the yellow dotted lines indicate hydrogen bonds.
Prediction of ADMET and drug-likeness properties
To investigate the pharmacokinetic profile of HFFO and its potential as a drug, we used ADMETlab 2.0 (Jiang et al., 2020; Guo et al., 2021) to predict its ADMET (absorption, distribution, metabolism, elimination, and toxicity) and drug-likeness properties. The corresponding results are presented in Table 2. Generally, HFFO possesses acceptable ADMET and drug-likeness properties as oral drugs. Its main components, including arachidonic acid, eicosatrienoic acid, palmitic acid, tetradecanoic acid, and 9-hexadecenoic, were endowed with promising activities in MDCK permeability, human intestinal absorption (HIA), and blood–brain barrier (BBB) penetration. The compounds also exhibited acceptable safety profiles, generally performing well on most metrics (e.g., low hERG blockers (index of heart toxicity), human hepatotoxicity (H-HT), and rat oral acute toxicity), and these components fulfilled one or more of the drug-likeness criteria, such as the Lipinski rule (Lipinski et al., 2001), Pfizer rule (Hughes et al., 2008), and golden triangle (Johnson et al., 2009). In addition, the physical properties of the main HFFO ingredients are within an acceptable range (Supplementary Tables S1–S6). In summary, the predicted ADMET properties of the main components within HFFO indicate that they are promising candidates as lead compounds.
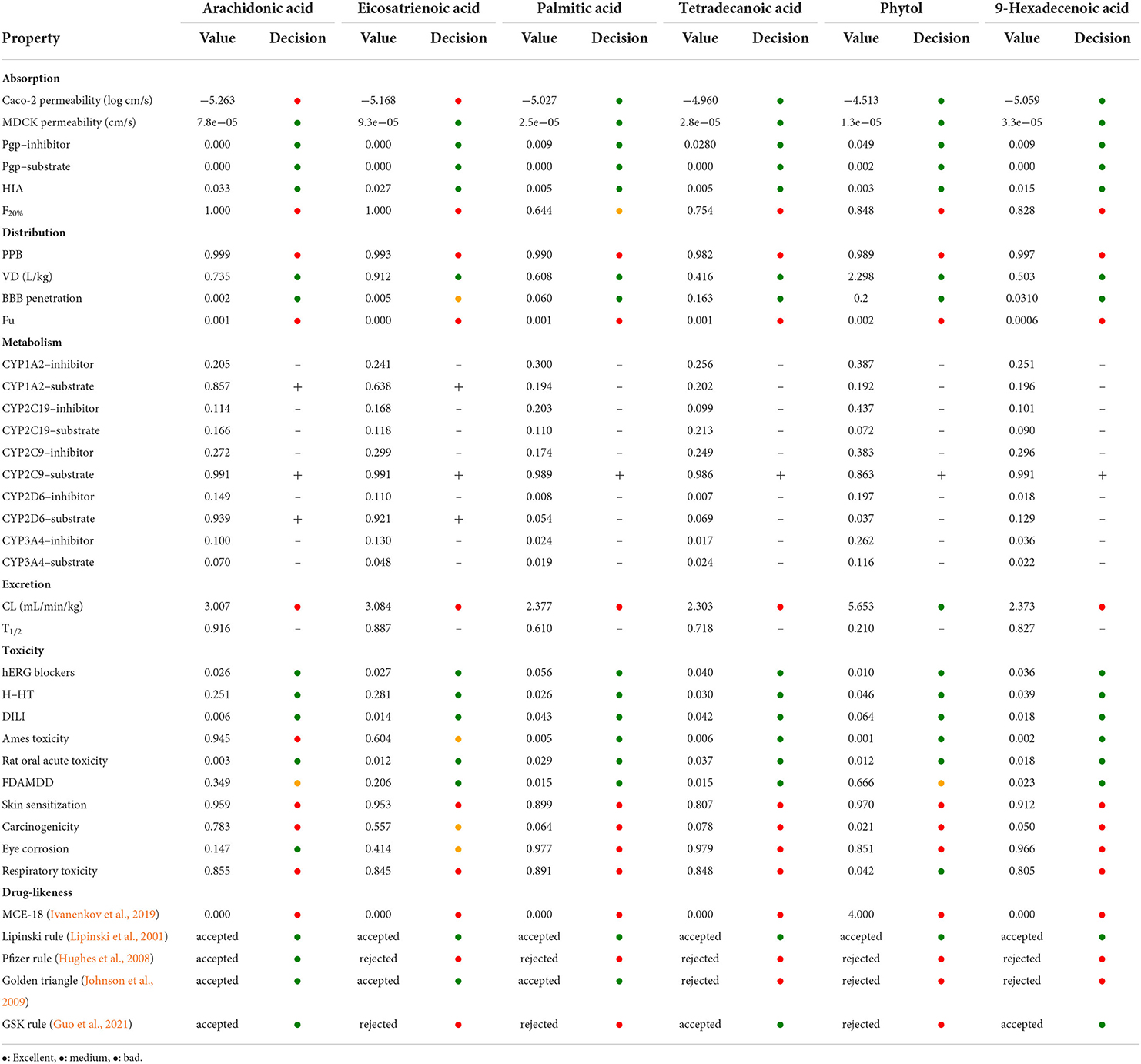
Table 2. ADMET and drug-likeness properties of HFFO main ingredient through online prediction tool of ADMETlab 2.0.
Discussion
Mounting evidence shows that peripheral administration of LPS in mice can activate astrocytes and microglia; increase the expression of COX-2, inducible nitric oxide synthase (i-NOS), and proinflammatory cytokines; promote the intracellular accumulation of amyloid precursor protein, amyloid β (Aβ) protein, and hyperphosphorylated tau; and finally exacerbate memory deficits (Catorce and Gevorkian, 2016). In addition, LPS potently induces oxidative stress in the rodent brain and impairs memory cognition (Cunningham and Sanderson, 2008; Tyagi et al., 2008; Czerniawski and Guzowski, 2014; Ming et al., 2015). In addition, LPS administration has also been linked to enhanced brain AChE activity following an intracerebroventricular or systemic administration of LPS in rodents (Sebai et al., 2009; Han et al., 2019).
Likewise, subchronic exposure of zebrafish to AlCl3 or feeding of AlCl3 in mice enhances brain AChE activity (Zatta et al., 2002; Senger et al., 2011; Liu et al., 2013). Intriguingly, activated AChE can deteriorate Aβ aggregation, decrease brain-derived neurotrophic factor (BDNF) expression (Auti and Kulkarni, 2019), and further promote oxidative stress and neuroinflammation through a ‘cholinergic anti-inflammatory pathway’ (CAIP) in which α7 nicotinic acetylcholine (ACh) receptor acts as an effector player (Tabet, 2006; Benfante et al., 2021). Another study also reported that 10–20 days of chronic exposure of adult zebrafish to aluminum leads to brain oxidative stress and behavioral disorders (Capriello et al., 2021).
In this study, i.p. injection of LPS/AlCl3 in zebrafish has successfully induced acute inflammatory responses peripherally and centrally and enhanced brain AChE activity and oxidative stress. Importantly, acute i.p. administration of LPS/AlCl3 strongly impaired the spatial and contextual memory of zebrafish in the T-maze test. Collectively, these findings are generally consistent with previous evidence that LPS and other proinflammatory factors, as well as AlCl3, induce memory deficits in vertebrates (White et al., 1992; Hauss-Wegrzyniak et al., 1998, 2000; Rosi et al., 2003, 2004, 2005; Senger et al., 2011; Lee et al., 2018; Huang et al., 2019). Thus, two zebrafish memory deficit models have been established, induced by LPS and AlCl3, respectively. Their memory impairment mechanisms involve cholinergic, inflammatory, and oxidative-stress systems.
In these two models, HFFO supplementation reversed LPS/AlCl3-induced memory deficits, exhibiting its suppression of AChE activity and oxidative stress in the brain as well as central and peripheral inflammation. Supplementation with HFFO potently inhibited acute central and peripheral inflammation in the LPS-treated zebrafish. We speculate that this may be partially related to the underlying mechanism of the anti-inflammatory effect of phytol, which interacts with nuclear factor kappa B (NF-κB) to reduce interleukin-1beta (IL-1 β) and tumor necrosis factor (TNF-α) levels (Tak and Firestein, 2001; Silva et al., 2014). In addition, phytol can inhibit cyclooxygenase 2 (COX-2) via suppressing IL-1β and NF-κB (Islam et al., 2020). Besides, eicosatrienoic acid may also contribute to the anti-inflammatory effect of HFFO because some studies suggest eicosatrienoic acid has an anti-inflammatory role by suppressing NF-κB (Chen et al., 2015), and eicosatrienoic acid (all cis-7,-11,-14 20:3) can inhibit COX-2 (Baker et al., 2021). Moreover, the docking results of phytol with NF-κB and COX-2 and eicosatrienoic acid with NF-κB and COX-2 in this study also support this hypothesis to some extent. COX-2 is an important enzyme that catalyzes the conversion of arachidonic acid to prostaglandins. Under the condition of inhibition of COX-2 activity, arachidonic acid will be accumulated or converted to epoxyeicosatrienoic acid (EETs) by lipoxygenase and cytochrome P450 (CYP) epoxygenases (Strauss, 2008). EETs play a protective role in peripheral tissues by modulating a variety of cellular signaling pathways, and these effects may contribute to neuroprotection (Lakkappa et al., 2016). Treatment of AlCl3 and 200 mg/kg HFFO showed a significant pro-inflammatory effect compared with the treatment of AlCl3. We speculate that this may be because AlCl3 causes severe oxidative damage to neurons, and HFFO does not show a significant ability to alleviate such oxidative damage, which may further aggravate the inflammatory response. HFFO has a poor effect on such severe oxidative damage-inflammation in general. In addition, an excessive supply of exogenous arachidonic acid affected the inhibitory effect of phytol and eicosatrienoic acid on COX-2. This leads to the increased levels of prostaglandins produced by COX-2, which are linked to inflammation (Hamilton et al., 1999). However, HFFO has a better effect on LPS-induced inflammation.
The inhibition of brain AChE elevates ACh levels and hence may positively affect cognitive function (Scarpini et al., 2016). In this study, supplementation with low and medium doses of HFFO also dramatically reversed the ACh decrease in the LPS/AlCl3-treated zebrafish (Figure 3). This is consistent with our previous in vitro study that HFFO possesses in vitro AChE inhibitory activity and it mainly comes from ARA and ETrA (Yang et al., 2020). Another study also supports the AChE inhibitory activity of ARA (Ahmed et al., 2011).
Besides, MDA levels in lipid peroxidation degradation products reflect the level of lipid peroxidation in vivo and indirectly reflect the cellular damage and oxidative stress in brain tissue (Leutner et al., 2001; Long et al., 2009). In this study, MDA brain levels showed no significant change, 24 h after the LPS administration, which was speculated to be caused by a too low dose of LPS. However, MDA content in the low-, medium-, and high-dose HFFO groups decreased significantly, indicating that HFFO can reduce LPS-caused oxidative stress by inhibiting lipid peroxidation mildly. The antioxidant activity of HFFO may be related to the antioxidant mechanism of ARA or its metabolite EETs and phytol (Liu et al., 2011; Silva et al., 2014; Akimov et al., 2020). After 24 h of the injection of AlCl3, MDA levels increased. This is consistent with the increase in lipid peroxidation reported in the literature (Capriello et al., 2021). However, supplementation with HFFO in low and medium doses significantly increased MDA levels, while a high dose of HFFO showed no significantly lower MDA level compared to the model group. It is supposed that HFFO only possesses mild antioxidant activity, as it displayed in our previous in vitro study on LPS-induced BV-2 microglial cell lines (Yang et al., 2020) and this study on LPS-induced zebrafish. So, low and medium doses of HFFO can inhibit low-level lipoperoxidation caused by LPS but are not sufficient to counter high-level lipoperoxidation caused by aluminum. However, the abnormal MDA increase compared to the model group may be due to the unclear interaction between AlCl3 and HFFO, which remains to be further investigated in the future.
The assessment of pharmacological properties of compounds is deemed to be a crucial step in drug discovery (Cumming et al., 2013; Neggers et al., 2018). On account of the time and expense required to perform in vivo estimations, in silico approaches have become indispensable elements for drug discovery (Daina et al., 2017; Göller et al., 2020; Guo et al., 2021). Our study employed ADMETlab2.0, a multitask graph attention framework-based method, for the prediction of absorption, distribution, metabolism, excretion, and toxicity of the compounds (Guo et al., 2021). In silico prediction indicated that the main components of HFFO possessed acceptable ADMET properties, were capable of crossing the BBB, and obeyed one or more of the drug-likeness criteria. Among them, the arachidonic acid (ARA), the highest proportion of HFFO, showed the best performance in drug-likeness by respecting the Lipinski rule, Pfizer rule, golden triangle, and GSK rule. In addition, ARA, as well as docosahexaenoic acid (DHA), is a major polyunsaturated fatty acid in the human brain (Sun et al., 2018). The uptake of ARA in the human brain was generally greater than the uptake of DHA. After esterification in vivo, ARA is stored in the phospholipids of the cellular membranes and plays an important role in signal transduction by modulating membrane dynamics and activating receptors. Moreover, ARA is an important precursor of anandamide (AEA) and 2-arachidonoylglycerol (2-AG), which are regulators of synaptic neurotransmitter release (Busquets-Garcia et al., 2011). The aforementioned features render ARA a promising candidate for applying in the field of NDs. Notably, the main components of HFFO were predicted to carry a risk of skin sensitization and respiratory toxicity, which needs to be validated in further work. In addition, ARA was predicted to be a substrate of CYP1A2, CYP2C9, and CYP2D6. Therefore, it may be essential to avoid co-administration with CYP1A2, CYP2C9, and CYP2D6 inhibitors.
Recently, many studies have found that Hizikia fusiforme extracts have the function of improving learning and memory (Kuang and Zhang, 2014; Liu et al., 2018; Wang et al., 2021). Here, we found that its oil HFFO prevents cognitive deficits (induced by LPS/AlCl3 in zebrafish models) and exerts neuroprotective effects by anti-neuroinflammation and inhibition of acetylcholinesterase activity.
Conclusion
Collectively, the findings suggest HFFO as a potent neuroprotectant potentially valuable for the prevention of memory impairment caused by cholinergic deficiency and neuroinflammation.
Data availability statement
The original contributions presented in the study are included in the article/Supplementary material, further inquiries can be directed to the corresponding author.
Ethics statement
The animal study was reviewed and approved by the Animal Ethics Committee of Guangdong Ocean University, numbered 2020-6-20-1.
Author contributions
Y-YN and L-JZ performed the zebrafish experiment, calculation study, analyzed the data, and wrote the original draft. W-CY, Y-ML, X-XM, Z-YY, and Y-PZ prepared the compound sample and assisted in zebrafish experiments. YZ designed, guided the experiments, provided critical comments for the research, and revised the study. P-ZH and Y-YL provided critical comments during the research and polished the study. All authors have read and approved the final manuscript.
Funding
This study was funded by the special project in key fields of Guangdong Provincial Higher Education Institutions (Biomedicine and Health Care) with grant number (2021ZDZX2064), the Natural Science Foundation of Guangdong Province (2022A1515010783), the Basic Research Project of Shenzhen Science and Technology Innovation Commission with grant number (JCYJ20190813105005619), the Shenzhen Dapeng New District Scientific and Technological Research and Development Fund with grant number (KJYF202001-07), and the Innovation and Development Project about Marine Economy Demonstration of Zhanjiang City (XM-202008-01B1). The APC was funded by the Shenzhen Dapeng New District Scientific and Technological Research and Development Fund with grant number (KJYF202001-07), the Guangdong Provincial Special Project in Science and Technology (2021A05240), and the Guangdong Higher Education Institution Innovative Team with grant number (2021KCXTD021).
Conflict of interest
The authors declare that the research was conducted in the absence of any commercial or financial relationships that could be construed as a potential conflict of interest.
Publisher's note
All claims expressed in this article are solely those of the authors and do not necessarily represent those of their affiliated organizations, or those of the publisher, the editors and the reviewers. Any product that may be evaluated in this article, or claim that may be made by its manufacturer, is not guaranteed or endorsed by the publisher.
Supplementary material
The Supplementary Material for this article can be found online at: https://www.frontiersin.org/articles/10.3389/fnagi.2022.941994/full#supplementary-material
References
Ahmad Rather, M., Justin-Thenmozhi, A., Manivasagam, T., Saravanababu, C., Guillemin, G. J., and Essa, M. M. (2019). Asiatic acid attenuated aluminum chloride-induced tau pathology, oxidative stress and apoptosis via AKT/GSK-3β signaling pathway in wistar rats. Neurotoxic. Res. 35, 955–968. doi: 10.1007/s12640-019-9999-2
Ahmed, M., Latif, N., Khan, R. A., Ahmad, A., Thome, G., and Schetinger, M. R. C. (2011). Inhibitory effect of arachidonic acid on venom acetylcholinesterase. Toxicol. Environ. Chem. 93, 1659–1665. doi: 10.1080/02772248.2011.593292
Akimov, M. G., Dudina, P. V., Fomina-Ageeva, E. V., Gretskaya, N. M., Bosaya, A. A., Rudakova, E. V., et al. (2020). Neuroprotective and antioxidant activity of arachidonoyl choline, Its bis-quaternized analogues and other acylcholines. Dokl. Biochem. Biophys. 491, 93–97. doi: 10.1134/S1607672920020027
Auti, S., and Kulkarni, Y. (2019). Neuroprotective effect of cardamom oil against aluminum induced neurotoxicity in rats. Front. Neurol. 10, 399. doi: 10.3389/fneur.2019.00399
Bachurin, S. O., Bovina, E. V., and Ustyugov, A. A. (2017). Drugs in clinical trials for Alzheimer's disease: the major trends. Med. Res. Rev. 37, 1186–1225. doi: 10.1002/med.21434
Baker, E. J., Miles, E. A., and Calder, P. C. (2021). A review of the functional effects of pine nut oil, pinolenic acid and its derivative eicosatrienoic acid and their potential health benefits. Prog Lipid Res. 82, 101097. doi: 10.1016/j.plipres.2021.101097
Barbalace, M. C., Malaguti, M., Giusti, L., Lucacchini, A., Hrelia, S., and Angeloni, C. (2019). Anti-inflammatory activities of marine algae in neurodegenerative diseases. Int. J. Mol. Sci. 20, 3061. doi: 10.3390/ijms20123061
Barbosa, M., Valentão, P., and Andrade, P. B. (2014). Bioactive compounds from macroalgae in the new millennium: implications for neurodegenerative diseases. Mar. Drugs 12, 4934–4972. doi: 10.3390/md12094934
Bazinet, R. P., and Layé, S. (2014). Polyunsaturated fatty acids and their metabolites in brain function and disease. Nat. Rev. Neurosci. 15, 771–785. doi: 10.1038/nrn3820
Benfante, R., Di Lascio, S., Cardani, S., and Fornasari, D. (2021). Acetylcholinesterase inhibitors targeting the cholinergic anti-inflammatory pathway: a new therapeutic perspective in aging-related disorders. Aging Clin. Exp. Res. 33, 823–834. doi: 10.1007/s40520-019-01359-4
Bogie, J., Hoeks, C., Schepers, M., Tiane, A., Cuypers, A., Leijten, F., et al. (2019). Dietary Sargassum fusiforme improves memory and reduces amyloid plaque load in an Alzheimer's disease mouse model. Sci. Rep. 9, 4908. doi: 10.1038/s41598-019-41399-4
Busquets-Garcia, A., Puighermanal, E., Pastor, A., de la Torre, R., Maldonado, R., and Ozaita, A. (2011). Differential role of anandamide and 2-arachidonoylglycerol in memory and anxiety-like responses. Biol. Psychiatry. 70, 479–486. doi: 10.1016/j.biopsych.2011.04.022
Capriello, T., Félix, L. M., Monteiro, S. M., Santos, D., Cofone, R., and Ferrandino, I. (2021). Exposure to aluminium causes behavioural alterations and oxidative stress in the brain of adult zebrafish. Environ. Toxicol. Pharmacol. 85, 103636. doi: 10.1016/j.etap.2021.103636
Catanesi, M., Caioni, G., Castelli, V., Benedetti, E., d'Angelo, M., and Cimini, A. (2021). Benefits under the sea: the role of marine compounds in neurodegenerative disorders. Mar. Drugs. 19, 24. doi: 10.3390/md19010024
Catorce, M. N., and Gevorkian, G. (2016). LPS-induced murine neuroinflammation model: main features and suitability for pre-clinical assessment of nutraceuticals. Curr. Neuropharmacol. 14, 155–164. doi: 10.2174/1570159X14666151204122017
Chen, S. J., Chuang, L. T., and Chen, S. N. (2015). Incorporation of eicosatrienoic acid exerts mild anti-inflammatory properties in murine RAW264.7 cells. Inflammation. 38, 534–545. doi: 10.1007/s10753-014-9960-8
Choi, J. Y., Hwang, C. J., Lee, H. P., Kim, H. S., Han, S. B., and Hong, J. T. (2017). Inhibitory effect of ethanol extract of nannochloropsis oceanica on lipopolysaccharide-induced neuroinflammation, oxidative stress, amyloidogenesis and memory impairment. Oncotarget. 8, 45517–45530. doi: 10.18632/oncotarget.17268
Cumming, J. G., Davis, A. M., Muresan, S., Haeberlein, M., and Chen, H. (2013). Chemical predictive modelling to improve compound quality. Nat. Rev. Drug. Discov. 12, 948–962. doi: 10.1038/nrd4128
Cunningham, C., and Sanderson, D. J. (2008). Malaise in the water maze: untangling the effects of LPS and IL-1β on learning and memory. Brain Behav. Immun. 22, 1117–1127. doi: 10.1016/j.bbi.2008.05.007
Czerniawski, J., and Guzowski, J. F. (2014). Acute neuroinflammation impairs context discrimination memory and disrupts pattern separation processes in hippocampus. J. Neurosci. 34, 12470–12480. doi: 10.1523/JNEUROSCI.0542-14.2014
Daina, A., Michielin, O., and Zoete, V. (2017). SwissADME: a free web tool to evaluate pharmacokinetics, drug-likeness and medicinal chemistry friendliness of small molecules. Sci. Rep. 7, 42717. doi: 10.1038/srep42717
Giacobini, E., Spiegel, R., Enz, A., Veroff, A. E., and Cutler, N. R. (2002). Inhibition of acetyl- and butyryl-cholinesterase in the cerebrospinal fluid of patients with Alzheimer's disease by rivastigmine: correlation with cognitive benefit. J. Neural Transm. 109, 1053–1065. doi: 10.1007/s007020200089
Göller, A. H., Kuhnke, L., Montanari, F., Bonin, A., Schneckener, S., ter Laak, A., et al. (2020). Bayer's in silico ADMET platform: a journey of machine learning over the past two decades. Drug Discovery Today 25, 1702–1709. doi: 10.1016/j.drudis.2020.07.001
Gonçalves, A. F., Páscoa, I., Neves, J. V., Coimbra, J., Vijayan, M. M., Rodrigues, P., et al. (2012). The inhibitory effect of environmental ammonia on Danio rerio LPS-induced acute phase response. Dev. Comp. Immunol 36, 279–288. doi: 10.1016/j.dci.2011.04.008
Guo, L., Wu, Z., Yi, J., Fu, L., Yang, Z., Hsieh, C., et al. (2021). ADMETlab 2.0: an integrated online platform for accurate and comprehensive predictions of ADMET properties. Nucleic Acids Res. 51, 817–834.
Hamilton, L. C., Mitchell, J. A., Tomlinson, A. M., and Warner, T. D. (1999). Synergy between cyclo-oxygenase-2 induction and arachidonic acid supply in vivo: consequences for nonsteroidal anti-inflammatory drug efficacy. Faseb J. 13, 245–251. doi: 10.1096/fasebj.13.2.245
Han, J., Lee, Y., Im, J., Ham, Y., Lee, H., Han, S., et al. (2019). Astaxanthin ameliorates lipopolysaccharide-induced neuroinflammation, oxidative stress and memory dysfunction through inactivation of the signal transducer and activator of transcription 3 pathway. Mar. Drugs. 17, 123. doi: 10.3390/md17020123
Hauss-Wegrzyniak, B., Galons, J. P., and Wenk, G. L. (2000). Quantitative volumetric analyses of brain magnetic resonance imaging from rat with chronic neuroinflammation. Exp. Neurol. 165, 347–354. doi: 10.1006/exnr.2000.7469
Hauss-Wegrzyniak, B., Lukovic, L., Bigaud, M., and Stoeckel, M. E. (1998). Brain inflammatory response induced by intracerebroventricular infusion of lipopolysaccharide: an immunohistochemical study. Brain Res. 794, 211–224. doi: 10.1016/S0006-8993(98)00227-3
Hu, P., Li, Z., Chen, M., Sun, Z., Ling, Y., Jiang, J., et al. (2016). Structural elucidation and protective role of a polysaccharide from Sargassum fusiforme on ameliorating learning and memory deficiencies in mice. Carbohydr. Polym. 139, 150–158. doi: 10.1016/j.carbpol.2015.12.019
Huang, C., Ng, O. T., Chu, J. M., Irwin, M. G., Hu, X., Zhu, S., et al. (2019). Differential effects of propofol and dexmedetomidine on neuroinflammation induced by systemic endotoxin lipopolysaccharides in adult mice. Neurosci. Lett. 707, 134309. doi: 10.1016/j.neulet.2019.134309
Huat, T. J., Camats-Perna, J., Newcombe, E. A., Valmas, N., Kitazawa, M., and Medeiros, R. (2019). Metal toxicity links to Alzheimer's disease and neuroinflammation. J. Mol. Biol 431, 1843–1868. doi: 10.1016/j.jmb.2019.01.018
Hughes, J. D., Blagg, J., Price, D. A., Bailey, S., Decrescenzo, G. A., Devraj, R. V., et al. (2008). Physiochemical drug properties associated with in vivo toxicological outcomes. Bioorg. Med. Chem. Lett. 18, 4872–4875. doi: 10.1016/j.bmcl.2008.07.071
Islam, M. T., Ayatollahi, S. A., Zihad, S., Sifat, N., and Sharifi-Rad, J. (2020). Phytol anti-inflammatory activity: pre-clinical assessment and possible mechanism of action elucidation. Cell. Mol. Biol. 66, 264–269. doi: 10.14715/cmb/2020.66.4.31
Ivanenkov, Y. A., Zagribelnyy, B. A., and Aladinskiy, V. A. (2019). Are we opening the door to a new era of medicinal chemistry or being collapsed to a chemical singularity? J. Med. Chem. 62, 10026–10043. doi: 10.1021/acs.jmedchem.9b00004
Jiang, D. J., Lei, T. L., Wang, Z., Shen, C., Cao, D. S., and Hou, T. J. (2020). ADMET evaluation in drug discovery. 20. prediction of breast cancer resistance protein inhibition through machine learning. J. Cheminf. 12, 16. doi: 10.1186/s13321-020-00421-y
Johnson, T. W., Dress, K. R., and Edwards, M. (2009). Using the golden triangle to optimize clearance and oral absorption. Bioorg. Med. Chem. Lett. 19, 5560–5564. doi: 10.1016/j.bmcl.2009.08.045
Kuang, M. L., and Zhang, J. (2014). Effects of Sargassum fusiforme phlorotanninon on the senile dementia mouse model induced by D-galactose. Guangdong Med. J. 35, 1984–1986.
Kumar, D. K., Choi, S. H., Washicosky, K. J., Eimer, W. A., Tucker, S., Ghofrani, J., et al. (2016). Amyloid-β peptide protects against microbial infection in mouse and worm models of Alzheimer's disease. Sci. Transl. Med 8, 340ra372. doi: 10.1126/scitranslmed.aaf1059
Lakkappa, N., Krishnamurthy, P. T., Hammock, B. D., Velmurugan, D., and Bharath, M. M. (2016). Possible role of epoxyeicosatrienoic acid in prevention of oxidative stress mediated neuroinflammation in Parkinson disorders. Med. Hypotheses 93, 161–165. doi: 10.1016/j.mehy.2016.06.003
Lee, Y., Lee, S., Park, J. W., Hwang, J. S., Kim, S. M., Lyoo, I. K., et al. (2018). Hypoxia-induced neuroinflammation and learning-memory impairments in adult zebrafish are suppressed by glucosamine. Mol. Neurobiol 55, 8738–8753. doi: 10.1007/s12035-018-1017-9
Leutner, S., Eckert, A., and Müller, W. E. (2001). ROS generation, lipid peroxidation and antioxidant enzyme activities in the aging brain. J. Neural Transm. 108, 955–967. doi: 10.1007/s007020170015
Lipinski, C. A., Lombardo, F., Dominy, B. W., and Feeney, P. J. (2001). Experimental and computational approaches to estimate solubility and permeability in drug discovery and development settings. Adv. Drug Delivery Rev. 46, 3–26. doi: 10.1016/S0169-409X(00)00129-0
Liu, H., Ying, M., Shi, W., Xie, J., Yang, J., and Wang, Z. (2018). Effect of Sargassum fusiforme polysaccharides with different relative molecular weights on learning and memory ability in mice model of memory impairment induced by raceanisodamine. Food Sci. 39, 221–225.
Liu, J., Luthuli, S., Yang, Y., Cheng, Y., and Tong, H. (2020). Therapeutic and nutraceutical potentials of a brown seaweed Sargassum fusiforme. Food Sci. Nutr. 8, 5195–5205. doi: 10.1002/fsn3.1835
Liu, L., Chen, C., Gong, W., Li, Y., Edin, M. L., Zeldin, D. C., et al. (2011). Epoxyeicosatrienoic acids attenuate reactive oxygen species level, mitochondrial dysfunction, caspase activation, and apoptosis in carcinoma cells treated with arsenic trioxide. J. Pharmacol. Exp. Ther. 339, 451–463. doi: 10.1124/jpet.111.180505
Liu, Y., Zhang, Z., Yang, C., Meng, L., and Yang, J. (2013). Influence of citric acid on aluminum-induced neurotoxicity in CA3 and dentate gyrus regions of mice hippocampus. Fresenius Environ. Bull. 22, 474–479. Available online at: https://www.webofscience.com/wos/alldb/full-record/WOS:000321721700001
Livingston, G., Huntley, J., Sommerlad, A., Ames, D., Ballard, C., Banerjee, S., et al. (2020). Dementia prevention, intervention, and care: 2020 report of the Lancet commission. The Lancet 396, 413–446. doi: 10.1016/S0140-6736(20)30367-6
Long, J., Liu, C., Sun, L., Gao, H., and Liu, J. (2009). Neuronal mitochondrial toxicity of malondialdehyde: inhibitory effects on respiratory function and enzyme activities in rat brain mitochondria. Neurochem. Res. 34, 786–794. doi: 10.1007/s11064-008-9882-7
Mathiyazahan, D. B., Justin Thenmozhi, A., and Manivasagam, T. (2015). Protective effect of black tea extract against aluminium chloride-induced Alzheimer's disease in rats: a behavioural, biochemical and molecular approach. J. Funct. Foods 16, 423–435. doi: 10.1016/j.jff.2015.05.001
Mcbean, G. J., López, M., and Wallner, F. K. (2016). Redox-based therapeutics in neurodegenerative disease. Br. J. Pharmacol 174, 1750–1770. doi: 10.1111/bph.13551
McGrattan, A. M., McGuinness, B., McKinley, M. C., Kee, F., Passmore, P., Woodside, J. V., et al. (2019). Diet and iInflammation in cognitive ageing and Alzheimer's disease. Curr. Nutr. Rep. 8, 53–65. doi: 10.1007/s13668-019-0271-4
Meinita, M. D. N., Harwanto, D., Sohn, J.-H., Kim, J.-S., and Choi, J.-S. (2021). Hizikia fusiformis: pharmacological and nutritional properties. Foods 10, 1660. doi: 10.3390/foods10071660
Ming, Z., Wotton, C. A., Appleton, R. T., Ching, J. C., Loewen, M. E., Sawicki, G., et al. (2015). Systemic lipopolysaccharide-mediated alteration of cortical neuromodulation involves increases in monoamine oxidase-A and acetylcholinesterase activity. J. Neuroinflammation 12, 37. doi: 10.1186/s12974-015-0259-y
Neggers, J. E., Kwanten, B., Dierckx, T., Noguchi, H., Voet, A., Bral, L., et al. (2018). Target identification of small molecules using large-scale CRISPR-Cas mutagenesis scanning of essential genes. Nat. Commun. 9, 502. doi: 10.1038/s41467-017-02349-8
Nie, Y. Y., Yang, J. M., Zhou, L. J., Yang, Z. Y., Liang, J. Y., Liu, Y. Y., et al. (2022). Marine fungal metabolite butyrolactone I prevents cognitive deficits by relieving inflammation and intestinal microbiota imbalance on aluminum trichloride-injured zebrafish. J. Neuroinflammation 19(1). doi: 10.1186/s12974-022-02403-3
Petersson, S. D., and Philippou, E. (2016). Mediterranean diet, cognitive function, and dementia: a systematic review of the evidence. Adv. Nutr. 7, 889–904. doi: 10.3945/an.116.012138
Ransohoff, R. M. (2016). How neuroinflammation contributes to neurodegeneration. Sci. 353, 777–783. doi: 10.1126/science.aag2590
Rishitha, N., and Muthuraman, A. (2018). Therapeutic evaluation of solid lipid nanoparticle of quercetin in pentylenetetrazole induced cognitive impairment of zebrafish. Life Sci. 199, 80–87. doi: 10.1016/j.lfs.2018.03.010
Rosi, A., Pert, B., Ruff, B., Gann Gramling, A., and Wenk, A. (2005). Chemokine receptor 5 antagonist D-ala-peptide T-amide reduces microglia and astrocyte activation within the hippocampus in a neuroinflammatory rat model of Alzheimer's disease. Neurosci. 134, 671–676. doi: 10.1016/j.neuroscience.2005.04.029
Rosi, S., Mcgann, K., Hauss-Wegrzyniak, B., and Wenk, G. L. (2003). The influence of brain inflammation upon neuronal adenosine A2B receptors. J. Neurochem 86, 220–227. doi: 10.1046/j.1471-4159.2003.01825.x
Rosi, S., Ramirez-Amaya, V., Hauss-Wegrzyniak, B., and Wenk, G. L. (2004). Chronic brain inflammation leads to a decline in hippocampal NMDA-R1 receptors. J. Neuroinflammation 1, 12. doi: 10.1186/1742-2094-1-12
Scarpini, E., and Galimberti, Daniela. (2016). Old and new acetylcholinesterase inhibitors for Alzheimer's disease. Expert Opin. Invest. Drugs 25, 1181–1187. doi: 10.1080/13543784.2016.1216972
Sebai, H., Gadacha, W., Sani, M., Aouani, E., Ghanem-Boughanmi, N., and Ben-Attia, M. (2009). Protective effect of resveratrol against lipopolysaccharide-induced oxidative stress in rat brain. Brain Inj. 23, 1089–1094. doi: 10.3109/02699050903379370
Senger, M. R., Seibt, K. J., Ghisleni, G. C., Dias, R. D., Bogo, M. R., and Bonan, C. D. (2011). Aluminum exposure alters behavioral parameters and increases acetylcholinesterase activity in zebrafish (Danio rerio) brain. Cell Biol. Toxicol. 27, 199–205. doi: 10.1007/s10565-011-9181-y
Silva, R. O., Sousa, F. B., Damasceno, S. R., Carvalho, N. S., Silva, V. G., Oliveira, F. R., et al. (2014). Phytol, a diterpene alcohol, inhibits the inflammatory response by reducing cytokine production and oxidative stress. Fundam. Clin. Pharmacol. 28, 455–464. doi: 10.1111/fcp.12049
Strauss, K. I. (2008). Antiinflammatory and neuroprotective actions of COX2 inhibitors in the injured brain. Brain Behav. Immun. 22, 285–298. doi: 10.1016/j.bbi.2007.09.011
Sun, G. Y., Simonyi, A., Fritsche, K. L., Chuang, D. Y., Hannink, M., Gu, Z., et al. (2018). Docosahexaenoic acid (DHA): An essential nutrient and a nutraceutical for brain health and diseases. Prostaglandins Leukot. Essent. Fatty Acids 136, 3–13. doi: 10.1016/j.plefa.2017.03.006
Tabet, N. (2006). Acetylcholinesterase inhibitors for Alzheimer's disease: anti-inflammatories in acetylcholine clothing! Age Ageing 35, 336–338. doi: 10.1093/ageing/afl027
Tak, P. P., and Firestein, G. S. (2001). NF-kappaB: a key role in inflammatory diseases. J. Clin. Invest. 107, 7–11. doi: 10.1172/JCI11830
Trépanier, M. O., Hopperton, K. E., Orr, S. K., and Bazinet, R. P. (2016). N-3 polyunsaturated fatty acids in animal models with neuroinflammation: an update. Eur. J. Pharmacol. 785, 187–206. doi: 10.1016/j.ejphar.2015.05.045
Trott,. O., and Olson, A. J. (2010). AutoDock Vina: improving the speed and accuracy of docking with a new scoring function, efficient optimization, and multithreading. J. Comput. Chem. 31, 455–461. doi: 10.1002/jcc.21334
Tyagi, E., Agrawal, R., Nath, C., and Shukla, R. (2008). Influence of LPS-induced neuroinflammation on acetylcholinesterase activity in rat brain. J. Neuroimmunol 205, 51–56. doi: 10.1016/j.jneuroim.2008.08.015
Tyagi, E., Agrawal, R., Nath, C., and Shukla, R. (2010). Cholinergic protection via alpha7 nicotinic acetylcholine receptors and PI3K-Akt pathway in LPS-induced neuroinflammation. Neurochem. Int. 56, 135–142. doi: 10.1016/j.neuint.2009.09.011
Uttara, B., Singh, A. V., Zamboni, P., and Mahajan, R. T. (2009). Oxidative stress and neurodegenerative diseases: a review of upstream and downstream antioxidant therapeutic options. Curr. Neuropharmacol. 7, 65–74. doi: 10.2174/157015909787602823
Wang, L., Oh, J. Y., Yang, H. W., Fu, X., Kim, J. I., and Jeon, Y. J. (2021). Fucoidan isolated from the popular edible brown seaweed Sargassum fusiforme suppresses lipopolysaccharide-induced inflammation by blocking NF-κB signal pathway. J. Appl. Phycol 33, 1–8. doi: 10.1007/s10811-021-02390-7
Westerfield, M. (2007). The Zebrafish Book. A Guide for the Laboratory Use of Zebrafish (Danio rerio). Eugene: University of Oregon Press.
White, D. M., Longstreth, W. T. Jr., Rosenstock, L., Claypoole, K. H., Brodkin, C. A., and Townes, B. D. (1992). Neurologic syndrome in 25 workers from an aluminum smelting plant. Arch. Intern. Med. 152, 1443–1448. doi: 10.1001/archinte.1992.00400190071014
Whitehouse, P. J., Price, D. L., Struble, R. G., Clark, A. W., Coyle, J. T., and Delon, M. R. (1982). Alzheimer's disease and senile dementia: loss of neurons in the basal forebrain. Science. 215, 1237–1239. doi: 10.1126/science.7058341
Yang, W. C., Zhang, Y. Y., Li, Y. J., Nie, Y. Y., and Zhang, Y. (2020). Chemical composition and anti-Alzheimer's disease-related activities of a functional oil from the edible seaweed Hizikia fusiforme. Chem. Bio. 17, e2000055. doi: 10.1002/cbdv.202000055
Keywords: oxidative stress, neuroinflammation, HFFO, memory impairment, ADMET
Citation: Nie Y-Y, Zhou L-J, Li Y-M, Yang W-C, Liu Y-Y, Yang Z-Y, Ma X-X, Zhang Y-P, Hong P-Z and Zhang Y (2022) Hizikia fusiforme functional oil (HFFO) prevents neuroinflammation and memory deficits evoked by lipopolysaccharide/aluminum trichloride in zebrafish. Front. Aging Neurosci. 14:941994. doi: 10.3389/fnagi.2022.941994
Received: 13 May 2022; Accepted: 05 August 2022;
Published: 09 September 2022.
Edited by:
Zhigang Liu, Northwest A&F University, ChinaReviewed by:
Jianrong Xu, Shanghai University of Traditional Chinese Medicine, ChinaGuoyuan Qi, University of Arizona, United States
Copyright © 2022 Nie, Zhou, Li, Yang, Liu, Yang, Ma, Zhang, Hong and Zhang. This is an open-access article distributed under the terms of the Creative Commons Attribution License (CC BY). The use, distribution or reproduction in other forums is permitted, provided the original author(s) and the copyright owner(s) are credited and that the original publication in this journal is cited, in accordance with accepted academic practice. No use, distribution or reproduction is permitted which does not comply with these terms.
*Correspondence: Yi Zhang, zhangyi@gdou.edu.cn; hubeizhangyi@163.com
†These authors have contributed equally to this work