tDCS over the primary motor cortex contralateral to the trained hand enhances cross-limb transfer in older adults
- 1Department of Movement Neuroscience, Faculty of Sport Science, Leipzig University, Leipzig, Germany
- 2Department of Neurology, Max Planck Institute for Human Cognitive and Brain Sciences, Leipzig, Germany
- 3Pediatric Epidemiology, Department of Pediatrics, Medical Faculty, Leipzig University, Leipzig, Germany
- 4Berlin School of Mind and Brain, Humboldt-Universität zu Berlin, Berlin, Germany
- 5Charité-Universitätsmedizin Berlin, Humboldt-Universität zu Berlin, Berlin, Germany
Transferring a unimanual motor skill to the untrained hand, a phenomenon known as cross-limb transfer, was shown to deteriorate as a function of age. While transcranial direct current stimulation (tDCS) ipsilateral to the trained hand facilitated cross-limb transfer in older adults, little is known about the contribution of the contralateral hemisphere to cross-limb transfer. In the present study, we investigated whether tDCS facilitates cross-limb transfer in older adults when applied over the motor cortex (M1) contralateral to the trained hand. Furthermore, the study aimed at investigating short-term recovery of tDCS-associated cross-limb transfer. In a randomized, double-blinded, sham-controlled setting, 30 older adults (67.0 ± 4.6 years, 15 female) performed a short grooved-pegboard training using their left hand, while anodal (a-tDCS) or sham-tDCS (s-tDCS) was applied over right M1 for 20 min. Left (LHtrained) - and right-hand (RHuntrained) performance was tested before and after training and in three recovery measures 15, 30 and 45 min after training. LHtrained performance improved during both a-tDCS and s-tDCS and improvements persisted during recovery measures for at least 45 min. RHuntrained performance improved only following a-tDCS but not after s-tDCS and outlasted the stimulation period for at least 45 min. Together, these data indicate that tDCS over the M1 contralateral to the trained limb is capable of enhancing cross-limb transfer in older adults, thus showing that cross-limb transfer is mediated not only by increased bi-hemispheric activation.
Introduction
During the aging process, the capability of learning novel motor skills declines (Voelcker-Rehage, 2008; Roig et al., 2014; VanSwearingen and Studenski, 2014; Cirillo, 2021). Additionally, motor performance becomes less reliable and a series of basic motor functions such as maximal strength and force steadiness but also performance of complex fine motor tasks decreases as a function of age (Voelcker-Rehage, 2008; Hunter et al., 2016). Motor dexterity is one of the key functions for the ability of independent living (Ranganathan et al., 2001a; Choi et al., 2017) and is defined as the ability to perform coordinated finger movements in order to grasp and manipulate small objects (Makofske, 2011). Research showed that for complex fine motor tasks, a stable level of motor dexterity is maintained until around 70–75 years (Hoogendam et al., 2014), while after this critical age period, performance declines rapidly (Smith et al., 1999). In simpler fine motor tasks, linear effects of age on manual dexterity have been reported, starting around the age of 65 years (Ranganathan et al., 2001a; Adler et al., 2002; Hoogendam et al., 2014). Among others, age-related impairments in manual dexterity were found regarding sensory functioning (Cole et al., 1999; Goble et al., 2009) and the ability to grasp small objects (Sears and Chung, 2010; Friedman et al., 2011). One underlying factor for age-related impairments is an age-related reorganization of the brain on a functional and structural level (Peters, 2006; Zapparoli et al., 2022). On the structural level, this age-related process manifests itself in a loss of fibers in gray matter (Sowell et al., 2003) but also white matter alterations (Guttmann et al., 1998; Pantoni, 2010) as well as chemical changes, such as a decrease of dopamine and serotonin receptors (Iyo and Yamasaki, 1993; Seidler et al., 2010). On the functional level, aging is associated with a reduction of task-based hemispheric asymmetry (Cabeza, 2002), which was found to be a compensatory mechanism associated with improved performance in some studies (Cabeza et al., 2018) while other findings indicate a rather non-specific mechanism of dedifferentiation (Cassady et al., 2020; Knights et al., 2021).
Transcranial direct-current stimulation (tDCS) has been identified as an effective and non-invasive strategy to modulate brain function (Nitsche and Paulus, 2000; Borchers et al., 2011; Kunze et al., 2016; Mancini et al., 2016) and subsequently also behavior (Jacobson et al., 2012). One major field of tDCS research is the stimulation of motor-relevant brain areas and its effects on motor skill learning and performance (Reis and Fritsch, 2011; Ammann et al., 2016; Buch et al., 2017), pointing toward the fact that tDCS might be able to support maintenance or even restoration of motor dexterity. Indeed, research indicates that motor dexterity can be facilitated using tDCS over the primary motor cortex M1, (Boggio et al., 2006; Sohn et al., 2012; Karok and Witney, 2013; Kidgell et al., 2013; Convento et al., 2014). In old adults, improvements in motor performance were found via concurrent stimulation over the M1 contralateral to the trained hand (Hummel et al., 2010; Goodwill et al., 2013; Zimerman et al., 2013) even though other studies only found improvements in case of non-dominant M1 stimulation (Marquez et al., 2015). Furthermore, M1 tDCS affects retention of motor learning suggesting that tDCS concurrently applied with motor practice supports older adults to retain their improvements in performance (Parikh and Cole, 2014).
It is known that even simple unimanual motor tasks induce bilateral increases in cortical excitability (Cramer et al., 1999; L. Lee et al., 2003; Koeneke et al., 2006; Carroll et al., 2008), presumably due to hemispheric specialization in controlling certain movement features like movement speed, acceleration, movement duration or final position information (Sainburg and Wang, 2002; Schaefer et al., 2009). However, even though each hemisphere may be related to certain distinct features, information are transferred between hemispheres (Schaefer et al., 2009). The ability to perform a motor task with the opposite, untrained limb after learning with the other limb is a phenomenon known as cross-limb transfer (van Mier and Petersen, 2006; Koeneke et al., 2009; Stockel and Wang, 2011; Pan and Van Gemmert, 2013). In previous studies, mainly M1 ipsilateral to the trained limb was investigated with regard to its contribution in cross-limb transfer (Bodwell et al., 2003; M. Lee et al., 2010). In older adults, anodal tDCS (a-tDCS) applied over the M1 ipsilateral to the trained limb facilitated cross-limb transfer and increased motor overflow in the untrained hand (Goodwill et al., 2015). Since motor overflow is associated with increased bi-hemispheric activity (Bodwell et al., 2003), cross-limb transfer effects were mainly attributed to the increase in untrained hemisphere activation induced by tDCS. However, greater bilateral activation during unimanual motor tasks does not necessarily manifest in more pronounced cross-limb transfer (Hinder et al., 2011). Furthermore, other studies found cross-limb transfer impairments after enhancement of M1 ipsilateral to the trained limb (Keitel et al., 2018) and others even found cross-limb transfer enhancement after suppression of the ipsilateral M1 (Kobayashi et al., 2009). Thus, it might be possible that other mechanisms than bi-hemispheric activation are responsible for cross-limb transfer improvements. Therefore, finding evidence on how tDCS over M1 contralateral to the trained limb affects cross-limb transfer in a unimanual motor task would be of great importance. Besides, studying cross-limb transfer as a mechanism to improve fine motor performance is of high practical relevance. For some old adults, active hand training is impossible due to disease or injury and even in healthy aging, cross-limb transfer effects could be used for example to align asymmetrical fine motor abilities. Furthermore, using a neuromodulatory tool such as tDCS during motor task performance may help compensating age-related reductions in brain function (Hummel et al., 2010; Cabeza et al., 2018) and thereby modulate neuroplasticity (Zimerman and Hummel, 2010). On a more general note, finding strategies to maintain or restore fine motor function aids to keep old people an active part of society since fine motor abilities are an important prerequisite of independent living (Ranganathan et al., 2001b; Choi et al., 2017).
In the current study, we aimed at assessing the effect of a single a-tDCS session over right M1 while older adults performed the grooved pegboard test (GPT) with their left hand (LHtrained). Cross-limb transfer was investigated in the right, untrained hand (RHuntrained) before and after LH training. The GPT was previously verified as a reliable measure of motor dexterity (Desrosiers et al., 1995) and has been attributed to general cognitive functioning in older age (Ashendorf et al., 2009). Motor performance measures of LHtrained and RHuntrained included three recovery measurements 15, 30 and 45 min after LH training to assess possible tDCS after-effects, which were previously found within 1 h after termination of the stimulation (Koo et al., 2016) and can be regarded as markers of ongoing neuroplastic processes (Koo et al., 2016). We hypothesized that motor performance of the RHuntrained improves after a-tDCS over right M1 and that improvements outlast the stimulation period, pointing toward the capability of tDCS contralateral to the trained limb to persistently facilitate cross-limb transfer. Furthermore, we hypothesized that in line with previous studies, a-tDCS over right M1 also facilitates motor performance of LHtrained (Hummel et al., 2010; Goodwill et al., 2013; Zimerman et al., 2013; Parikh and Cole, 2014).
Materials and methods
Ethical approval
This study was approved by the local ethics committee of Leipzig University (ref. nr. 202-13-150-72013). All participants provided written informed consent and all procedures were conducted in accordance with the Declaration of Helsinki.
Participants
An a priori sample size calculation (G*Power 3.1) specified a total sample size of 22 participants to obtain a moderate effect size f = 0.25 and power of 1-β = 0.8 using a mixed model with two groups and five measurement points and a significance level of α < 0.05. A moderate effect size was assumed since a recent meta-analysis (Summers et al., 2016) found a robust medium effect (standardized mean difference = 0.65) for the modulation of motor task performance in older adults using tDCS. To ensure high enough sample size also in cases of drop outs, we enrolled a total of 32 volunteers (16 female). Participants were included according to the following criteria: (a) age between 60 and 80 years, (b) a score of at least 24 on the Mini-Mental State Examination (MMSE), and (c) right-handedness according to the Edinburgh Handedness Inventory (Oldfield, 1971). The exclusion criteria were as follows: (a) cognitive impairments, (b) focal cortical lesions, (c) neurological or psychiatric disorders, (d) drug abuse, (e) previous neurosurgical operations, (f) migraine, (g) epilepsy or other cerebral seizures, (h) a pacemaker, (i) intracranial metal clips (j) cochlear implants, (k) liquor-shunt, (l) pregnancy, (m) sleep disorders, or (n) brain activity influencing medication. All participants were neurologically assessed by a qualified physician before the experiment. Following this examination, one female participant was excluded because of migraine and one male participant because of a strong manual tremor. Finally, 30 healthy adults (15 female, 15 male; age: 67.0 ± 4.6 years (mean ± standard deviation); laterality quotient (LQ): 93.7 ± 11.7) were included in the experiment. Participants were randomly assigned to one tDCS-stimulation group (anodal tDCS (a-tDCS); n = 15) or a sham-group (s-tDCS; n = 15). The number of hours of physical exercise and video gaming during an average week was assessed with a questionnaire to control for potential confounding factors on manual dexterity. Additionally, the amount of sleep (h) before the experiment was assessed. To assess baseline levels of attention, the d2-R test (Brickenkamp et al., 2010) was performed before the experiment. Here, the parameter of accuracy (total of crossed-out items divided by errors) was used as the main outcome measure. An overview of participant characteristics is provided in Table 1. All participants received monetary compensation for their participation.
Experimental procedure
In this randomized, double-blinded, sham-controlled study (see Figure 1A), participants had to perform a manual dexterity task called the grooved pegboard test (GPT). Thereafter, GPT was completed once, as a baseline measure, with each hand (left hand: LH; right hand: RH) before tDCS was applied to the right M1 (PRE) but with the tDCS-electrodes already attached to the scalp. Next, a-tDCS was applied for a total duration of 20 min and was initiated 10 min before participants started training GPT performance. The training consisted of a total of 4 learning trials (LT1-LT4) with the left (non-dominant) hand (LHtrained) including rest phases of 90 seconds in between each successive trial. After a-tDCS or s-tDCS, GPT performance of both hands (POST) was immediately re-assessed. Additionally, 3 recovery trials (REC1, REC2, REC3) with 15-min breaks between each of the trials were performed to assess tDCS after-effects. The order of LH or RH trials during PRE, POST, REC1, REC2, and REC3 were randomized. Before and after tDCS, a Visual Analogue Scale (VAS) was used to measure participants’ level of attention, tiredness, and pain (scale from 1 to 10). All experiments were performed in a laboratory environment with an ambient temperature between 19 and 22°C.
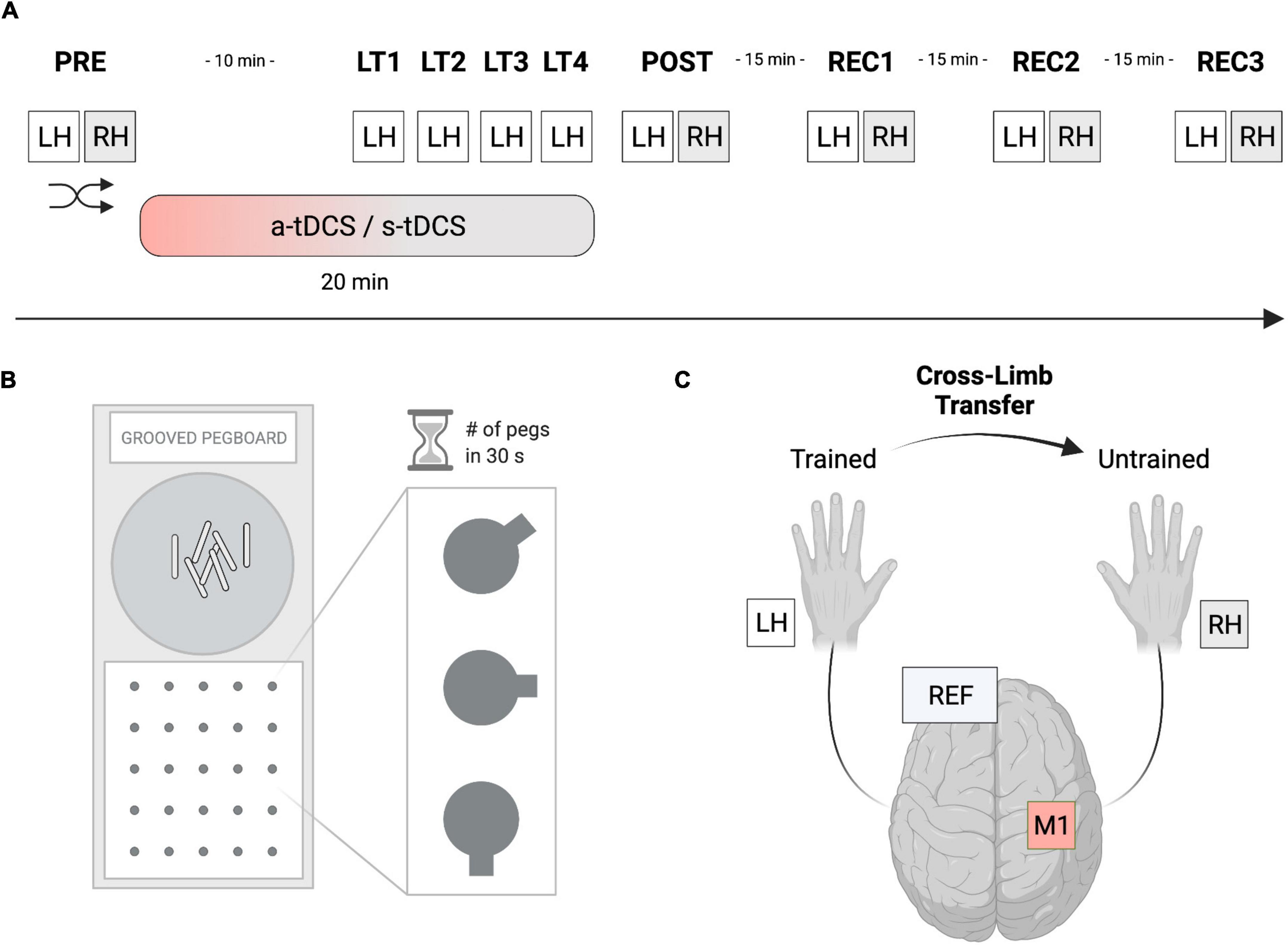
Figure 1. Experimental setup. (A) In this randomized, double-blinded, sham-controlled study, participants had to perform a manual dexterity task called the Grooved Pegboard Test (GPT). The baseline performance of both hands was assessed (PRE), before performance of the left hand (LHtrained) was investigated during 4 learning trials (LT1-LT4). Thereafter, performance improvements of both hands were reassessed (POST). Furthermore, 3 recovery trials (REC1-REC3) were performed, separated by 15 min of rest to assess tDCS after-effects. Anodal transcranial direct current stimulation (a-tDCS) or sham stimulation (s-tDCS) was applied for 20 min during motor practice of LHtrained. The order of left- or right-hand testing was randomized per timepoint. (B) The Grooved Pegboard Test (GPT) was implemented to investigate eye-hand coordination and motor speed for manual dexterity. (C) During a-tDCS and s-tDCS, LHtrained performed the GPT while RHuntrained was resting. Anodal tDCS of the right M1 was applied for 20 min with the reference electrode placed on the left supraorbital cortex. Created with Biorender.com.
Manual dexterity task—grooved pegboard test
The GPT (Model 32025, Lafayette Instrument, United States) was used to measure eye-hand coordination and motor speed for manual dexterity (see Figure 1B). The test consists of a board with a matrix of five-by-five holes, each containing a groove pointing in a specific direction. In contrast to the Purdue Pegboard Test, where the holes are round, participants had to rotate the metal pins called Pegs to fit into the grooves of the board matrix. This design added another level of complexity to the manual task requiring not only motor abilities but also visuomotor coordination. For each trial, the number of pegs participants were able to correctly put into the respective slot was measured within 30 s as the outcome measure of interest. Participants always started on the upper left-hand side of the matrix continuing on the row to the right side before switching to a lower row. The trial time of 30 s was measured with a stopwatch and only fully completed pegs were counted. In the starting position, the participants sat comfortably, with the pegboard aligned straight in front of them and both hands resting next to it. The pegs were picked up by the participant from the intended area of the board (see Figure 1).
Transcranial direct current stimulation
Transcranial direct current stimulation (tDCS) was applied via a battery-driven stimulator (neuroConn GmbH, Ilmenau, Germany) with two attached electrodes (see Figure 1C). The active (anodal) electrode had a size of 5 × 5 cm while an electrode size of 10 × 10 cm was chosen for the reference (cathodal) electrode. According to the 10–20 system, the active electrode was positioned over the right M1 contralateral to LHtrained (electrode position C4). The reference electrode was placed on the contralateral (left) supraorbital cortex. The scalp was first rubbed with alcohol, then both electrodes were soaked in saline and fixed to the scalp with rubber bands. A-tDCS of 1 mA was applied for 20 min with a fade-in and fade-out period of 30 s each. During s-tDCS, the current was ramped up for 30 s, held constant at 1 mA for 30 s, and ramped down for 30 s. This short duration of stimulation has been shown to elicit no changes in cortical excitability while it may provide the same tingling sensation on the scalp of the participant (Nitsche et al., 2008). Electrode montage, active electrode size (5 × 5 cm) and current intensity were essentially adopted from a previous study (Goodwill et al., 2015). However, a larger reference electrode was used to minimize the influence of cathodal frontal cortex stimulation (Kaminski et al., 2013). Generally, the impedance was monitored and kept under 10 Ω. Both the researcher and the participants were blinded.
Statistical analysis
All statistical analyses were performed using JASP (Version 0.16, JASP Team 2021). The majority of the GPT performance variables were normally distributed according to Shapiro–Wilk-Tests (α = 0.05). Demographic and handedness variables, d2-R accuracy as well as other questionnaire variables (VAS) were not normally distributed. To compare these variables between a-tDCS and s-tDCS, non-parametric Mann–Whitney U tests were used.
Baseline GPT performance of both hands (LHtrained and RHuntrained) was compared between groups (a-tDCS, s-tDCS) using independent samples t-tests. Subsequently, all individual GPT values obtained at POST, REC1, REC2, and REC3 were normalized to values obtained at PRE. This normalization procedure was done separately for each hand. Furthermore, individual motor learning trials (LT1-LT4) were also normalized to baseline GPT performance of LHtrained obtained during PRE.
GPT performance of LHtrained was assessed using a repeated-measures ANOVA with the within-subject factor TRIAL (PRE, LT1, LT2, LT3, LT4, POST), the between-subject factor GROUP (a-tDCS, s-tDCS) and sex of the participants as covariate.
GPT performance during recovery trials and cross-limb transfer effects to RHuntrained was assessed using repeated-measures ANOVAs with the within-subject factor TRIAL (PRE, POST, REC1, REC2, REC3), the between-subject factor GROUP (a-tDCS, s-tDCS) and sex as covariate for each hand separately.
VAS ratings of attention, tiredness, and pain before and after stimulation were compared within groups using Wilcoxon-signed rank tests. Additionally, VAS ratings were compared between groups (a-tDCS, s-tDCS) using separate Mann–Whitney U tests.
The statistical threshold was set at p = 0.05 for all analyses. For ANOVAs, partial eta squared (ηp2) was used to report effect sizes while Cohen’s d was used for Bonferroni corrected post hoc comparisons. Effect sizes for the pairwise Mann–Whitney U test were expressed with the rank-biserial correlation coefficient (r). When the sphericity assumption of repeated-measures ANOVAs was violated, a Greenhouse-Geisser correction was applied.
Results
Participant characteristics
Participants of the randomly allocated groups a-tDCS and s-tDCS did not differ in terms of age (W = 131.00, p = 0.453, r = 0.164), LQ (W = 133.50, p = 0.311, r = 0.186), the amount of physical activity (W = 130.00, p = 0.477, r = 0.156) or videogaming (W = 129.50, p = 0.414, r = 0.151) during an average week and the amount of sleep before the experiment (W = 94.50, p = 0.464, r = −0.160) (see Table 1). Furthermore, no difference in attention before the experiment as assessed by d2-R accuracy could be revealed between groups (W = 144.50, p = 0.191, r = 0.284).
Baseline grooved pegboard test performance at PRE
Baseline GPT performance of LHtrained did not differ between group (a-tDCS: 9.6 pegs; s-tDCS: 8.9 pegs; t(28) = 1.134, p = 0.266, d = 0.414). However, for RHuntrained, baseline GPT performance was slightly higher in s-tDCS compared to a-tDCS (a-tDCS: 9.9 pegs, s-tDCS: 11.5 pegs; t(28) = −2.112, p = 0.044, d = −0.771; see Table 2).
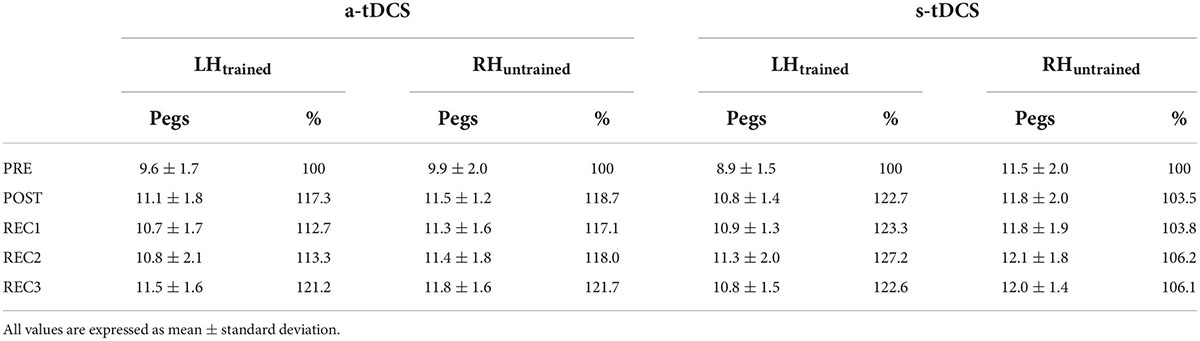
Table 2. Overview of raw Grooved Pegboard Test performance (number of pegs) and percentage improvement (normalized to values obtained at PRE) for both groups (a-tDCS, s-tDCS) for LHtrained and RHuntrained.
Manual dexterity motor performance
With regard to GPT performance of LHtrained, repeated measures ANOVA indicated a significant effect for TRIAL (F(3.525, 95.186) = 9.623, p < 0.001, ηp2 = 0.263). Pairwise post hoc comparisons revealed, that for both groups, GPT performance during LT2 (mean difference (MD) = 16.9%, p < 0.001, d = 1.124), LT3 (MD = 18.5%, p < 0.001, d = 1.231), LT4 (MD = 19.2%, p < 0.001, d = 1.280) and POST (MD = 20.0%, p < 0.001, d = 1.332) was significantly higher compared to baseline performance during PRE (see Figure 2). However, no significant effect for GROUP (F(1, 27) = 0.651, p = 0.318, ηp2 = 0.012) and no significant interaction effect GROUP × TRIAL (F(3.525, 95.186) = 0.240, p = 0.896, ηp2 = 0.009) was observed.
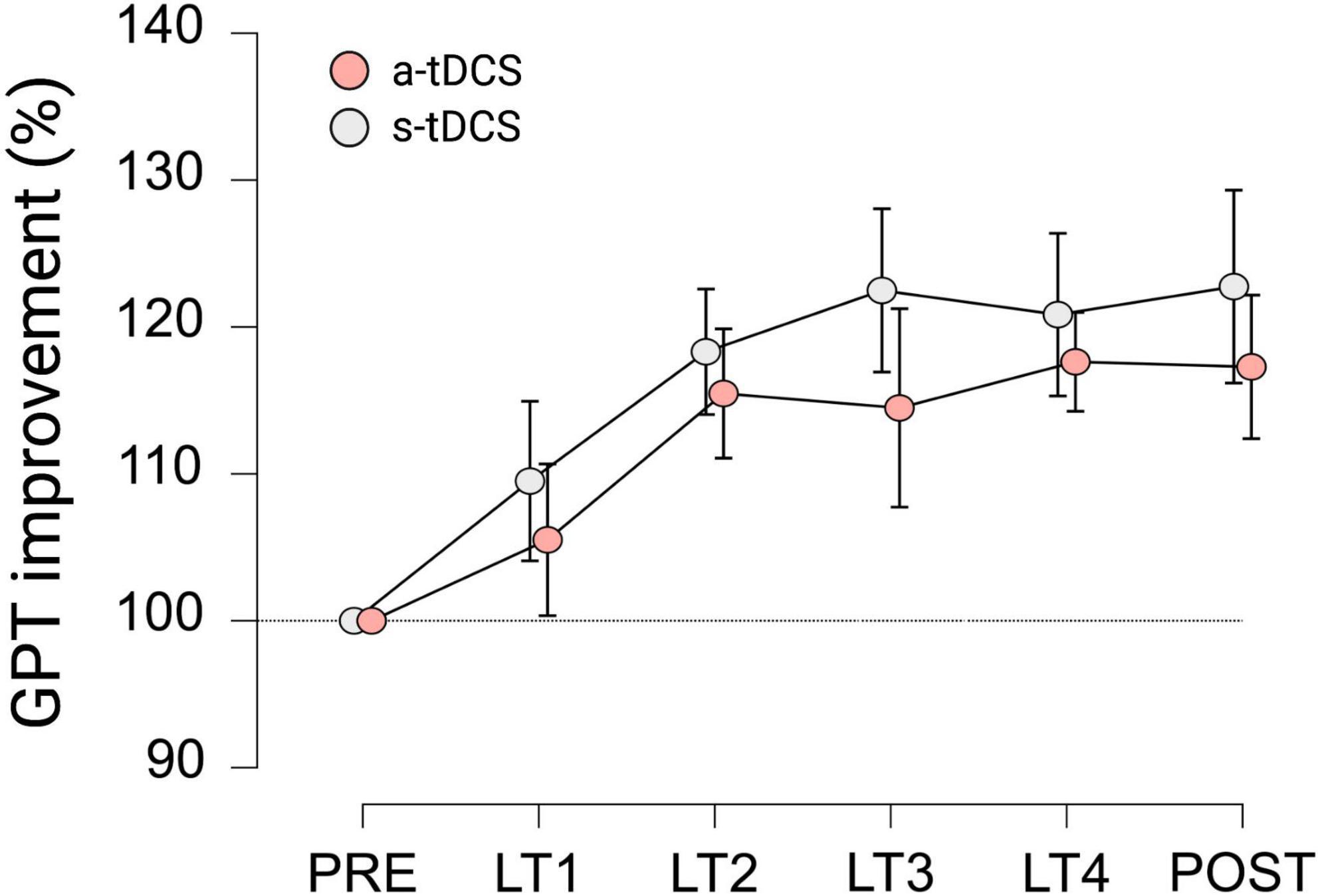
Figure 2. Motor performance of LHtrained during learning trials (LT) of GPT for both groups. All values are normalized to values obtained at PRE, representing percentage improvements. Displayed are mean ± 95% confidence intervals.
Performance during recovery and cross-limb transfer effects
In terms of GPT performance of LHtrained during recovery trials, repeated measures ANOVA revealed a significant effect for TRIAL (F(4, 108) = 10.182, p < 0.001, ηp2 = 0.274). Pairwise post hoc tests indicated that both groups improved GPT performance of LHtrained during POST (MD = 20.0%, p < 0.001, d = 1.247), REC1 (MD = 18.0%, p < 0.003, d = 1.121), REC2 (MD = 20.3%, p < 0.001, d = 1.262), and REC3 (MD = 21.9%, p < 0.001, d = 1.366) compared to baseline performance obtained during PRE (see Figure 3A). However, no significant effect for GROUP (F(1, 27) = 1.487, p = 0.233, ηp2 = 0.052; see Figure 3B) and no significant interaction effect GROUP × TRIAL (F(4, 108) = 1.485, p = 0.212, ηp2 = 0.052) was observed.
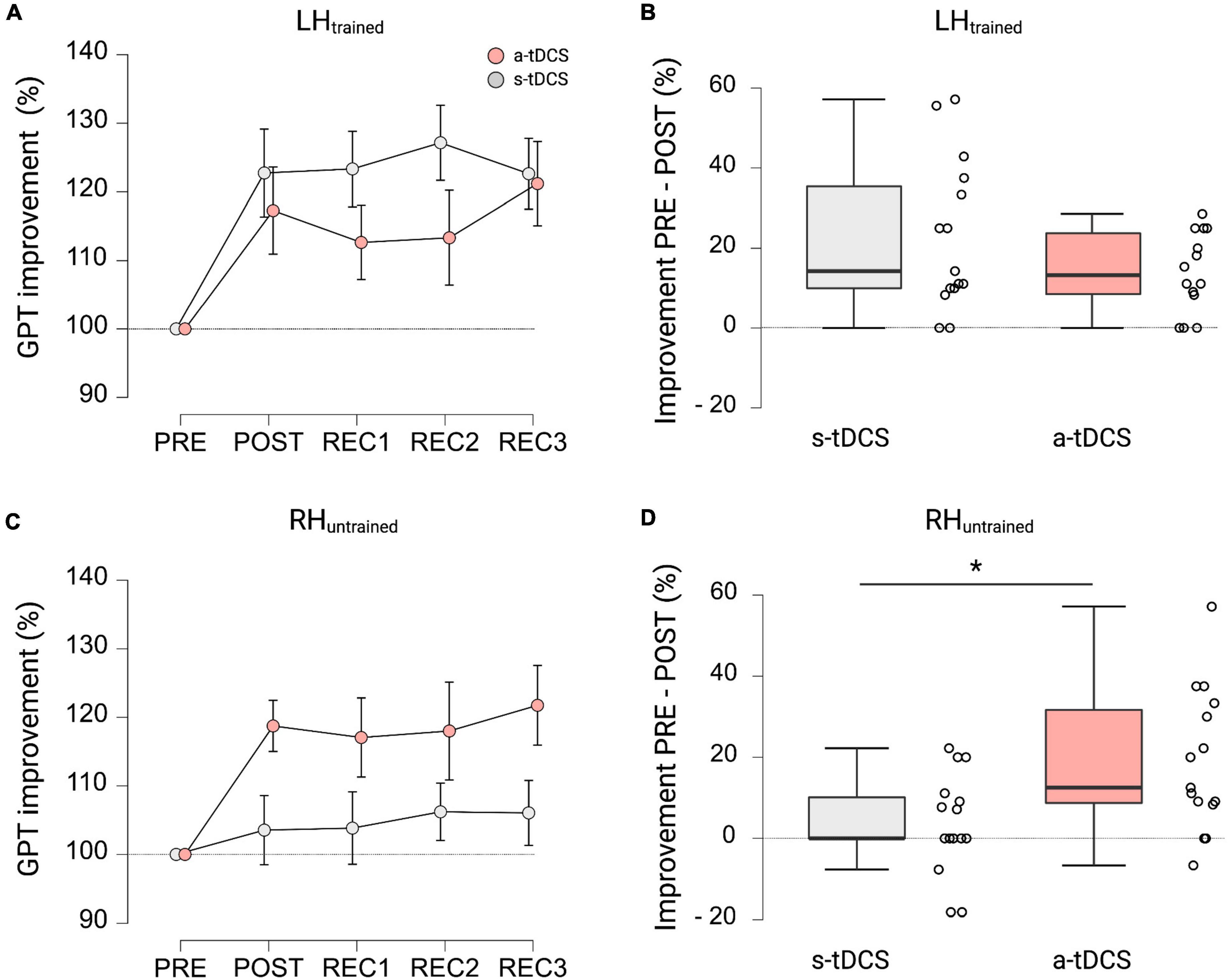
Figure 3. GPT performance during recovery trials and cross-limb transfer for both groups. (A) Motor performance of LHtrained. Displayed are mean ± 95% confidence intervals. All values are normalized to values obtained at PRE, representing percentage improvements. (B) Percentage improvements in GPT of LHtrained from PRE to POST between a-tDCS and s-tDCS. No significant difference in motor performance was observed between groups. (C) Cross-limb transfer of RHuntrained. Displayed are mean ± 95% confidence intervals. All values are normalized to values obtained at PRE, representing percentage improvements. (D) Percentage improvements in GPT of RHuntrained from PRE to POST between a-tDCS and s-tDCS. A-tDCS demonstrated significantly higher cross-limb transfer compared to s-tDCS. *Indicates a significant group difference.
When looking at cross-limb transfer effects to RHuntrained, repeated measures ANOVA revealed a significant effect for GROUP (F(1, 27) = 4.234, p = 0.049, ηp2 = 0.136) and TRIAL (F(2.842, 76.728) = 3.030, p = 0.037, ηp2 = 0.101). Pairwise post hoc comparisons for TRIAL showed that GPT performance of RHuntrained during POST (MD = 11.1%, p = 0.001, d = 0.724), REC1 (MD = 10.5%, p = 0.003, d = 0.679), REC2 (MD = 12.1%, p < 0.001, d = 0.787), and REC3 (MD = 13.9%, p < 0.001, d = 0.903) was significantly higher compared to baseline performance during PRE (see Figure 3C). The post hoc test for GROUP showed, that a-tDCS had higher improvements in GPT performance of RHuntrained and therefore higher cross-limb transfer compared to s-tDCS (MD = 10.6%, p < 0.049, d = 0.376; see Figure 3D). Again, the interaction effect GROUP × TRIAL failed to reach significance (F(2.842, 86.728) = 2.275, p = 0.090, ηp2 = 0.078).
Attention, tiredness, pain (VAS scales)
No significant differences in levels of attention, tiredness, or pain could be detected between VAS ratings before and after stimulation for a-tDCS and s-tDCS (all p > 0.05). Furthermore, no significant between-group differences were observed for any of the VAS scales (all p > 0.05).
Discussion
The main aim of the present study was to investigate whether a-tDCS applied over M1 contralateral to the trained limb is capable of enhancing cross-limb transfer in older adults. Furthermore, the effect of a-tDCS on motor dexterity performance in GPT with the non-dominant LHtrained was investigated. Our results showed that older adults improved GPT performance with their LHtrained and improvements persisted up to 45 min after training. Even more interestingly, older adults also improved RHuntrained performance but only when a-tDCS was applied during left hand training. RHuntrained improvement persisted during recovery measures, thus lasted for at least 45 min after training. After s-tDCS, no RHuntrained improvement was found, showing that cross-limb transfer was presumably mediated by modulation of right M1 excitability.
Our results support previous findings showing that older adults only exhibit cross-limb transfer after tDCS but not after sham stimulation (Goodwill et al., 2015). Our results show that stimulating M1 contralateral to the trained limb is capable of facilitating cross-limb transfer in older adults. This is of particular interest, since cross-limb transfer enhancement was previously mainly associated with increased activation in the hemisphere ipsilateral to the trained limb (Goodwill et al., 2015), hence due to increased bi-hemispheric activation. However, there is no causal relationship between the amount of bilateral activation and the amount of cross-limb transfer (Hinder et al., 2011) and other studies found divergent results after stimulating M1 ipsilateral to the trained limb (Kobayashi et al., 2009; Keitel et al., 2018). In our study, activation in the trained hemisphere was upregulated by a-tDCS, presumably resulting in reduced activation in the other (Tazoe et al., 2014) since M1-tDCS was shown to modulate remote interconnected neuronal networks apart from local M1 modulations in excitation and/or inhibition (Boros et al., 2008; Polania et al., 2011). Thus, alterations in interhemispheric communication can be regarded as one candidate mechanism responsible for cross-limb transfer improvements. There are different theories on how communication between hemispheres arises during unimanual motor tasks. While according to the cross activation hypothesis, bilateral activation induced by unimanual training evokes adaptations on both hemispheres, the bilateral access hypothesis states that information are resided at a central site other than the involved cortices (such as premotor cortex or supplementary motor area) where information can be accessed also from the untrained hemisphere (M. Lee et al., 2010). Our results are not exclusively in favor of one of the theories but do support the assumption that facilitated access to M1 contralateral to the trained limb enhances cross-limb transfer, suggesting a bilateral access either from the untrained M1 itself or from areas upstream of M1 (Boros et al., 2008).
It has been shown that dominant hand practice of GPT results in improved GPT performance of the non-dominant hand (Beg et al., 2021), speaking in favor of cross-limb transfer of the manual dexterity motor skill. The results of the current study expand these findings by showing that non-dominant hand training of GPT does not facilitate dominant hand performance in older adults receiving s-tDCS, suggesting that no skill transfer to the untrained hand took place. This result is in line with previous findings, showing that non-dominant hand performance improvements in most cases do not transfer to the dominant hand (Teixeira, 2000; Hinder et al., 2011). Differences between dominant and non-dominant hand transfer have mainly been attributed to more accurate internal models created by dominant hand training and better accessibility of the left (dominant) hemisphere (Wang and Sainburg, 2004; Pan and Van Gemmert, 2013). This explanation is also in line with our results where upregulation of the non-dominant right hemisphere by means of a-tDCS facilitated cross-limb transfer. In contrast to other studies (Kidgell et al., 2013; Marquez et al., 2015), a-tDCS did not facilitate left hand GPT learning. This finding was surprising since we expected tDCS over the right M1 to facilitate motor practice of the left hand especially because stimulation parameters comparable with other studies such as 20 min of stimulation duration, 1 mA current intensity and also similar electrode positions were used (Hummel et al., 2010; Marquez et al., 2015; Patel et al., 2019). However, GPT training only included four 30-second trials of left hand practice, thus may have been too short for the induction of differential tDCS effects. It might be, that longer training periods with a higher number of trials are necessary to observe differential GPT performance effects induced by tDCS. Besides, tDCS effects are highly variable across as well as within subjects and may be influenced by many different factors like age, sex, time of the day, quality of sleep or physical activity (Ridding and Ziemann, 2010; Polania et al., 2018). However, since a tDCS-induced effect on cross-limb transfer was found, we assume that most participants have responded to the stimulation. Interestingly, the missing tDCS effect on GPT performance of LHtrained suggests, that it is not the magnitude of training-induced improvement per se that is transferred via cross-limb transfer. Thus, a linear relationship between motor performance and cross-limb transfer is not necessarily evident (Goodwill et al., 2015) and both potentially evolve from different neural mechanisms. As postulated earlier, better accessibility of the non-dominant M1 induced by tDCS might be held responsible for facilitated cross-limb transfer, speaking in favor of the bilateral access hypothesis (Boros et al., 2008). Facilitation of GPT performance in trained limbs by means of tDCS, however, might need a sufficient time of concurrent M1-activation via both stimulation and training (Reis et al., 2008), which in our setup was potentially too short. Remarkably, we found that GPT performance improvement of the right hand induced by cross-limb skill transfer was comparable to GPT performance improvement of the left hand induced by training (13–20% skill improvement, see Table 2 for details). Transferred to absolute values, this means an improvement of around 1.5 pegs in 30 s, which seems like a small change. However, we never the less argue that this improvement is meaningful. Normative data states, that mean peg rate decreases by about 1 peg per decade (Agnew et al., 1988; Desrosiers et al., 1995). Given that this small but steady linear decrease is accompanied by major decreases in general cognitive functioning (Ashendorf et al., 2009), it is reasonable to assume that a 1.5 pegs improvement is relevant for daily living. This is also supported by a study showing that a difference of 1–2 pegs has high predictive value for a subsequent Parkinson’s diagnosis (Adler et al., 2002). Thus, one can conclude that cross-limb transfer may be a powerful way to improve performance in the opposite limb even in the absence of active practice. This may be of particular importance in the context of rehabilitation, where in some cases active training is impossible. One example could be either disease-related functional losses of hand motor function for example after stroke or Parkinson but also if active hand training is limited through limb pain or immobilization. Also, for healthy old adults, maximizing cross-limb transfer effects by using neuromodulatory tDCS could be important for example to align asymmetrical fine motor abilities.
The current study faces some limitations. Since only behavioral data was assessed and no control region was stimulated by means of a-tDCS, we can only speculate that the current findings were related to altered intra-/and/or interhemispheric communication. Furthermore, it would be of great importance to include also other stimulation sites such as the premotor cortex since it is known to be heavily involved in motor preparation and performance (Boros et al., 2008; Pavlova et al., 2022) and investigate potential mediatory effects on magnitude but also timing of transfer. Moreover, we found baseline differences between groups showing higher baseline GPT performance in the right hand in the sham group. However, all data was normalized to this first baseline timepoint and subsequent analyses were only performed using this normalized data. Furthermore, given that normative data shows, that older adults in the age range of our participants (60–69 years) are able to plug 13.9/13.1 pegs with their non-dominant hand in 30 s (Agnew et al., 1988; Desrosiers et al., 1995), we believe that possible ceiling effects of performance improvement did not affect our results. We are confident, that even though these baseline differences exist at least in one hand, performance improvements can nonetheless be interpreted. Furthermore, our groups contained an uneven number of male and female participants (s-tDCS: 10 females, a-tDCS: 5 females). Given that there is evidence for a strong effect of sex on simulated current intensity at older ages (Bhattacharjee et al., 2022), one could speculate that tDCS application created different brain activation patterns in our male and female participants. We addressed this issue by adding sex as a covariate to our statistical model, however, possible sex differences regarding responsiveness to tDCS may still have influenced our results.
Taken together, our study shows that tDCS over right M1 is capable of facilitating cross-limb transfer from left to right hand in older adults. Remarkably, the amount of cross-limb transfer induced by tDCS was comparable to the amount of skill learning induced by active training. This finding may specifically important in the context of neurorehabilitation, where training regimes other than active training are necessary to improve motor dexterity function.
Data availability statement
The raw data supporting the conclusions of this article will be made available by the authors, without undue reservation.
Ethics statement
The studies involving human participants were reviewed and approved by the Local Ethics Committee of Leipzig University (ref. nr. 202-13-150-72013), Stephanstraße 9a, 04103 Leipzig. The patients/participants provided their written informed consent to participate in this study.
Author contributions
PB and PR designed the study. PB and MO collected the data. TM analyzed the data and created the figures. TM and EK drafted the manuscript. PB, MO, AV, and PR provided critical revision. All authors approved the final version of the manuscript and agreed to be accountable for all aspects of the work in ensuring that questions related to the accuracy or integrity of any part of the work are appropriately investigated and resolved.
Funding
This study was supported by the Max-Planck-Society.
Acknowledgments
We thank Ramona Menger and Bettina Johst for organizational and technical support.
Conflict of interest
The authors declare that the research was conducted in the absence of any commercial or financial relationships that could be construed as a potential conflict of interest.
Publisher’s note
All claims expressed in this article are solely those of the authors and do not necessarily represent those of their affiliated organizations, or those of the publisher, the editors and the reviewers. Any product that may be evaluated in this article, or claim that may be made by its manufacturer, is not guaranteed or endorsed by the publisher.
References
Adler, C. H., Hentz, J. G., Joyce, J. N., Beach, T., and Caviness, J. N. (2002). Motor impairment in normal aging, clinically possible Parkinson’s disease, and clinically probable Parkinson’s disease: Longitudinal evaluation of a cohort of prospective brain donors. Parkinsonism Relat. Disord 9, 103–110. doi: 10.1016/s1353-8020(02)00012-3
Agnew, J., Bollawilson, K., Kawas, C. H., and Bleecker, M. L. (1988). Purdue pegboard age and sex norms for people 40 Years old and older. Dev. Neuropsychol. 4, 29–35. doi: 10.1080/87565648809540388
Ammann, C., Spampinato, D., and Marquez-Ruiz, J. (2016). Modulating motor learning through transcranial direct-current stimulation: An integrative view. Front. Psychol. 7:1981. doi: 10.3389/fpsyg.2016.01981
Ashendorf, L., Vanderslice-Barr, J. L., and McCaffrey, R. J. (2009). Motor tests and cognition in healthy older adults. Appl. Neuropsychol. 16, 171–176. doi: 10.1080/09084280903098562
Beg, R. A., Shaphe, M. A., Qasheesh, M., Ahmad, F., Anwer, S., and Alghadir, A. H. (2021). Intermanual transfer effects on performance gain following dominant hand training in community-dwelling healthy adults: A preliminary study. J. Multidiscip. Healthc. 14, 1007–1016. doi: 10.2147/JMDH.S298991
Bhattacharjee, S., Kashyap, R., Goodwill, A. M., O’Brien, B. A., Rapp, B., Oishi, K., et al. (2022). Sex difference in tDCS current mediated by changes in cortical anatomy: A study across young, middle and older adults. Brain Stimul. 15, 125–140. doi: 10.1016/j.brs.2021.11.018
Bodwell, J. A., Mahurin, R. K., Waddle, S., Price, R., and Cramer, S. C. (2003). Age and features of movement influence motor overflow. J. Am. Geriatr. Soc. 51, 1735–1739. doi: 10.1046/j.1532-5415.2003.51557.x
Boggio, P. S., Castro, L. O., Savagim, E. A., Braite, R., Cruz, V. C., Rocha, R. R., et al. (2006). Enhancement of non-dominant hand motor function by anodal transcranial direct current stimulation. Neurosci. Lett. 404, 232–236. doi: 10.1016/j.neulet.2006.05.051
Borchers, S., Himmelbach, M., Logothetis, N., and Karnath, H. O. (2011). Direct electrical stimulation of human cortex - the gold standard for mapping brain functions? Nat. Rev. Neurosci. 13, 63–70. doi: 10.1038/nrn3140
Boros, K., Poreisz, C., Munchau, A., Paulus, W., and Nitsche, M. A. (2008). Premotor transcranial direct current stimulation (tDCS) affects primary motor excitability in humans. Eur. J. Neurosci. 27, 1292–1300. doi: 10.1111/j.1460-9568.2008.06090.x
Brickenkamp, R., Schmidt-Atzert, L., and Liepmann, D. (2010). Test d2-Revision: Aufmerksamkeits-und Konzentrationstest. Göttingen: Hogrefe.
Buch, E. R., Santarnecchi, E., Antal, A., Born, J., Celnik, P. A., Classen, J., et al. (2017). Effects of tDCS on motor learning and memory formation: A consensus and critical position paper. Clin. Neurophysiol. 128, 589–603. doi: 10.1016/j.clinph.2017.01.004
Cabeza, R. (2002). Hemispheric asymmetry reduction in older adults: The HAROLD model. Psychol. Aging 17, 85–100. doi: 10.1037//0882-7974.17.1.85
Cabeza, R., Albert, M., Belleville, S., Craik, F. I. M., Duarte, A., Grady, C. L., et al. (2018). Maintenance, reserve and compensation: The cognitive neuroscience of healthy ageing. Nat. Rev. Neurosci. 19, 701–710. doi: 10.1038/s41583-018-0068-2
Carroll, T. J., Lee, M., Hsu, M., and Sayde, J. (2008). Unilateral practice of a ballistic movement causes bilateral increases in performance and corticospinal excitability. J. Appl. Physiol (1985) 104, 1656–1664. doi: 10.1152/japplphysiol.01351.2007
Cassady, K., Ruitenberg, M. F. L., Reuter-Lorenz, P. A., Tommerdahl, M., and Seidler, R. D. (2020). Neural dedifferentiation across the lifespan in the motor and somatosensory systems. Cereb. Cortex 30, 3704–3716. doi: 10.1093/cercor/bhz336
Choi, Y. I., Song, C. S., and Chun, B. Y. (2017). Activities of daily living and manual hand dexterity in persons with idiopathic Parkinson disease. J. Phys. Ther. Sci. 29, 457–460. doi: 10.1589/jpts.29.457
Cirillo, J. (2021). Physical activity, motor performance and skill learning: A focus on primary motor cortex in healthy aging. Exp. Brain Res. 239, 3431–3438. doi: 10.1007/s00221-021-06218-1
Cole, K. J., Rotella, D. L., and Harper, J. G. (1999). Mechanisms for age-related changes of fingertip forces during precision gripping and lifting in adults. J. Neurosci. 19, 3238–3247. doi: 10.1523/JNEUROSCI.19-08-03238.1999
Convento, S., Bolognini, N., Fusaro, M., Lollo, F., and Vallar, G. (2014). Neuromodulation of parietal and motor activity affects motor planning and execution. Cortex 57, 51–59. doi: 10.1016/j.cortex.2014.03.006
Cramer, S. C., Finklestein, S. P., Schaechter, J. D., Bush, G., and Rosen, B. R. (1999). Activation of distinct motor cortex regions during ipsilateral and contralateral finger movements. J. Neurophysiol. 81, 383–387. doi: 10.1152/jn.1999.81.1.383
Desrosiers, J., Hebert, R., Bravo, G., and Dutil, E. (1995). The purdue pegboard test: Normative data for people aged 60 and over. Disabil. Rehabil. 17, 217–224. doi: 10.3109/09638289509166638
Friedman, N., Chan, V., Zondervan, D., Bachman, M., and Reinkensmeyer, D. J. (2011). MusicGlove: Motivating and quantifying hand movement rehabilitation by using functional grips to play music. Annu. Int. Conf. IEEE Eng. Med. Biol. Soc. 2011, 2359–2363. doi: 10.1109/IEMBS.2011.6090659
Goble, D. J., Coxon, J. P., Wenderoth, N., Van Impe, A., and Swinnen, S. P. (2009). Proprioceptive sensibility in the elderly: Degeneration, functional consequences and plastic-adaptive processes. Neurosci. Biobehav. Rev. 33, 271–278. doi: 10.1016/j.neubiorev.2008.08.012
Goodwill, A. M., Daly, R. M., and Kidgell, D. J. (2015). The effects of anodal-tDCS on cross-limb transfer in older adults. Clin. Neurophysiol. 126, 2189–2197. doi: 10.1016/j.clinph.2015.01.006
Goodwill, A. M., Reynolds, J., Daly, R. M., and Kidgell, D. J. (2013). Formation of cortical plasticity in older adults following tDCS and motor training. Front. Aging Neurosci. 5:87. doi: 10.3389/fnagi.2013.00087
Guttmann, C. R., Jolesz, F. A., Kikinis, R., Killiany, R. J., Moss, M. B., Sandor, T., et al. (1998). White matter changes with normal aging. Neurology 50, 972–978. doi: 10.1212/wnl.50.4.972
Hinder, M. R., Schmidt, M. W., Garry, M. I., Carroll, T. J., and Summers, J. J. (2011). Absence of cross-limb transfer of performance gains following ballistic motor practice in older adults. J. Appl. Physiol (1985) 110, 166–175. doi: 10.1152/japplphysiol.00958.2010
Hoogendam, Y. Y., van der Lijn, F., Vernooij, M. W., Hofman, A., Niessen, W. J., van der Lugt, A., et al. (2014). Older age relates to worsening of fine motor skills: A population-based study of middle-aged and elderly persons. Front. Aging Neurosci. 6:259. doi: 10.3389/fnagi.2014.00259
Hummel, F. C., Heise, K., Celnik, P., Floel, A., Gerloff, C., and Cohen, L. G. (2010). Facilitating skilled right hand motor function in older subjects by anodal polarization over the left primary motor cortex. Neurobiol. Aging 31, 2160–2168. doi: 10.1016/j.neurobiolaging.2008.12.008
Hunter, S. K., Pereira, H. M., and Keenan, K. G. (2016). The aging neuromuscular system and motor performance. J. Appl. Physiol (1985) 121, 982–995. doi: 10.1152/japplphysiol.00475.2016
Iyo, M., and Yamasaki, T. (1993). The detection of age-related decrease of dopamine D1, D2 and serotonin 5-HT2 receptors in living human brain. Prog. Neuropsychopharmacol. Biol. Psychiatry 17, 415–421. doi: 10.1016/0278-5846(93)90075-4
Jacobson, L., Koslowsky, M., and Lavidor, M. (2012). tDCS polarity effects in motor and cognitive domains: A meta-analytical review. Exp. Brain Res. 216, 1–10. doi: 10.1007/s00221-011-2891-9
Kaminski, E., Hoff, M., Sehm, B., Taubert, M., Conde, V., Steele, C. J., et al. (2013). Effect of transcranial direct current stimulation (tDCS) during complex whole body motor skill learning. Neurosci. Lett. 552, 76–80. doi: 10.1016/j.neulet.2013.07.034
Karok, S., and Witney, A. G. (2013). Enhanced motor learning following task-concurrent dual transcranial direct current stimulation. PLoS One 8:e85693. doi: 10.1371/journal.pone.0085693
Keitel, A., Ofsteng, H., Krause, V., and Pollok, B. (2018). Anodal transcranial direct current stimulation (tDCS) over the right primary motor cortex (M1) impairs implicit motor sequence learning of the ipsilateral hand. Front. Hum. Neurosci. 12:289. doi: 10.3389/fnhum.2018.00289
Kidgell, D. J., Goodwill, A. M., Frazer, A. K., and Daly, R. M. (2013). Induction of cortical plasticity and improved motor performance following unilateral and bilateral transcranial direct current stimulation of the primary motor cortex. BMC Neurosci. 14:64. doi: 10.1186/1471-2202-14-64
Knights, E., Morcom, A. M., and Henson, R. N. (2021). Does hemispheric asymmetry reduction in older adults in motor cortex reflect compensation? J. Neurosci. 41, 9361–9373. doi: 10.1523/JNEUROSCI.1111-21.2021
Kobayashi, M., Theoret, H., and Pascual-Leone, A. (2009). Suppression of ipsilateral motor cortex facilitates motor skill learning. Eur. J. Neurosci. 29, 833–836. doi: 10.1111/j.1460-9568.2009.06628.x
Koeneke, S., Battista, C., Jancke, L., and Peters, M. (2009). Transfer effects of practice for simple alternating movements. J. Mot. Behav. 41, 347–355. doi: 10.3200/JMBR.41.4.347-356
Koeneke, S., Lutz, K., Herwig, U., Ziemann, U., and Jancke, L. (2006). Extensive training of elementary finger tapping movements changes the pattern of motor cortex excitability. Exp. Brain Res. 174, 199–209. doi: 10.1007/s00221-006-0440-8
Koo, H., Kim, M. S., Han, S. W., Paulus, W., Nitche, M. A., Kim, Y. H., et al. (2016). After-effects of anodal transcranial direct current stimulation on the excitability of the motor cortex in rats. Restor. Neurol. Neurosci. 34, 859–868. doi: 10.3233/RNN-160664
Kunze, T., Hunold, A., Haueisen, J., Jirsa, V., and Spiegler, A. (2016). Transcranial direct current stimulation changes resting state functional connectivity: A large-scale brain network modeling study. Neuroimage 140, 174–187. doi: 10.1016/j.neuroimage.2016.02.015
Lee, L., Siebner, H. R., Rowe, J. B., Rizzo, V., Rothwell, J. C., Frackowiak, R. S. J., et al. (2003). Acute remapping within the motor system induced by low-frequency repetitive transcranial magnetic stimulation. J. Neurosci. 23, 5308–5318.
Lee, M., Hinder, M. R., Gandevia, S. C., and Carroll, T. J. (2010). The ipsilateral motor cortex contributes to cross-limb transfer of performance gains after ballistic motor practice. J. Physiol. 588(Pt 1), 201–212. doi: 10.1113/jphysiol.2009.183855
Makofske, B. (2011). “Manual dexterity,” in Encyclopedia of clinical neuropsychology, eds J. S. Kreutzer, J. DeLuca, and B. Caplan (New York, NY: Springer New York), 1522–1523.
Mancini, M., Brignani, D., Conforto, S., Mauri, P., Miniussi, C., and Pellicciari, M. C. (2016). Assessing cortical synchronization during transcranial direct current stimulation: A graph-theoretical analysis. Neuroimage 140, 57–65. doi: 10.1016/j.neuroimage.2016.06.003
Marquez, J., Conley, A., Karayanidis, F., Lagopoulos, J., and Parsons, M. (2015). Anodal direct current stimulation in the healthy aged: Effects determined by the hemisphere stimulated. Restor. Neurol. Neurosci. 33, 509–519. doi: 10.3233/RNN-140490
Nitsche, M. A., and Paulus, W. (2000). Excitability changes induced in the human motor cortex by weak transcranial direct current stimulation. J. Physiol. 527(Pt 3), 633–639. doi: 10.1111/j.1469-7793.2000.t01-1-00633.x
Nitsche, M. A., Cohen, L. G., Wassermann, E. M., Priori, A., Lang, N., Antal, A., et al. (2008). Transcranial direct current stimulation: State of the art 2008. Brain Stimul. 1, 206–223. doi: 10.1016/j.brs.2008.06.004
Oldfield, R. C. (1971). The assessment and analysis of handedness: The Edinburgh inventory. Neuropsychologia 9, 97–113. doi: 10.1016/0028-3932(71)90067-4
Pan, Z., and Van Gemmert, A. W. (2013). The effects of aging on the asymmetry of inter-limb transfer in a visuomotor task. Exp. Brain Res. 229, 621–633. doi: 10.1007/s00221-013-3625-y
Pantoni, L. (2010). Cerebral small vessel disease: From pathogenesis and clinical characteristics to therapeutic challenges. Lancet Neurol. 9, 689–701. doi: 10.1016/S1474-4422(10)70104-6
Parikh, P. J., and Cole, K. J. (2014). Effects of transcranial direct current stimulation in combination with motor practice on dexterous grasping and manipulation in healthy older adults. Physiol. Rep. 2:e00255. doi: 10.1002/phy2.255
Patel, R., Ashcroft, J., Patel, A., Ashrafian, H., Woods, A. J., Singh, H., et al. (2019). the impact of transcranial direct current stimulation on upper-limb motor performance in healthy adults: A systematic review and meta-analysis. Front. Neurosci. 13:1213. doi: 10.3389/fnins.2019.01213
Pavlova, E. L., Semenov, R. V., Pavlova-Deb, M. P., and Guekht, A. B. (2022). Transcranial direct current stimulation of the premotor cortex aimed to improve hand motor function in chronic stroke patients. Brain Res. 178:147790. doi: 10.1016/j.brainres.2022.147790
Polania, R., Nitsche, M. A., and Paulus, W. (2011). Modulating functional connectivity patterns and topological functional organization of the human brain with transcranial direct current stimulation. Hum. Brain Mapp. 32, 1236–1249. doi: 10.1002/hbm.21104
Polania, R., Nitsche, M. A., and Ruff, C. C. (2018). Studying and modifying brain function with non-invasive brain stimulation. Nat. Neurosci. 21, 174–187. doi: 10.1038/s41593-017-0054-4
Ranganathan, V. K., Siemionow, V., Sahgal, V., and Yue, G. H. (2001a). Effects of aging on hand function. J. Am. Geriatr. Soc. 49, 1478–1484. doi: 10.1046/j.1532-5415.2001.4911240.x
Ranganathan, V. K., Siemionow, V., Sahgal, V., Liu, J. Z., and Yue, G. H. (2001b). Skilled finger movement exercise improves hand function. J. Gerontol. A Biol. Sci. Med. Sci. 56, M518–M522. doi: 10.1093/gerona/56.8.m518
Reis, J., and Fritsch, B. (2011). Modulation of motor performance and motor learning by transcranial direct current stimulation. Curr. Opin. Neurol. 24, 590–596. doi: 10.1097/WCO.0b013e32834c3db0
Reis, J., Robertson, E., Krakauer, J. W., Rothwell, J., Marshall, L., Gerloff, C., et al. (2008). Consensus: “Can tDCS and TMS enhance motor learning and memory formation?”. Brain Stimul. 1, 363–369. doi: 10.1016/j.brs.2008.08.001
Ridding, M. C., and Ziemann, U. (2010). Determinants of the induction of cortical plasticity by non-invasive brain stimulation in healthy subjects. J. Physiol. 588(Pt 13), 2291–2304. doi: 10.1113/jphysiol.2010.190314
Roig, M., Ritterband-Rosenbaum, A., Lundbye-Jensen, J., and Nielsen, J. B. (2014). Aging increases the susceptibility to motor memory interference and reduces off-line gains in motor skill learning. Neurobiol. Aging 35, 1892–1900. doi: 10.1016/j.neurobiolaging.2014.02.022
Sainburg, R. L., and Wang, J. (2002). Interlimb transfer of visuomotor rotations: Independence of direction and final position information. Exp. Brain Res. 145, 437–447. doi: 10.1007/s00221-002-1140-7
Schaefer, S. Y., Haaland, K. Y., and Sainburg, R. L. (2009). Hemispheric specialization and functional impact of ipsilesional deficits in movement coordination and accuracy. Neuropsychologia 47, 2953–2966. doi: 10.1016/j.neuropsychologia.2009.06.025
Sears, E. D., and Chung, K. C. (2010). Validity and responsiveness of the jebsen-taylor hand function test. J. Hand. Surg. Am. 35, 30–37. doi: 10.1016/j.jhsa.2009.09.008
Seidler, R. D., Bernard, J. A., Burutolu, T. B., Fling, B. W., Gordon, M. T., Gwin, J. T., et al. (2010). Motor control and aging: Links to age-related brain structural, functional, and biochemical effects. Neurosci. Biobehav. Rev. 34, 721–733. doi: 10.1016/j.neubiorev.2009.10.005
Smith, C. D., Umberger, G. H., Manning, E. L., Slevin, J. T., Wekstein, D. R., Schmitt, F. A., et al. (1999). Critical decline in fine motor hand movements in human aging. Neurology 53, 1458–1458. doi: 10.1212/wnl.53.7.1458
Sohn, M. K., Kim, B. O., and Song, H. T. (2012). Effect of stimulation polarity of transcranial direct current stimulation on non-dominant hand function. Ann. Rehabil. Med. 36, 1–7. doi: 10.5535/arm.2012.36.1.1
Sowell, E. R., Peterson, B. S., Thompson, P. M., Welcome, S. E., Henkenius, A. L., and Toga, A. W. (2003). Mapping cortical change across the human life span. Nat. Neurosci. 6, 309–315. doi: 10.1038/nn1008
Stockel, T., and Wang, J. (2011). Transfer of short-term motor learning across the lower limbs as a function of task conception and practice order. Brain Cogn. 77, 271–279. doi: 10.1016/j.bandc.2011.07.010
Summers, J. J., Kang, N., and Cauraugh, J. H. (2016). Does transcranial direct current stimulation enhance cognitive and motor functions in the ageing brain? A systematic review and meta- analysis. Ageing Res. Rev. 25, 42–54. doi: 10.1016/j.arr.2015.11.004
Tazoe, T., Endoh, T., Kitamura, T., and Ogata, T. (2014). Polarity specific effects of transcranial direct current stimulation on interhemispheric inhibition. PLoS One 9:e114244. doi: 10.1371/journal.pone.0114244
Teixeira, L. A. (2000). Timing and force components in bilateral transfer of learning. Brain Cogn. 44, 455–469. doi: 10.1006/brcg.1999.1205
van Mier, H. I., and Petersen, S. E. (2006). Intermanual transfer effects in sequential tactuomotor learning: Evidence for effector independent coding. Neuropsychologia 44, 939–949. doi: 10.1016/j.neuropsychologia.2005.08.010
VanSwearingen, J. M., and Studenski, S. A. (2014). Aging, motor skill, and the energy cost of walking: Implications for the prevention and treatment of mobility decline in older persons. J. Gerontol. A Biol. Sci. Med. Sci. 69, 1429–1436. doi: 10.1093/gerona/glu153
Voelcker-Rehage, C. (2008). Motor-skill learning in older adults - a review of studies on age-related differences. Eur. Rev. Aging Phys. Act. 5, 5–16. doi: 10.1007/s11556-008-0030-9
Wang, J., and Sainburg, R. L. (2004). Interlimb transfer of novel inertial dynamics is asymmetrical. J. Neurophysiol. 92, 349–360. doi: 10.1152/jn.00960.2003
Zapparoli, L., Mariano, M., and Paulesu, E. (2022). How the motor system copes with aging: A quantitative meta-analysis of the effect of aging on motor function control. Commun. Biol. 5:79. doi: 10.1038/s42003-022-03027-2
Zimerman, M., and Hummel, F. C. (2010). Non-invasive brain stimulation: Enhancing motor and cognitive functions in healthy old subjects. Front. Aging Neurosci. 2:149. doi: 10.3389/fnagi.2010.00149
Keywords: manual dexterity, grooved pegboard task, transcranial direct current stimulation, aging, cross-limb transfer
Citation: Kaminski E, Maudrich T, Bassler P, Ordnung M, Villringer A and Ragert P (2022) tDCS over the primary motor cortex contralateral to the trained hand enhances cross-limb transfer in older adults. Front. Aging Neurosci. 14:935781. doi: 10.3389/fnagi.2022.935781
Received: 04 May 2022; Accepted: 29 August 2022;
Published: 20 September 2022.
Edited by:
Hakuei Fujiyama, Murdoch University, AustraliaReviewed by:
Eduardo Lattari, Federal University of Rio de Janeiro, BrazilAlicia M. Goodwill, Nanyang Technological University, Singapore
Copyright © 2022 Kaminski, Maudrich, Bassler, Ordnung, Villringer and Ragert. This is an open-access article distributed under the terms of the Creative Commons Attribution License (CC BY). The use, distribution or reproduction in other forums is permitted, provided the original author(s) and the copyright owner(s) are credited and that the original publication in this journal is cited, in accordance with accepted academic practice. No use, distribution or reproduction is permitted which does not comply with these terms.
*Correspondence: Elisabeth Kaminski, Elisabeth.kaminski@uni-leipzig.de
†These authors have contributed equally to this work