Targeting DNA-Protein Crosslinks via Post-Translational Modifications
- The Novo Nordisk Foundation Center for Protein Research, Faculty of Health and Medical Sciences, University of Copenhagen, Copenhagen, Denmark
Covalent binding of proteins to DNA forms DNA-protein crosslinks (DPCs), which represent cytotoxic DNA lesions that interfere with essential processes such as DNA replication and transcription. Cells possess different enzymatic activities to counteract DPCs. These include enzymes that degrade the adducted proteins, resolve the crosslinks, or incise the DNA to remove the crosslinked proteins. An important question is how DPCs are sensed and targeted for removal via the most suited pathway. Recent advances have shown the inherent role of DNA replication in triggering DPC removal by proteolysis. However, DPCs are also efficiently sensed and removed in the absence of DNA replication. In either scenario, post-translational modifications (PTMs) on DPCs play essential and versatile roles in orchestrating the repair routes. In this review, we summarize the current knowledge of the mechanisms that trigger DPC removal via PTMs, focusing on ubiquitylation, small ubiquitin-related modifier (SUMO) conjugation (SUMOylation), and poly (ADP-ribosyl)ation (PARylation). We also briefly discuss the current knowledge gaps and emerging hypotheses in the field.
Introduction
DNA-interacting proteins become crosslinked to DNA by endogenous or exogenous sources including reactive chemicals (e.g., aldehydes), physical agents (e.g., ultraviolet (UV) light and ionizing radiation (IR)), chemotherapeutics (e.g., topoisomerase poisons and cisplatin-based compounds), and DNA damages (e.g., abasic sites). These lesions are highly diverse in the nature and size of the crosslinked protein, the chemical properties of the covalent linkage, and the structure of the linked DNA. DPCs are commonly classified as enzymatic DPCs, for DNA acting enzymes that remain stalled as covalent intermediates during their catalytic cycles, and non-enzymatic DPCs, for proteins that become linked to DNA via crosslinking agents (Stingele and Jentsch, 2015).
Non-enzymatic DPCs are generated in cells by crosslinking agents such as reactive aldehydes. Formaldehyde, the byproduct of histone demethylation or lipid peroxidation, covalently links proteins to DNA through a methylene bridge (Esterbauer et al., 1982; Shi et al., 2004; Lu et al., 2010). Formaldehyde is abundant in human blood and might be the major DPC inducer in cells (Heck et al., 1985; Luo et al., 2001; Nakamura and Nakamura, 2020). Highlighting the physiological relevance of formaldehyde, recent evidence in mice suggests that formaldehyde drives the phenotype of Cockayne syndrome, a disease caused by dysfunctional transcription-coupled nucleotide excision repair (TC-NER), suggesting that TC-NER may play a central role in removing formaldehyde-induced DPCs and/or other DNA lesions (e.g., DNA intra- or inter-strand crosslinks) (Mulderrig et al., 2021). Non-enzymatic DPCs can also form between nucleophilic amino acids of proteins and the open chain conformation of native apurinic/apyrimidinic (AP) sites or with the repair intermediates of certain DNA base modifications (e.g., N7-methyl-dG, N3-methyl-dA, oxidized DNA 8-oxo-dG, or modified DNA base 5-formylcytosine) (Li et al., 2017; Bai et al., 2018; Yang et al., 2018; Yang et al., 2019). Exogenous DNA damaging agents such as UV light, IR, and chemotherapeutics such as cisplatin-based compounds, can also crosslink proteins to DNA (Chodosh, 2001; Barker et al., 2005; Chvalova et al., 2007). Despite the abundance of crosslinking agents present in cells, the biological relevance of non-enzymatic DPCs is largely undetermined. This is because the protein identities and chemical properties of non-enzymatic DPCs remain poorly defined despite recent advances in mass spectrometry techniques that can quantify and monitor these DPCs in cells (Tretyakova et al., 2015; Groehler et al., 2017; Liu et al., 2018; Tayri-Wilk et al., 2020). Moreover, these agents also generate other predominant DNA lesions (e.g., DNA breaks and DNA-DNA crosslinks), making it difficult to assess the impact of non-enzymatic DPCs on cellular sensitivity and the DNA damage response using these pleiotropic crosslinkers.
In contrast to non-enzymatic DPCs, enzymatic DPCs can be induced to generate specific lesions in cells. For example, the catalytic cycles of topoisomerase 1 and 2 (TOP1 and TOP2) can be interrupted by chemotherapeutic agents (e.g., topotecan and etoposide, respectively), stabilizing the covalent links to DNA and forming topoisomerase cleavage complexes (TOP1/2-ccs, also known as TOP1/2-DPCs) (Liu et al., 1996; Champoux, 2001). The resulting 3′- and 5′-phosphotyrosyl bonds (3′-pY and 5′-pY) that link TOP1 and TOP2 to DNA can be hydrolyzed by specialized tyrosyl-DNA phosphodiesterase 1 and 2 (TDP1 and TDP2), respectively (Yang et al., 1996; Cortes Ledesma et al., 2009). Topoisomerase DPCs can also be generated via self-trapping mutations (e.g., E. coli topoisomerase I R321K/F/L or human topoisomerase 3B R338W) that inhibit the resealing step in their catalytic cycles (Narula et al., 2011; Saha et al., 2020). Similarly, the flippase recombinase (Flp) mutant H305L site-specifically crosslinks to a FRT recognition site via a 3′-pY, mimicking TOP1-DPCs (Nielsen et al., 2009). In analogy to TOP2, SPO11 induces concerted DSBs to initiate meiotic recombination by forming cleavage complexes that covalently link the catalytic tyrosines to DNA through 5′-Ys (Keeney et al., 1997; Johnson et al., 2021; Prieler et al., 2021). Additionally, the Epstein-Barr virus protein EBNA1 covalently binds to the origin of replication site (oriP) to promote viral replication termination (Dheekollu et al., 2021). Enzymes with AP lyase activity can also become covalently trapped on DNA when acting on abasic sites, such as DNA polymerase β (Polβ) (DeMott et al., 2002), DNA-formamidopyrimidine glycosylase (Fpg) (Gilboa et al., 2002), and poly (ADP-ribose) polymerase 1 (PARP1) (Prasad et al., 2014; Prasad et al., 2020). Alternatively, abasic sites on ssDNA are rapidly crosslinked and protected by HMCES, which represents a beneficial DPC that prevents AP sites from detrimental processing (Mohni et al., 2019). Last, DNA methyltransferases, such as DNMT1 and M.HpaII, can be covalently trapped on DNA methylation sites with modified nucleotides 5′-aza-dC and 5′-fluro-dC (Santi et al., 1984; Chen et al., 1991; Maslov et al., 2012). Various DPC repair mechanisms have been identified by studying enzymatic DPCs because they form specific lesions that can be readily induced and monitored in cells. Moreover, enzymatic DPCs are of high clinical relevance and have been extensively exploited in cancer treatment using their inducing agents (e.g., topotecan and etoposide) (Topcu, 2001).
Cells utilize three strategies to repair DPCs: 1) targeting the protein to proteolysis; 2) resolving the crosslink bond by hydrolysis; 3) and/or removing the DNA encompassing the protein adduct by DNA excision (Stingele et al., 2017). These strategies are often selectively or coordinately used to repair DPCs (Kuhbacher and Duxin, 2020). Importantly, the crosslinked proteins undergo extensive modifications during the repair process, including ubiquitylation, SUMOylation, and PARylation. Emerging evidence indicates that these PTMs are part of the sensing response directing the DPC repair pathways. In recent years, it has become evident that DNA replication stalling at DPCs triggers PTMs on DPCs to target their removal. However, DPCs are also efficiently targeted for repair by PTMs in the absence of DNA replication. Below, we summarize the mechanisms by which DPCs are sensed and targeted for removal via PTMs conjugated and processed by corresponding PTM writers and readers (Table 1).
Replication-Coupled DNA-Protein Crosslink Targeting and Proteolysis
The bulkiness of crosslinked proteins makes DPCs detrimental to normal DNA processes. Thus, proteolysis is often required to reduce the bulkiness of DPCs (Vaz et al., 2017). Ubiquitylation, with certain chain types (i.e., K48/K11), can target substrate proteins for degradation by proteolysis. Indeed, ubiquitylation has been identified on many types of DPCs, rendering them permissible to degradation by the proteasome or specialized proteases. Particularly, the process of DNA replication triggers DPC ubiquitylation by replisome- and ssDNA-associated ubiquitin ligases.
Replication-Coupled DNA-Protein Crosslink Ubiquitylation by TRAIP
Unrepaired DPCs block DNA transaction processes including DNA replication (Kuo et al., 2007; Duxin et al., 2014). Replication-coupled DPC proteolysis was first identified in Xenopus egg extracts using a methyltransferase-based DPC (M.HpaII) site-specifically linked to duplex DNA (Duxin et al., 2014). This finding coincided with the discovery in yeast of the first DPC protease, Wss1 (Stingele et al., 2014). In egg extracts, it was found that DPCs stall replisome translocation, which activates a series of events that ultimately leads to the degradation of the protein adduct by the metalloproteases SPRTN (the functional homolog of Wss1 in metazoans) and/or the proteasome (Larsen et al., 2019). Upon replisome-DPC collision, the DPC is first ubiquitylated by the replisome-associated ubiquitin ligase TRAIP, which stimulates the bypass of the protein adduct by the CMG helicase and the subsequent degradation of the DPC by the proteasome (Larsen et al., 2019) (Figure 1A). In the absence of TRAIP, DPC ubiquitylation is delayed but still occurs, suggesting that additional ubiquitin ligase(s) act on the DPC downstream of TRAIP (likely RFWD3; reviewed below and Figure 1B). Importantly, the function of TRAIP is not exclusive to DPC repair. TRAIP also ubiquitylates opposing CMGs that converge on DNA inter-strand crosslinks (ICLs) to stimulate ICL unhooking by the DNA glycosylase NEIL3 or to promote the unloading of CMG and subsequent repair of the ICL by the Fanconi anemia pathway (Wu et al., 2019; Li et al., 2020). TRAIP also triggers CMG unloading at stalled replisomes to enable mitotic DNA synthesis (MiDAs) (Deng et al., 2019; Priego Moreno et al., 2019; Sonneville et al., 2019). Interestingly, the AlphaFold predicted structure of TRAIP suggests a “fishing pole” like conformation made of successive long alpha helices with the ubiquitin ligase RING domain located at one end of the pole (https://alphafold.ebi.ac.uk/entry/Q9BWF2). Such conformation would be well suited for TRAIP to interact with the replisome while simultaneously reaching and targeting protein roadblocks ahead of CMG, conferring TRAIP a universal function in ubiquitylating substrates that hinder replisome translocation.
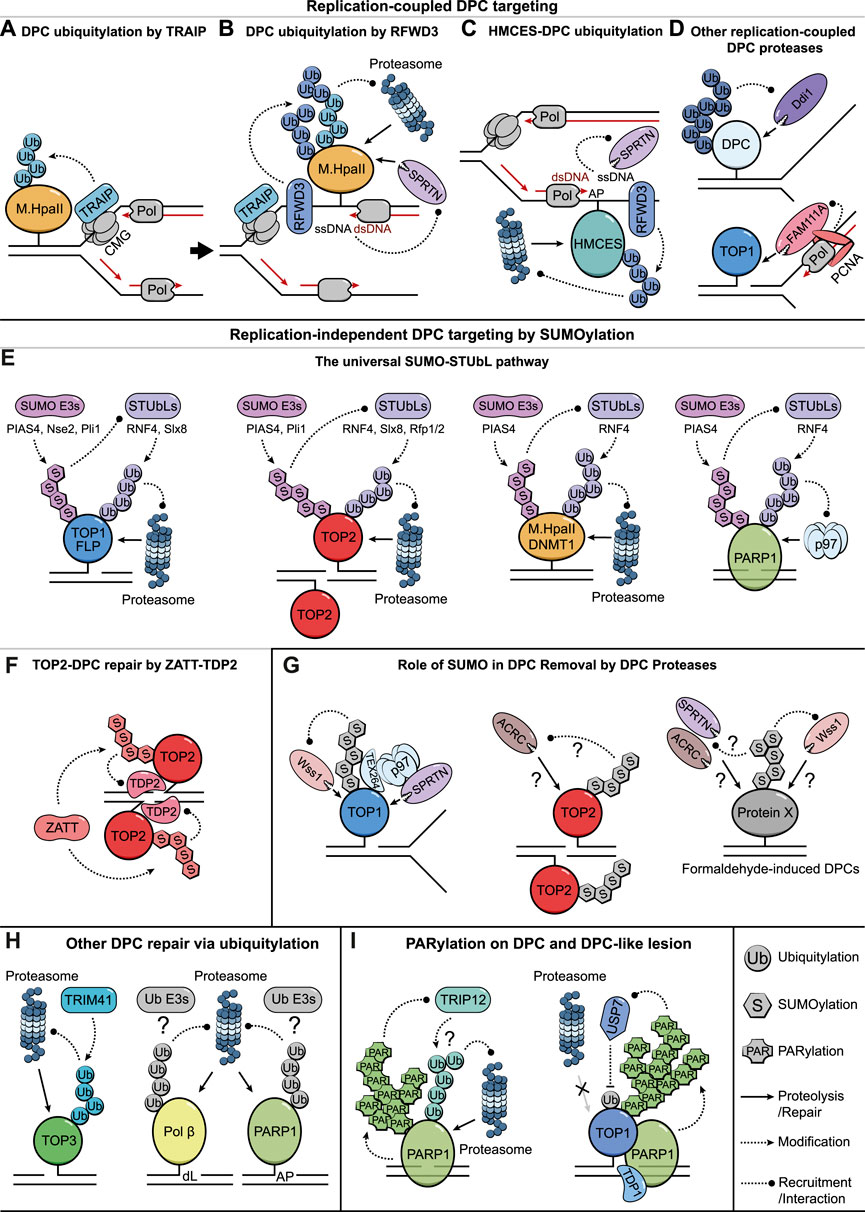
FIGURE 1. Targeting DPCs for Removal via PTMs. (A) A schematic illustration of TRAIP-mediated ubiquitylation of DPCs that hinder CMG progression. (B) CMG bypass of DPCs exposes ssDNA and likely triggers RFWD3-mediated DPC ubiquitylation that further leads to proteolysis by the proteasome and SPRTN. SPRTN protease is targeted by ssDNA/dsDNA junctions. (C) Putative models illustrating how HMCES-DPCs on AP sites are either ubiquitylated by RFWD3 to undergo proteasomal degradation or targeted by SPRTN via nascent DNA strands extended to the lesion. (D) Ddi1/DDI2 might target DPCs with long ubiquitin chains, presumably associated with DNA replication (top illustration). FAM111A degrades DPCs during DNA replication via its interaction with PCNA (bottom illustration). (E) The SUMO-STUbL pathway targets DPCs to degradation in the absence of DNA replication and serves as a universal repair solution for various types of DPCs and DPC-like lesions. (F) ZATT-mediated TOP2-DPC SUMOylation facilitates the recruitment of TDP2 to hydrolyze the covalent linkages. (G) Role of SUMOylation in promoting the removal of TOP1/2-DPCs and formaldehyde-induced DPCs by DPC proteases. (H) Ubiquitylation-mediated proteasomal degradation of crosslinked TOP3B, Polβ-, and PARP1-DPCs. (I) PARP1 auto-PARylation limits PARP1-traping via TRIP12-mediated ubiquitylation and proteasomal degradation (left illustration). PARylation stimulates TDP1 recruitment to TOP1-DPCs while also preventing their proteasomal degradation by recruiting the deubiquitylating enzyme USP7 (right illustration).
Ubiquitylation of DNA-Protein Crosslink on ssDNA by RFWD3
Once bypassed by the CMG helicase, DPCs block DNA synthesis behind replication forks exposing ssDNA. Such ssDNA is quickly coated by the ssDNA-binding replication protein A (RPA), which associates with the ubiquitin ligase RFWD3 (Elia et al., 2015; Feeney et al., 2017). In Xenopus egg extracts, RFWD3 stimulates ubiquitylation of DPCs on ssDNA (i.e., M.HpaII-, Fpg-, and HMCES-DPCs), which likely further promotes their proteolysis during replication (Gallina et al., 2021) (Figure 1B). However, as seen for TRAIP, RFWD3 function is not exclusive to DPC repair. In fact, RFWD3 seems to indiscriminately ubiquitylate proteins that stably associate with ssDNA such as RPA and RAD51 to promote their turnover during replication stress (Elia et al., 2015; Inano et al., 2017). RFWD3 also stimulates DNA damage bypass of a variety of DNA lesions by promoting protein recruitment to ssDNA gaps via PCNA ubiquitylation (Gallina et al., 2021). In short, while TRAIP senses and stimulates the bypass or removal of obstacles such as DPCs that block CMG translocation in front of the replication fork (Figure 1A), RFWD3 does so by ubiquitylating proteins on ssDNA generated behind the fork caused by lesions like DPCs that impair DNA synthesis (Figure 1B). In contrast to TRAIP, the function of RFWD3 can be uncoupled from the replisome and may operate on RPA coated DNA gaps throughout the cell cycle (Gallina et al., 2021).
Consistent with its activity in targeting proteins on ssDNA, RFWD3 may also have an important function in removing HMCES-DPCs, which rapidly form on ssDNA AP sites behind replication forks (Mohni et al., 2019; Thompson et al., 2019). HMCES-DPCs at AP sites induced by UV were shown to be ubiquitylated and further stabilized by proteasome inhibition in cells (Mohni et al., 2019). Consistently, HMCES-DPCs on ssDNA are ubiquitylated by RFWD3 in the absence of SPRTN in Xenopus egg extracts (Gallina et al., 2021). Whether RFWD3 also ubiquitylates HMCES-DPCs in mammalian cells remains to be investigated. Nevertheless, it is tempting to speculate that, once crosslinked to ssDNA, HMCES-DPCs are either rapidly degraded by SPRTN (i.e., via nascent DNA strand synthesized up to the lesion; see below) (Semlow et al., 2022) or ubiquitylated by RFWD3 to promote their degradation (Figure 1C). However, the impacts of HMCES-DPC formation and subsequent degradation on the DNA damage tolerance response remain vastly unknown.
Ubiquitin and Replication-Coupled DNA-Protein Crosslink Proteolysis
DPC ubiquitylation triggered by replication is essential to recruit the proteasome for DPC degradation (Figure 1B) (Larsen et al., 2019). This was shown in egg extracts by generating a M.HpaII-DPC substrate where all ubiquitin acceptor lysines were methylated, which shielded the DPC from ubiquitylation and degradation by the proteasome. Independently of the proteasome, SPRTN also degrades DPCs during DNA replication and is likely the preferred replication-coupled protease operating in cells (Lopez-Mosqueda et al., 2016; Stingele et al., 2016; Vaz et al., 2016; Larsen et al., 2019). SPRTN can process a broad spectrum of DPC and tightly associated DNA binding protein substrates both in cells and in vitro (e.g., TOP1/2-, HMCES-, M.HpaII-, and Eos-DPCs; formaldehyde induced DPCs; and non-covalently associated histones, PARP1, CHK1, and USP1) (Lopez-Mosqueda et al., 2016; Stingele et al., 2016; Maskey et al., 2017; Morocz et al., 2017; Borgermann et al., 2019; Halder et al., 2019; Larsen et al., 2019; Fielden et al., 2020; Saha et al., 2021; Coleman et al., 2022; Kroning et al., 2022; Semlow et al., 2022). In contrast to the proteasome, SPRTN-mediated DPC degradation still occurred in egg extracts without DPC ubiquitylation (on the methylated DPC substrate), albeit with slower kinetics (Larsen et al., 2019). This indicates that although DPC ubiquitylation likely stimulates SPRTN activity, it is not strictly required to target SPRTN to the DPC. Instead, SPRTN is activated by nascent DNA strands synthesized up to the lesion (Larsen et al., 2019). By binding to ssDNA and dsDNA simultaneously via two distinct DNA binding domains (ZBD and BR, respectively) (Reinking et al., 2020b), SPRTN activity is safely localized to ssDNA/dsDNA junctions that are generated behind the replication fork (Larsen et al., 2019; Reinking et al., 2020b) (Figure 1B). Notably, SPRTN ZBD appears to shield its metalloprotease active site when SPRTN is unbound to DNA, representing another layer of regulation that activates the protease upon DNA binding (Li et al., 2019). These mechanisms safeguard essential replisome components from promiscuous SPRTN activity and are consistent with CMG’s remarkable capacity to bypass DPCs encountered on its translocating strand (Sparks et al., 2019). Moreover, SPRTN activity also requires its UBZ domain, which suggests that ubiquitylation on proteins other than the DPC may further help SPRTN localize behind the fork (Morocz et al., 2017; Larsen et al., 2019). SPRTN can also be deactivated by degradation and autocleavage via a mono-ubiquitylation switch, which can be antagonized by the deubiquitylating enzymes USP7, VCPIP, and/or USP11 (Huang et al., 2020; Perry et al., 2021; Zhao et al., 2021). In summary, replisomes act as sensing machineries that detect DPCs and elicit their degradation via direct ubiquitylation but also by generating intermediates that activate the DNA structure-specific protease SPRTN (Figure 1B). As observed for DNA replication, DPCs also block RNA polymerases during DNA transcription (Desai et al., 2003; Sordet et al., 2008; Nakano et al., 2012; Ji et al., 2019). It is therefore conceivable that transcription may offer another efficient sensing mechanism that triggers PTM-mediated DPC removal.
DNA-Protein Crosslink Removal by Other Proteases During DNA Replication
In addition to SPRTN and the proteasome, other proteases have recently been implicated in DPC removal during S-phase. The human trypsin-like protease FAM111A was first shown to associate with nascent chromatin via an interaction with PCNA (Alabert et al., 2014). More recently, it was shown to participate in the resolution of TOP1-DPCs and trapped PARP1 during DNA replication (Kojima et al., 2020). FAM111A’s PCNA-interacting protein (PIP) box and its protease activity appear essential for the removal of these lesions, suggesting that PCNA recruits FAM111A during DNA replication to process protein roadblocks that impede DNA synthesis (Kojima et al., 2020) (Figure 1D). While it is still unknown whether PTMs, such as ubiquitylation, direct FAM111A activity, dysregulation of FAM111A protease severely impacts DNA replication and transcription highlighting the critical need to fine-tune protease activity in cells (Hoffmann et al., 2020; Nie et al., 2021). In contrast, the yeast aspartic protease Ddi1 and its human homolog DDI2 specifically cleave substrates containing long ubiquitin chains, potentially acting as a backup proteolysis pathway for substrates that escape proteasomal degradation (Dirac-Svejstrup et al., 2020; Yip et al., 2020). The protease activity of yeast Ddi1 was shown to contribute to the repair of Top1- and Flp-DPCs (Serbyn et al., 2020). Additionally, the recruitment of Ddi1 to DPCs coincides with the beginning of S-phase (Serbyn et al., 2020), suggesting that Ddi1 removes ubiquitylated DPCs during DNA replication but whether this is also the case in vertebrates remains unknown (Figure 1D). Taken together, FAM111A and Ddi1 represent two novel proteases that participate in DPC repair in S-phase, but their regulation and interplay with other replication-coupled DPC repair mechanisms warrant further investigations.
Replication-Independent DNA-Protein Crosslink Targeting by SUMOylation
While DNA replication is an efficient way to sense and remove DPCs, recent evidence has highlighted the versatile roles of SUMOylation in triggering DPC repair independently of DNA replication. SUMO-targeted DPC resolution can be achieved either by their direct proteolysis via SUMO-targeted ubiquitylation, or through specialized pathways that remove SUMOylated DPCs in the absence of subsequent ubiquitylation.
The Universal SUMO-STUbL DNA-Protein Crosslink Removal Pathway
SUMOylation of proteins can target them for degradation via SUMO-targeted ubiquitin ligases (STUbLs) (Prudden et al., 2007). The role of the SUMO-STUbL pathway in DPC repair was first suggested in fission yeast. It was shown that Top1-DPCs are first SUMOylated by the SUMO ligases Nse2 (NSMCE2 in humans) or Pli1 (PIAS family proteins in humans) and subsequently ubiquitylated by Slx8 (RNF4 in humans) to stimulate Top1-DPC repair in the absence of Tdp1 (Heideker et al., 2011; Steinacher et al., 2013). The same SUMO-STUbL pathway mediated by Pli1 and Slx8 was also observed on Top2-DPCs in fission yeast (Wei et al., 2017) and on Top1/2-DPCs in budding yeast, via Siz1 (PIAS4 in human) and Slx5-Slx8 (Sun et al., 2020). Likewise, Flp-DPCs also undergo SUMO-targeted ubiquitylation, which promotes their degradation by the proteasome (Ma et al., 2019) suggesting a conserved TOP-DPC resolution mechanism via the SUMO-STUbL pathway (Figure 1E). Importantly, recent advances demonstrated that this pathway also operates in human cells where SUMOylation by PIAS4 and subsequent ubiquitylation by RNF4 stimulate TOP1/2-DPC repair by proteasomal degradation (Sun et al., 2020) (Figure 1E). These findings rationalize the original observations that TOP1/2-DPCs undergo SUMO conjugation and ubiquitylation-mediated proteasomal degradation (Desai et al., 1997; Mao et al., 2000a; Mao et al., 2000b; Mao et al., 2001; Lin et al., 2008). However, whether such ubiquitin-mediated resolution of TOP-DPCs is exclusively dependent on the SUMO-STUbL pathway or whether, in some instances, SUMO-independent ubiquitin ligases act on TOP1/2-DPCs is still unclear (see below).
Recent studies in Xenopus egg extracts and human cells showed that the SUMO-STUbL pathway can be extended to other types of DPCs and DPC-like lesions (Figure 1E). DNA methyltransferase DPCs (e.g., DNMT1 and M.HpaII) on duplex DNA undergo extensive SUMOylation, which similarly stimulates their RNF4-mediated ubiquitylation and proteasomal degradation (Figure 1E) (Liu et al., 2021). While DPC SUMOylation in Xenopus egg extracts is primarily performed by PIAS4, multiple SUMO ligases appear to compensate for PIAS4 activity on DNMT1-DPCs in human cells, and this is also likely the case for TOP1/2-DPCs (Sun et al., 2020; Liu et al., 2021). Notably, DPC sensing and repair via the SUMO-STUbL pathway does not rely on DNA replication, underlining the autonomous function of this pathway (Liu et al., 2021). Moreover, unrepaired DPCs, due to inhibition of RNF4, evade DNA damage checkpoint signaling and cause chromosomal instability during mitosis, highlighting the need to repair DPCs that are formed post-replicatively before cell division (Liu et al., 2021). This pathway may also be critical in non-dividing cells that must sense and remove DPCs independently of DNA replication. Recently, PIAS4-RNF4 mediated repair has also been reported for non-covalently trapped PARP1 (Krastev et al., 2022), a DPC-like lesion induced by PARP inhibitors that impedes DNA processes (Murai and Pommier, 2019). Interestingly, removing trapped PARP1 via the PIAS4-RNF4 pathway relies on the unfoldase p97 (Figure 1E) (Krastev et al., 2022). Thus, DPCs and non-covalently trapped proteins elicit the same SUMO-ubiquitylation response. While proteolysis is essential to resolve DPCs, p97 unfoldase activity might be sufficient to release non-covalently trapped proteins from chromatin. Additionally, formaldehyde treatment of cells also induces a heavy SUMOylation response on chromatin and these cells rely on RNF4 to survive (Borgermann et al., 2019; Liu et al., 2021), supporting a universal role of the SUMO-STUbL pathway in the repair of all DPCs.
Taken together, the SUMO-STUbL pathway triggers DPC removal independently of DNA replication, suggesting that other DPC sensing mechanisms are engaged. Whereas PIAS family SUMO ligases may rely on their conserved DNA-binding SAP domain (Aravind and Koonin, 2000; Sun et al., 2020) to sense DPCs on DNA, other DNA scanning mechanisms may also exist to stimulate PIAS4 recruitment or other SUMO ligases to initiate SUMO-STUbL DPC removal. Although the SUMO-STUbL pathway appears to be a universal DPC repair solution, it may not always be the preferred choice and perhaps serves as a backup mechanism for DPCs escaping or lacking specialized repair pathways (reviewed below).
ZATT-Mediated TOP2-DNA-Protein Crosslink Resolution
Although DPCs can be targeted for proteasomal degradation by the SUMO-STUbL pathway, a more direct proteolysis-free approach has been observed on TOP2-DPCs (Schellenberg et al., 2017). Initial observations showed that covalent TOP2 crosslinking by teniposide and non-covalent TOP2 trapping by ICRF-193 trigger a swift TOP2-SUMOylation response (Mao et al., 2000a; Isik et al., 2003). Recently, it was shown that the hydrolysis of the 5′-pY bonds of TOP2-DPCs by TDP2 is facilitated by TOP2 conformational changes induced by the SUMO ligase ZATT/ZNF451 (Schellenberg et al., 2017) (Figure 1F). TOP2 SUMOylation by ZATT recruits TDP2, which binds SUMOylated TOP2 via its split SUMO-interacting motif (SIM) and subsequently hydrolyzes the 5′-pYs. This pathway protects TOP2 from degradation, presumably allowing TOP2 recycling and downstream repair of the adduct-free DSB by non-homologous end-joining (NHEJ) (Gomez-Herreros et al., 2013). Thus, ZATT-dependent TOP2 SUMOylation could be considered as a safe mode of TOP2-DPC repair and perhaps the preferred choice employed by cells. This is consistent with the severe sensitivity of ZATT-deficient cells to TOP2 poisons (i.e., etoposide), which contrasts to the lack of sensitivity observed in the absence of RNF4 (Schellenberg et al., 2017; Olivieri et al., 2020). However, under certain conditions or when ZATT becomes limited because of excessive TOP2-DPC generation (e.g., upon etoposide treatment), cells may also rely on the SUMO-STUbL pathway to degrade the protein adduct. Curiously, ZATT-deficient cells are even more sensitive to etoposide than TDP2-deficient cells, suggesting that ZATT possesses TDP2-independent functions in repairing TOP2-DPCs (Schellenberg et al., 2017). This could be related to a role of ZATT in recruiting the DNA translocase PICH by SUMOylating TOP2 to promote genome stability upon replication stress (Tian et al., 2021), although whether PICH localizes in the nucleus in interphase is debatable (Nielsen et al., 2015). Yet, ZATT-mediated repair appears specific to TOP2-DPCs, highlighting the relevance and abundance of endogenous TOP2-DPCs in cells (without exogenous TOP2 poisons). Whether analogous specialized pathways operate on other abundant DPCs in the absence of proteolysis remains to be discovered.
Role of SUMO in DNA-Protein Crosslink Removal by DNA-Protein Crosslink Proteases
In addition to its role in stimulating DPC removal via the STUbL pathway, DPC SUMOylation has been shown to stimulate DPC removal by DPC proteases. DPC proteases emerged as an efficient way to resolve DPCs, since the discovery of the first DPC protease Wss1 in yeast (Stingele et al., 2014). Wss1 was originally characterized as a SUMO-binding isopeptidase that cleaves SUMO-conjugated protein substrates and also removes ubiquitin from ubiquitin/SUMO hybrid chains in vitro (Mullen et al., 2010). Wss1 was then shown to degrade proteins entrapped on DNA including camptothecin-induced Top1-DPCs (Stingele et al., 2014). Consistent with SUMO conjugation on TOP1-DPCs (Mao et al., 2000b), the DPC protease activity of Wss1 (harboring SIM domains) is largely SUMO-targeted and associated with Cdc48/p97 activity (Stingele et al., 2014; Balakirev et al., 2015) (Figure 1G). Interestingly, a more recent study in yeast showed that the SUMO ligase Siz2 (PIAS4 in humans) SUMOylates proteins in the vicinity of DPCs to stimulate Wss1 recruitment to the lesion (Serbyn et al., 2021). Proteins associated with DNMT1 are also SUMOylated in cells following DNMT1 trapping via 5-aza-dC treatment (Borgermann et al., 2019). Thus, it appears that the DPC-triggered SUMO response not only occurs on DPCs but also on the proteins in close interaction with DPCs, forming a SUMO hub that boosts the recruitment of SUMO-targeted repair factors (e.g., SUMO proteases or STUbLs).
In vertebrates, the metalloproteases SPRTN and ACRC (GCNA) are phylogenetically related to yeast Wss1 (Vaz et al., 2017; Reinking et al., 2020a). Notably, while SPRTN carries a ubiquitin-binding domain (UBZ), ACRC possesses SUMO-interacting motifs (SIMs). Despite the lack of SIM, the DPC protease activity of SPRTN was recently shown to interact with SUMO- and ubiquitin-conjugated non-enzymatic DPCs during DNA replication (Ruggiano et al., 2021). Additionally, SPRTN can be recruited along with p97 to SUMO-conjugated TOP1-DPCs via the p97 co-factor TEX264, which associates with replication fork presumably tethering SPRTN to DPCs that block ongoing DNA replication (Fielden et al., 2020) (Figure 1G). However, the stimulatory function of SUMO on SPRTN activity has yet to be confirmed in vitro, and could be caused by indirect effects due to the global role of SUMOylation on DNA replication (Lecona et al., 2016; Franz et al., 2021). In contrast to SPRTN, which is expressed in different cell types, ACRC (GCNA) is mainly expressed in germ cells (Carmell et al., 2016) where it was recently shown to target TOP2-DPCs during meiosis (Bhargava et al., 2020; Dokshin et al., 2020) (Figure 1G). When ectopically expressed in mammalian cells, ACRC can be recruited to DNMT1-DPC sites via its SIMs and participate in DMNT1-DPC resolution (Borgermann et al., 2019). However, whether ACRC is a SUMO-targeted DPC protease remains to be formally demonstrated. In germ cells, the specialized topoisomerase-like enzyme SPO11, forms DPCs similarly to TOP2-DPCs via 5′-pYs to direct meiotic recombination (Keeney et al., 1997; Johnson et al., 2021; Prieler et al., 2021). SPO11-DPCs are processed via MRN endo/exonuclease activity, which excises the DNA crosslinked to SPO11 to initiate meiotic recombination (Neale et al., 2005; Garcia et al., 2011). Although the SUMO response on SPO11-DPCs has not yet been observed, whether ACRC processes SPO11-DPCs is an interesting object for future research. Additionally, Wss1, SPRTN, and ACRC were all shown to counteract formaldehyde-induced DPCs (Stingele et al., 2014; Borgermann et al., 2019; Ruggiano et al., 2021), consistent with the potent SUMOylation response on chromatin induced by formaldehyde (Borgermann et al., 2019; Ruggiano et al., 2021) (Figure 1G). However, whether these proteases directly act on formaldehyde-induced DPCs via SUMO targeting is not clear.
To sum up, while the action of Wss1 in yeast is clearly linked to the SUMO system (Stingele et al., 2014; Balakirev et al., 2015), this link has not been firmly validated for vertebrate DPC proteases. Although SPRTN can process SUMO-conjugated DPCs (Fielden et al., 2020; Ruggiano et al., 2021), its recruitment to lesions does not seem to rely on SUMOylation (Borgermann et al., 2019; Ruggiano et al., 2021) and SUMO inhibition in Xenopus egg extracts does not affect replication-coupled DPC proteolysis (Liu et al., 2021). ACRC, albeit harboring SIMs, has yet to be shown to process SUMOylated DPCs directly. Thus, whether SPRTN and ACRC depend on SUMOylation to remove DPCs and how they interact with the SUMO-STUbL pathway await further investigation. Considering that DPC SUMOylation can trigger activities that antagonize STUbLs (Nie et al., 2017; Wei et al., 2017), we envisage that the SUMO chain growth on DPCs might act as a timer to record the dwell time of a protein crosslinked to DNA. In this scenario, the initial SUMOylation of the DPC may first facilitate DPC resolution (i.e., by SUMO-induced crosslink reversal or by DPC proteases). However, if primary processing fails or are not available, SUMO chains extension may facilitate the downstream universal SUMO-STUbL removal pathway.
Other DNA-Protein Crosslink Ubiquitin Ligases?
DPC ubiquitylation and degradation can be effectively stimulated by DNA replication or via the SUMO-STUbL pathway. However, some ubiquitin-mediated DPC degradation events have so far not been linked to either pathways. For example, although TOP1/2-DPCs are subjective to STUbL mediated repair, other ubiquitin ligases have been also implicated in their degradation. CUL3 and CUL4B, the scaffold proteins of Cullin-RING E3 ligase complexes, have been shown to counteract TOP1-DPCs and render cells resistance to TOP1 poison camptothecin (Zhang et al., 2004; Kerzendorfer et al., 2010). Another ubiquitin ligase BRCA1 has been shown to contribute to the degradation of TOP1-DPCs encountered by transcription machinery (Sordet et al., 2008). Similarly, the ubiquitylation-mediated degradation of etoposide-induced TOP2-DPCs were shown to be dependent on BMI1/RING1A and SCFβ-TrCP ubiquitin E3 ligase complexes (Alchanati et al., 2009; Shu et al., 2020). However, these findings have so far not been further validated and it remains unclear whether these ubiquitin ligases directly target TOP1/2-DPCs. Other repair-oriented DPC ubiquitylation events have also been observed on the crosslinked TOP3B R338W mutant, Polβ-DPCs, and PARP1-DPCs (Figure 1H) (Quinones et al., 2015; Prasad et al., 2019; Saha et al., 2020). In the case of trapped TOP3B, ubiquitylation was shown to be dependent on the ubiquitin ligase TRIM41, which targets TOP3B for proteasomal degradation to promote resolution of the crosslink by TDP2 (Saha et al., 2020) (Figure 1H). Although Polβ-DPCs and PARP1-DPCs have been suggested to undergo ubiquitin-mediated proteasomal degradation (Quinones et al., 2015; Prasad et al., 2019; Prasad et al., 2020; Quinones et al., 2020), the ubiquitin ligases operating on these DPCs remain unknown. Possibly these results suggest the existence of additional specialized DPC repair pathways that counteract specific DPC-types.
PARylation as an Emerging Post-Translational Modification on DNA-Protein Crosslinks
In addition to ubiquitylation and SUMOylation, PARylation, which is catalyzed by Poly (ADP-ribose) polymerases (PARPs), mainly PARP1, has also been observed on DPCs and may represent another regulatory mechanism of DPC repair (Figure 1I). The dynamic turnover of PARylation on target proteins has been tightly connected to DNA damage repair and genome maintenance (Harrision et al., 2020; Demin et al., 2021). PARP1 is the prime target of PARylation (i.e., auto-PARylation), which stimulates PARP1 release from DNA (Muthurajan et al., 2014; Steffen et al., 2016; Kruger et al., 2020). Additionally, PARylation on PARP1 recruits the PAR-targeted ubiquitin ligase (PTUbL) TRIP12 to mediate proteasomal degradation of PARP1, further limiting PARP1-trapping (Figure 1I) (Gatti et al., 2020). Importantly, PARP-mediated PARylation is activated by DNA damage (i.e., DNA nicks, gaps or breaks) but not by intact DNA (Langelier et al., 2011; Langelier et al., 2012; Rudolph et al., 2021). This suggests that the PARylation response may be excluded from DPCs on intact duplex DNA, but may influence the resolution of DPCs flanked by DNA breaks (e.g., TOP-DPCs and DPCs on ssDNA or ssDNA/dsDNA junctions such as the ones generated during replication). Consistently, PARP1 appears to play a critical role in regulating TOP1-DPC repair. While several studies initially reported that TOP1-DPCs are PARylated by PARP1 in vitro, the outcome of this PARylation in cells was largely unknown (Malanga and Althaus, 2004; Yung et al., 2004; Park and Cheng, 2005). A recent work showed that TOP1-PARylation exhibits an adverse effect on TOP1-DPCs compared to its effect on PARP1 trapping (Sun et al., 2021). By inhibiting poly (ADP-ribose) glycohydrolase (PARG), sustained PARylation on TOP1-DPCs was shown to recruit the deubiquitylating enzyme USP7 to remove ubiquitylation on TOP1-DPCs, thus preventing their proteasomal degradation (Sun et al., 2021) (Figure 1I). Notably, TDP1 interacts with PARP1 and PARylation of TDP1 promotes its recruitment to damage sites for TOP1-DPC resolution (Das et al., 2014), suggesting that PARP1 plays a dual function in orchestrating TOP1-DPC repair (Figure 1I). On one side, PARylation would prevent the premature proteasomal degradation of TOP1-DPC while simultaneously stimulating TDP1 recruitment to the lesion. Following TDP1 recruitment, PARG removal of PAR chains on TOP1-DPCs would allow TOP1-DPC ubiquitylation and degradation by the proteasome and subsequent hydrolysis of the covalent linkage by TDP1. Importantly, the essential role of PARylation in regulating TOP1-DPC repair is highlighted by the synergistic effect of combining PARP inhibitors and TOP1 poisons to kill cancer cells (Murai and Pommier, 2019; Chowdhuri and Das, 2021). It is possible that PARP1 is recruited to other DPCs that are flanked by DNA breaks and exhibits additional roles in regulating DPC repair, which remains largely undiscovered.
Discussion and Perspectives
Current frontiers of research in the DPC field have unveiled the essential roles of PTMs in orchestrating DPC resolution. However, it is worth mentioning that many DPC-forming enzymes, such as topoisomerases and PARP1, are routinely post-translationally modified when associated with DNA in a non-covalent manner. Such PTMs regulate their catalytic activity or affinity to DNA and therefore can influence the level of DPC formation, making it challenging to attribute the roles of PTMs to the formation or the repair of DPCs. While the replication machinery serves as a proficient DPC sensor by directly bumping into DPCs and targeting their removal, how DPCs are recognized in the absence of replication is still under debate. Nevertheless, SUMO appears to play a central role in directing the repair of DPCs that are not sensed by DNA replication. DPC SUMOylation either initiates cost-effective repair pathways (e.g., ZATT-TDP2 for TOP2-DPCs) (Schellenberg et al., 2017) or directly engages DPC proteases (e.g., Wss1 and ACRC) (Stingele et al., 2014; Balakirev et al., 2015; Borgermann et al., 2019). On the other hand, the SUMO-STUbL proteasome pathway functions as the universal solution to ensure DPC removal (Sun et al., 2020; Liu et al., 2021; Krastev et al., 2022). Thus, it is plausible that in the absence of replication, cells recognize DPCs by their retention times on DNA recorded by SUMO chain development and accordingly employ appropriate repair mechanisms. However, additional mechanisms that initially trigger DPC SUMOylation might be engaged, for example, through certain DNA-scanning enzymes or processes like DNA transcription, or via specific DNA structures and topologies, which remain to be identified.
Author Contributions
XL and JPD wrote this review.
Funding
This work was supported by the Danish National Independent Research Found grant # 9060-00030B and the Novo Nordisk Foundation (Grant No. NNF14CC0001).
Conflict of Interest
The authors declare that the research was conducted in the absence of any commercial or financial relationships that could be construed as a potential conflict of interest.
Publisher’s Note
All claims expressed in this article are solely those of the authors and do not necessarily represent those of their affiliated organizations, or those of the publisher, the editors and the reviewers. Any product that may be evaluated in this article, or claim that may be made by its manufacturer, is not guaranteed or endorsed by the publisher.
References
Alabert, C., Bukowski-Wills, J.-C., Lee, S.-B., Kustatscher, G., Nakamura, K., de Lima Alves, F., et al. (2014). Nascent Chromatin Capture Proteomics Determines Chromatin Dynamics During DNA Replication and Identifies Unknown Fork Components. Nat. Cell Biol. 16 (3), 281–291. doi:10.1038/ncb2918
Alchanati, I., Teicher, C., Cohen, G., Shemesh, V., Barr, H. M., Nakache, P., et al. (2009). The E3 Ubiquitin-Ligase Bmi1/Ring1A Controls the Proteasomal Degradation of Top2α Cleavage Complex - A Potentially New Drug Target. PLoS One 4 (12), e8104. doi:10.1371/journal.pone.0008104
Aravind, L., and Koonin, E. V. (2000). SAP - a Putative DNA-Binding Motif Involved in Chromosomal Organization. Trends Biochem. Sci. 25 (3), 112–114. doi:10.1016/s0968-0004(99)01537-6
Bai, J., Zhang, Y., Xi, Z., Greenberg, M. M., and Zhou, C. (2018). Oxidation of 8-Oxo-7,8-Dihydro-2′-Deoxyguanosine Leads to Substantial DNA-Histone Cross-Links within Nucleosome Core Particles. Chem. Res. Toxicol. 31 (12), 1364–1372. doi:10.1021/acs.chemrestox.8b00244
Balakirev, M. Y., Mullally, J. E., Favier, A., Assard, N., Sulpice, E., Lindsey, D. F., et al. (2015). Wss1 Metalloprotease Partners with Cdc48/Doa1 in Processing Genotoxic SUMO Conjugates. Elife 4, e06763. doi:10.7554/eLife.06763
Barker, S., Weinfeld, M., Zheng, J., Li, L., and Murray, D. (2005). Identification of Mammalian Proteins Cross-Linked to DNA by Ionizing Radiation. J. Biol. Chem. 280 (40), 33826–33838. doi:10.1074/jbc.M502477200
Bhargava, V., Goldstein, C. D., Russell, L., Xu, L., Ahmed, M., Li, W., et al. (2020). GCNA Preserves Genome Integrity and Fertility Across Species. Dev. Cell 52 (1), 38–52. doi:10.1016/j.devcel.2019.11.007
Borgermann, N., Ackermann, L., Schwertman, P., Hendriks, I. A., Thijssen, K., Liu, J. C., et al. (2019). SUMO Ylation Promotes Protective Responses to DNA ‐protein Crosslinks. EMBO J. 38 (8), e101496. doi:10.15252/embj.2019101496
Carmell, M. A., Dokshin, G. A., Skaletsky, H., Hu, Y.-C., van Wolfswinkel, J. C., Igarashi, K. J., et al. (2016). A Widely Employed Germ Cell Marker Is an Ancient Disordered Protein with Reproductive Functions in Diverse Eukaryotes. Elife 5, e19993. doi:10.7554/eLife.19993
Champoux, J. J. (2001). DNA Topoisomerases: Structure, Function, and Mechanism. Annu. Rev. Biochem. 70, 369–413. doi:10.1146/annurev.biochem.70.1.369
Chen, L., MacMillan, A. M., Chang, W., Ezaz-Nikpay, K., Lane, W. S., and Verdine, G. L. (1991). Direct Identification of the Active-Site Nucleophile in a DNA (Cytosine-5)-methyltransferase. Biochemistry 30 (46), 11018–11025. doi:10.1021/bi00110a002
Chodosh, L. A. (2001). UV Crosslinking of Proteins to Nucleic Acids. Curr. Protoc. Mol. Biol. 36. Unit 12‐15. doi:10.1002/0471142727.mb1205s36
Chowdhuri, S. P., and Das, B. B. (2021). Top1-PARP1 Association and beyond: from DNA Topology to Break Repair. Nar. Cancer 3 (1), zcab003. doi:10.1093/narcan/zcab003
Chválová, K., Brabec, V., and Kašpárková, J. (2007). Mechanism of the Formation of DNA-Protein Cross-Links by Antitumor Cisplatin. Nucleic Acids Res. 35 (6), 1812–1821. doi:10.1093/nar/gkm032
Coleman, K. E., Yin, Y., Lui, S. K. L., Keegan, S., Fenyo, D., Smith, D. J., et al. (2022). USP1-trapping Lesions as a Source of DNA Replication Stress and Genomic Instability. Nat. Commun. 13 (1), 1740. doi:10.1038/s41467-022-29369-3
Das, B. B., Huang, S.-y. N., Murai, J., Rehman, I., Amé, J.-C., Sengupta, S., et al. (2014). PARP1-TDP1 Coupling for the Repair of Topoisomerase I-Induced DNA Damage. Nucleic Acids Res. 42 (7), 4435–4449. doi:10.1093/nar/gku088
Demin, A. A., Hirota, K., Tsuda, M., Adamowicz, M., Hailstone, R., Brazina, J., et al. (2021). XRCC1 Prevents Toxic PARP1 Trapping during DNA Base Excision Repair. Mol. Cell 81 (14), 3018–3030 e5. doi:10.1016/j.molcel.2021.05.009
DeMott, M. S., Beyret, E., Wong, D., Bales, B. C., Hwang, J.-T., Greenberg, M. M., et al. (2002). Covalent Trapping of Human DNA Polymerase β by the Oxidative DNA Lesion 2-Deoxyribonolactone. J. Biol. Chem. 277 (10), 7637–7640. doi:10.1074/jbc.C100577200
Deng, L., Wu, R. A., Sonneville, R., Kochenova, O. V., Labib, K., Pellman, D., et al. (2019). Mitotic CDK Promotes Replisome Disassembly, Fork Breakage, and Complex DNA Rearrangements. Mol. Cell 73 (5), 915–929. doi:10.1016/j.molcel.2018.12.021
Desai, S. D., Liu, L. F., Vazquez-Abad, D., and D'Arpa, P. (1997). Ubiquitin-dependent Destruction of Topoisomerase I Is Stimulated by the Antitumor Drug Camptothecin. J. Biol. Chem. 272 (39), 24159–24164. doi:10.1074/jbc.272.39.24159
Desai, S. D., Zhang, H., Rodriguez-Bauman, A., Yang, J.-M., Wu, X., Gounder, M. K., et al. (2003). Transcription-dependent Degradation of Topoisomerase I-DNA Covalent Complexes. Mol. Cell Biol. 23 (7), 2341–2350. doi:10.1128/MCB.23.7.2341-2350.2003
Dheekollu, J., Wiedmer, A., Ayyanathan, K., Deakyne, J. S., Messick, T. E., and Lieberman, P. M. (2021). Cell-cycle-dependent EBNA1-DNA Crosslinking Promotes Replication Termination at oriP and Viral Episome Maintenance. Cell 184 (3), 643–654 e613. doi:10.1016/j.cell.2020.12.022
Dirac-Svejstrup, A. B., Walker, J., Faull, P., Encheva, V., Akimov, V., Puglia, M., et al. (2020). DDI2 Is a Ubiquitin-Directed Endoprotease Responsible for Cleavage of Transcription Factor NRF1. Mol. Cell 79 (2), 332–341 e337. doi:10.1016/j.molcel.2020.05.035
Dokshin, G. A., Davis, G. M., Sawle, A. D., Eldridge, M. D., Nicholls, P. K., Gourley, T. E., et al. (2020). GCNA Interacts with Spartan and Topoisomerase II to Regulate Genome Stability. Dev. Cell 52 (1), 53–68. doi:10.1016/j.devcel.2019.11.006
Duxin, J. P., Dewar, J. M., Yardimci, H., and Walter, J. C. (2014). Repair of a DNA-Protein Crosslink by Replication-Coupled Proteolysis. Cell 159 (2), 346–357. doi:10.1016/j.cell.2014.09.024
Elia, A. E. H., Wang, D. C., Willis, N. A., Boardman, A. P., Hajdu, I., Adeyemi, R. O., et al. (2015). RFWD3-Dependent Ubiquitination of RPA Regulates Repair at Stalled Replication Forks. Mol. Cell 60 (2), 280–293. doi:10.1016/j.molcel.2015.09.011
Esterbauer, H., Cheeseman, K. H., Dianzani, M. U., Poli, G., and Slater, T. F. (1982). Separation and Characterization of the Aldehydic Products of Lipid Peroxidation Stimulated by ADP-Fe2+ in Rat Liver Microsomes. Biochem. J. 208 (1), 129–140. doi:10.1042/bj2080129
Feeney, L., Muñoz, I. M., Lachaud, C., Toth, R., Appleton, P. L., Schindler, D., et al. (2017). RPA-mediated Recruitment of the E3 Ligase RFWD3 Is Vital for Interstrand Crosslink Repair and Human Health. Mol. Cell 66 (5), 610–621 e614. doi:10.1016/j.molcel.2017.04.021
Fielden, J., Wiseman, K., Torrecilla, I., Li, S., Hume, S., Chiang, S.-C., et al. (2020). TEX264 Coordinates P97- and SPRTN-Mediated Resolution of Topoisomerase 1-DNA Adducts. Nat. Commun. 11 (1), 1274. doi:10.1038/s41467-020-15000-w
Franz, A., Valledor, P., Ubieto-Capella, P., Pilger, D., Galarreta, A., Lafarga, V., et al. (2021). USP7 and VCPFAF1 Define the SUMO/Ubiquitin Landscape at the DNA Replication Fork. Cell Rep. 37 (2), 109819. doi:10.1016/j.celrep.2021.109819
Gallina, I., Hendriks, I. A., Hoffmann, S., Larsen, N. B., Johansen, J., Colding-Christensen, C. S., et al. (2021). The Ubiquitin Ligase RFWD3 Is Required for Translesion DNA Synthesis. Mol. Cell 81 (3), 442–458. doi:10.1016/j.molcel.2020.11.029
Garcia, V., Phelps, S. E. L., Gray, S., and Neale, M. J. (2011). Bidirectional Resection of DNA Double-Strand Breaks by Mre11 and Exo1. Nature 479 (7372), 241–244. doi:10.1038/nature10515
Gatti, M., Imhof, R., Huang, Q., Baudis, M., and Altmeyer, M. (2020). The Ubiquitin Ligase TRIP12 Limits PARP1 Trapping and Constrains PARP Inhibitor Efficiency. Cell Rep. 32 (5), 107985. doi:10.1016/j.celrep.2020.107985
Gilboa, R., Zharkov, D. O., Golan, G., Fernandes, A. S., Gerchman, S. E., Matz, E., et al. (2002). Structure of Formamidopyrimidine-DNA Glycosylase Covalently Complexed to DNA. J. Biol. Chem. 277 (22), 19811–19816. doi:10.1074/jbc.M202058200
Gómez-Herreros, F., Romero-Granados, R., Zeng, Z., Álvarez-Quilón, A., Quintero, C., Ju, L., et al. (2013). TDP2-dependent Non-homologous End-Joining Protects against Topoisomerase II-Induced DNA Breaks and Genome Instability in Cells and In Vivo. PLoS Genet. 9 (3), e1003226. doi:10.1371/journal.pgen.1003226
Groehler, A., Degner, A., and Tretyakova, N. Y. (2017). Mass Spectrometry-Based Tools to Characterize DNA-Protein Cross-Linking by Bis -Electrophiles. Basic Clin. Pharmacol. Toxicol. 121 (Suppl. 3), 63–77. doi:10.1111/bcpt.12751
Halder, S., Torrecilla, I., Burkhalter, M. D., Popović, M., Fielden, J., Vaz, B., et al. (2019). SPRTN Protease and Checkpoint Kinase 1 Cross-Activation Loop Safeguards DNA Replication. Nat. Commun. 10 (1), 3142. doi:10.1038/s41467-019-11095-y
Harrision, D., Gravells, P., Thompson, R., and Bryant, H. E. (2020). Poly(ADP-Ribose) Glycohydrolase (PARG) vs. Poly(ADP-Ribose) Polymerase (PARP) - Function in Genome Maintenance and Relevance of Inhibitors for Anti-cancer Therapy. Front. Mol. Biosci. 7, 191. doi:10.3389/fmolb.2020.00191
Heck, H. d. A., Casanova-schm1Tz, M., Dodd, P. B., Schachter, E. N., Witek, T. J., and Tosun, T. (1985). Formaldehyde (CH2O) Concentrations in the Blood of Humans and Fischer-344 Rats Exposed to CH2O under Controlled Conditions. Am. Industrial Hyg. Assoc. J. 46 (1), 1–3. doi:10.1080/15298668591394275
Heideker, J., Prudden, J., Perry, J. J. P., Tainer, J. A., and Boddy, M. N. (2011). SUMO-targeted Ubiquitin Ligase, Rad60, and Nse2 SUMO Ligase Suppress Spontaneous Top1-Mediated DNA Damage and Genome Instability. PLoS Genet. 7 (3), e1001320. doi:10.1371/journal.pgen.1001320
Hoffmann, S., Pentakota, S., Mund, A., Haahr, P., Coscia, F., Gallo, M., et al. (2020). FAM111 Protease Activity Undermines Cellular Fitness and Is Amplified by Gain‐of‐function Mutations in Human Disease. EMBO Rep. 21 (10), e50662. doi:10.15252/embr.202050662
Huang, J., Zhou, Q., Gao, M., Nowsheen, S., Zhao, F., Kim, W., et al. (2020). Tandem Deubiquitination and Acetylation of SPRTN Promotes DNA-Protein Crosslink Repair and Protects against Aging. Mol. Cell 79 (5), 824–835. doi:10.1016/j.molcel.2020.06.027
Inano, S., Sato, K., Katsuki, Y., Kobayashi, W., Tanaka, H., Nakajima, K., et al. (2017). RFWD3-Mediated Ubiquitination Promotes Timely Removal of Both RPA and RAD51 from DNA Damage Sites to Facilitate Homologous Recombination. Mol. Cell 66 (5), 622–634. doi:10.1016/j.molcel.2017.04.022
Isik, S., Sano, K., Tsutsui, K., Seki, M., Enomoto, T., Saitoh, H., et al. (2003). The SUMO Pathway Is Required for Selective Degradation of DNA Topoisomerase IIbeta Induced by a Catalytic Inhibitor ICRF-193(1). FEBS Lett. 546 (2-3), 374–378. doi:10.1016/s0014-5793(03)00637-9
Ji, S., Thomforde, J., Rogers, C., Fu, I., Broyde, S., and Tretyakova, N. Y. (2019). Transcriptional Bypass of DNA-Protein and DNA-Peptide Conjugates by T7 RNA Polymerase. ACS Chem. Biol. 14 (12), 2564–2575. doi:10.1021/acschembio.9b00365
Johnson, D., Crawford, M., Cooper, T., Claeys Bouuaert, C., Keeney, S., Llorente, B., et al. (2021). Concerted Cutting by Spo11 Illuminates Meiotic DNA Break Mechanics. Nature 594 (7864), 572–576. doi:10.1038/s41586-021-03389-3
Keeney, S., Giroux, C. N., and Kleckner, N. (1997). Meiosis-specific DNA Double-Strand Breaks Are Catalyzed by Spo11, a Member of a Widely Conserved Protein Family. Cell 88 (3), 375–384. doi:10.1016/s0092-8674(00)81876-0
Kerzendorfer, C., Whibley, A., Carpenter, G., Outwin, E., Chiang, S.-C., Turner, G., et al. (2010). Mutations in Cullin 4B Result in a Human Syndrome Associated with Increased Camptothecin-Induced Topoisomerase I-dependent DNA Breaks. Hum. Mol. Genet. 19 (7), 1324–1334. doi:10.1093/hmg/ddq008
Kojima, Y., Machida, Y., Palani, S., Caulfield, T. R., Radisky, E. S., Kaufmann, S. H., et al. (2020). FAM111A Protects Replication Forks from Protein Obstacles via its Trypsin-like Domain. Nat. Commun. 11 (1), 1318. doi:10.1038/s41467-020-15170-7
Krastev, D. B., Li, S., Sun, Y., Wicks, A. J., Hoslett, G., Weekes, D., et al. (2022). The Ubiquitin-dependent ATPase P97 Removes Cytotoxic Trapped PARP1 from Chromatin. Nat. Cell Biol. 24 (1), 62–73. doi:10.1038/s41556-021-00807-6
Kröning, A., van den Boom, J., Kracht, M., Kueck, A. F., and Meyer, H. (2022). Ubiquitin-directed AAA+ ATPase P97/VCP Unfolds Stable Proteins Crosslinked to DNA for Proteolysis by SPRTN. J. Biol. Chem. 298 (6), 101976. doi:10.1016/j.jbc.2022.101976
Krüger, A., Bürkle, A., Hauser, K., and Mangerich, A. (2020). Real-time Monitoring of PARP1-dependent PARylation by ATR-FTIR Spectroscopy. Nat. Commun. 11 (1), 2174. doi:10.1038/s41467-020-15858-w
Kühbacher, U., and Duxin, J. P. (2020). How to Fix DNA-Protein Crosslinks. DNA Repair 94, 102924. doi:10.1016/j.dnarep.2020.102924
Kuo, H. K., Griffith, J. D., and Kreuzer, K. N. (2007). 5-Azacytidine-Induced Methyltransferase-DNA Adducts Block DNA ReplicationIn Vivo. Cancer Res. 67 (17), 8248–8254. doi:10.1158/0008-5472.CAN-07-1038
Langelier, M.-F., Planck, J. L., Roy, S., and Pascal, J. M. (2011). Crystal Structures of Poly(ADP-Ribose) Polymerase-1 (PARP-1) Zinc Fingers Bound to DNA. J. Biol. Chem. 286 (12), 10690–10701. doi:10.1074/jbc.M110.202507
Langelier, M.-F., Planck, J. L., Roy, S., and Pascal, J. M. (2012). Structural Basis for DNA Damage-dependent poly(ADP-Ribosyl)ation by Human PARP-1. Science 336 (6082), 728–732. doi:10.1126/science.1216338
Larsen, N. B., Gao, A. O., Sparks, J. L., Gallina, I., Wu, R. A., Mann, M., et al. (2019). Replication-Coupled DNA-Protein Crosslink Repair by SPRTN and the Proteasome in Xenopus Egg Extracts. Mol. Cell 73 (3), 574–588. doi:10.1016/j.molcel.2018.11.024
Lecona, E., Rodriguez-Acebes, S., Specks, J., Lopez-Contreras, A. J., Ruppen, I., Murga, M., et al. (2016). USP7 Is a SUMO Deubiquitinase Essential for DNA Replication. Nat. Struct. Mol. Biol. 23 (4), 270–277. doi:10.1038/nsmb.3185
Ledesma, F. C., El Khamisy, S. F., Zuma, M. C., Osborn, K., and Caldecott, K. W. (2009). A Human 5′-tyrosyl DNA Phosphodiesterase that Repairs Topoisomerase-Mediated DNA Damage. Nature 461 (7264), 674–678. doi:10.1038/nature08444
Li, F., Raczynska, J. E., Chen, Z., and Yu, H. (2019). Structural Insight into DNA-dependent Activation of Human Metalloprotease Spartan. Cell Rep. 26 (12), 3336–3346 e4. doi:10.1016/j.celrep.2019.02.082
Li, F., Zhang, Y., Bai, J., Greenberg, M. M., Xi, Z., and Zhou, C. (2017). 5-Formylcytosine Yields DNA-Protein Cross-Links in Nucleosome Core Particles. J. Am. Chem. Soc. 139 (31), 10617–10620. doi:10.1021/jacs.7b05495
Li, N., Wang, J., Wallace, S. S., Chen, J., Zhou, J., and D’Andrea, A. D. (2020). Cooperation of the NEIL3 and Fanconi Anemia/BRCA Pathways in Interstrand Crosslink Repair. Nucleic Acids Res. 48 (6), 3014–3028. doi:10.1093/nar/gkaa038
Lin, C.-P., Ban, Y., Lyu, Y. L., Desai, S. D., and Liu, L. F. (2008). A Ubiquitin-Proteasome Pathway for the Repair of Topoisomerase I-DNA Covalent Complexes. J. Biol. Chem. 283 (30), 21074–21083. doi:10.1074/jbc.M803493200
Liu, C.-W., Tian, X., Hartwell, H. J., Leng, J., Chi, L., Lu, K., et al. (2018). Accurate Measurement of Formaldehyde-Induced DNA-Protein Cross-Links by High-Resolution Orbitrap Mass Spectrometry. Chem. Res. Toxicol. 31 (5), 350–357. doi:10.1021/acs.chemrestox.8b00040
Liu, J. C. Y., Kühbacher, U., Larsen, N. B., Borgermann, N., Garvanska, D. H., Hendriks, I. A., et al. (2021). Mechanism and Function of DNA Replication‐independent DNA‐protein Crosslink Repair via the SUMO‐RNF4 Pathway. EMBO J. 40 (18), e107413. doi:10.15252/embj.2020107413
Liu, L. F., Duann, P., Lin, C.-T., D'Arpa, P., and Wu, J. (1996). Mechanism of Action of Camptothecin. Ann. N. Y. Acad. Sci. 803, 44–49. doi:10.1111/j.1749-6632.1996.tb26375.x
Lopez-Mosqueda, J., Maddi, K., Prgomet, S., Kalayil, S., Marinovic-Terzic, I., Terzic, J., et al. (2016). SPRTN Is a Mammalian DNA-Binding Metalloprotease that Resolves DNA-Protein Crosslinks. Elife 5, e21491. doi:10.7554/eLife.21491
Lu, K., Ye, W., Zhou, L., Collins, L. B., Chen, X., Gold, A., et al. (2010). Structural Characterization of Formaldehyde-Induced Cross-Links between Amino Acids and Deoxynucleosides and Their Oligomers. J. Am. Chem. Soc. 132 (10), 3388–3399. doi:10.1021/ja908282f
Luo, W., Li, H., Zhang, Y., and Ang, C. Y. W. (2001). Determination of Formaldehyde in Blood Plasma by High-Performance Liquid Chromatography with Fluorescence Detection. J. Chromatogr. B Biomed. Sci. Appl. 753 (2), 253–257. doi:10.1016/s0378-4347(00)00552-1
Ma, C.-H., Su, B.-Y., Maciaszek, A., Fan, H.-F., Guga, P., and Jayaram, M. (2019). A Flp-SUMO Hybrid Recombinase Reveals Multi-Layered Copy Number Control of a Selfish DNA Element through Post-translational Modification. PLoS Genet. 15 (6), e1008193. doi:10.1371/journal.pgen.1008193
Malanga, M., and Althaus, F. R. (2004). Poly(ADP-ribose) Reactivates Stalled DNA Topoisomerase I and Induces DNA Strand Break Resealing. J. Biol. Chem. 279 (7), 5244–5248. doi:10.1074/jbc.C300437200
Mao, Y., Desai, S. D., and Liu, L. F. (2000a). SUMO-1 Conjugation to Human DNA Topoisomerase II Isozymes. J. Biol. Chem. 275 (34), 26066–26073. doi:10.1074/jbc.M001831200
Mao, Y., Desai, S. D., Ting, C.-Y., Hwang, J., and Liu, L. F. (2001). 26 S Proteasome-Mediated Degradation of Topoisomerase II Cleavable Complexes. J. Biol. Chem. 276 (44), 40652–40658. doi:10.1074/jbc.M104009200
Mao, Y., Sun, M., Desai, S. D., and Liu, L. F. (2000b). SUMO-1 Conjugation to Topoisomerase I: A Possible Repair Response to Topoisomerase-Mediated DNA Damage. Proc. Natl. Acad. Sci. U.S.A. 97 (8), 4046–4051. doi:10.1073/pnas.080536597
Maskey, R. S., Flatten, K. S., Sieben, C. J., Peterson, K. L., Baker, D. J., Nam, H.-J., et al. (2017). Spartan Deficiency Causes Accumulation of Topoisomerase 1 Cleavage Complexes and Tumorigenesis. Nucleic Acids Res. 45 (8), 4564–4576. doi:10.1093/nar/gkx107
Maslov, A. Y., Lee, M., Gundry, M., Gravina, S., Strogonova, N., Tazearslan, C., et al. (2012). 5-Aza-2′-deoxycytidine-induced Genome Rearrangements Are Mediated by DNMT1. Oncogene 31 (50), 5172–5179. doi:10.1038/onc.2012.9
Mohni, K. N., Wessel, S. R., Zhao, R., Wojciechowski, A. C., Luzwick, J. W., Layden, H., et al. (2019). HMCES Maintains Genome Integrity by Shielding Abasic Sites in Single-Strand DNA. Cell 176 (1-2), 144–153 e13. doi:10.1016/j.cell.2018.10.055
Mórocz, M., Zsigmond, E., Tóth, R., Enyedi, M. Z., Pintér, L., and Haracska, L. (2017). DNA-dependent Protease Activity of Human Spartan Facilitates Replication of DNA-Protein Crosslink-Containing DNA. Nucleic Acids Res. 45 (6), 3172–3188. doi:10.1093/nar/gkw1315
Mulderrig, L., Garaycoechea, J. I., Tuong, Z. K., Millington, C. L., Dingler, F. A., Ferdinand, J. R., et al. (2021). Aldehyde-driven Transcriptional Stress Triggers an Anorexic DNA Damage Response. Nature 600 (7887), 158–163. doi:10.1038/s41586-021-04133-7
Mullen, J. R., Chen, C.-F., and Brill, S. J. (2010). Wss1 Is a SUMO-dependent Isopeptidase that Interacts Genetically with the Slx5-Slx8 SUMO-Targeted Ubiquitin Ligase. Mol. Cell Biol. 30 (15), 3737–3748. doi:10.1128/MCB.01649-09
Murai, J., and Pommier, Y. (2019). PARP Trapping beyond Homologous Recombination and Platinum Sensitivity in Cancers. Annu. Rev. Cancer Biol. 3, 131–150. doi:10.1146/annurev-cancerbio-030518-055914
Muthurajan, U. M., Hepler, M. R. D., Hieb, A. R., Clark, N. J., Kramer, M., Yao, T., et al. (2014). Automodification Switches PARP-1 Function from Chromatin Architectural Protein to Histone Chaperone. Proc. Natl. Acad. Sci. U.S.A. 111 (35), 12752–12757. doi:10.1073/pnas.1405005111
Nakamura, J., and Nakamura, M. (2020). DNA-protein Crosslink Formation by Endogenous Aldehydes and AP Sites. DNA Repair 88, 102806. doi:10.1016/j.dnarep.2020.102806
Nakano, T., Ouchi, R., Kawazoe, J., Pack, S. P., Makino, K., and Ide, H. (2012). T7 RNA Polymerases Backed up by Covalently Trapped Proteins Catalyze Highly Error Prone Transcription. J. Biol. Chem. 287 (9), 6562–6572. doi:10.1074/jbc.M111.318410
Narula, G., Annamalai, T., Aedo, S., Cheng, B., Sorokin, E., Wong, A., et al. (2011). The Strictly Conserved Arg-321 Residue in the Active Site of Escherichia coli Topoisomerase I Plays a Critical Role in DNA Rejoining. J. Biol. Chem. 286 (21), 18673–18680. doi:10.1074/jbc.M111.229450
Neale, M. J., Pan, J., and Keeney, S. (2005). Endonucleolytic Processing of Covalent Protein-Linked DNA Double-Strand Breaks. Nature 436 (7053), 1053–1057. doi:10.1038/nature03872
Nie, M., Moser, B. A., Nakamura, T. M., and Boddy, M. N. (2017). SUMO-targeted Ubiquitin Ligase Activity Can Either Suppress or Promote Genome Instability, Depending on the Nature of the DNA Lesion. PLoS Genet. 13 (5), e1006776. doi:10.1371/journal.pgen.1006776
Nie, M., Oravcová, M., Jami‐Alahmadi, Y., Wohlschlegel, J. A., Lazzerini‐Denchi, E., and Boddy, M. N. (2021). FAM111A Induces Nuclear Dysfunction in Disease and Viral Restriction. EMBO Rep. 22 (2), e50803. doi:10.15252/embr.202050803
Nielsen, C. F., Huttner, D., Bizard, A. H., Hirano, S., Li, T.-N., Palmai-Pallag, T., et al. (2015). PICH Promotes Sister Chromatid Disjunction and Co-operates with Topoisomerase II in Mitosis. Nat. Commun. 6, 8962. doi:10.1038/ncomms9962
Nielsen, I., Bentsen, I. B., Lisby, M., Hansen, S., Mundbjerg, K., Andersen, A. H., et al. (2009). A Flp-Nick System to Study Repair of a Single Protein-Bound Nick In Vivo. Nat. Methods 6 (10), 753–757. doi:10.1038/nmeth.1372
Olivieri, M., Cho, T., Álvarez-Quilón, A., Li, K., Schellenberg, M. J., Zimmermann, M., et al. (2020). A Genetic Map of the Response to DNA Damage in Human Cells. Cell 182 (2), 481–496. doi:10.1016/j.cell.2020.05.040
Park, S.-Y., and Cheng, Y.-C. (2005). Poly(ADP-ribose) Polymerase-1 Could Facilitate the Religation of Topoisomerase I-Linked DNA Inhibited by Camptothecin. Cancer Res. 65 (9), 3894–3902. doi:10.1158/0008-5472.CAN-04-4014
Perry, M., Biegert, M., Kollala, S. S., Mallard, H., Su, G., Kodavati, M., et al. (2021). USP11 Mediates Repair of DNA-Protein Cross-Links by Deubiquitinating SPRTN Metalloprotease. J. Biol. Chem. 296, 100396. doi:10.1016/j.jbc.2021.100396
Prasad, R., Horton, J. K., Chastain, P. D., Gassman, N. R., Freudenthal, B. D., Hou, E. W., et al. (2014). Suicidal Cross-Linking of PARP-1 to AP Site Intermediates in Cells Undergoing Base Excision Repair. Nucleic Acids Res. 42 (10), 6337–6351. doi:10.1093/nar/gku288
Prasad, R., Horton, J. K., Dai, D.-P., and Wilson, S. H. (2019). Repair Pathway for PARP-1 DNA-Protein Crosslinks. DNA Repair 73, 71–77. doi:10.1016/j.dnarep.2018.11.004
Prasad, R., Horton, J. K., and Wilson, S. H. (2020). Requirements for PARP-1 Covalent Crosslinking to DNA (PARP-1 DPC). DNA Repair 90, 102850. doi:10.1016/j.dnarep.2020.102850
Priego Moreno, S., Jones, R. M., Poovathumkadavil, D., Scaramuzza, S., and Gambus, A. (2019). Mitotic Replisome Disassembly Depends on TRAIP Ubiquitin Ligase Activity. Life Sci. Alliance 2 (2), e201900390. doi:10.26508/lsa.201900390
Prieler, S., Chen, D., Huang, L., Mayrhofer, E., Zsótér, S., Vesely, M., et al. (2021). Spo11 Generates Gaps through Concerted Cuts at Sites of Topological Stress. Nature 594 (7864), 577–582. doi:10.1038/s41586-021-03632-x
Prudden, J., Pebernard, S., Raffa, G., Slavin, D. A., Perry, J. J. P., Tainer, J. A., et al. (2007). SUMO-targeted Ubiquitin Ligases in Genome Stability. EMBO J. 26 (18), 4089–4101. doi:10.1038/sj.emboj.7601838
Quiñones, J. L., Thapar, U., Wilson, S. H., Ramsden, D. A., and Demple, B. (2020). Oxidative DNA-Protein Crosslinks Formed in Mammalian Cells by Abasic Site Lyases Involved in DNA Repair. DNA Repair 87, 102773. doi:10.1016/j.dnarep.2019.102773
Quiñones, J. L., Thapar, U., Yu, K., Fang, Q., Sobol, R. W., and Demple, B. (2015). Enzyme Mechanism-Based, Oxidative DNA-Protein Cross-Links Formed with DNA Polymerase β In Vivo. Proc. Natl. Acad. Sci. U.S.A. 112 (28), 8602–8607. doi:10.1073/pnas.1501101112
Reinking, H. K., Hofmann, K., and Stingele, J. (2020a). Function and Evolution of the DNA-Protein Crosslink Proteases Wss1 and SPRTN. DNA Repair 88, 102822. doi:10.1016/j.dnarep.2020.102822
Reinking, H. K., Kang, H.-S., Götz, M. J., Li, H.-Y., Kieser, A., Zhao, S., et al. (2020b). DNA Structure-specific Cleavage of DNA-Protein Crosslinks by the SPRTN Protease. Mol. Cell 80 (1), 102–113 e6. doi:10.1016/j.molcel.2020.08.003
Rudolph, J., Muthurajan, U. M., Palacio, M., Mahadevan, J., Roberts, G., Erbse, A. H., et al. (2021). The BRCT Domain of PARP1 Binds Intact DNA and Mediates Intrastrand Transfer. Mol. Cell 81 (24), 4994–5006. doi:10.1016/j.molcel.2021.11.014
Ruggiano, A., Vaz, B., Kilgas, S., Popović, M., Rodriguez-Berriguete, G., Singh, A. N., et al. (2021). The Protease SPRTN and SUMOylation Coordinate DNA-Protein Crosslink Repair to Prevent Genome Instability. Cell Rep. 37 (10), 110080. doi:10.1016/j.celrep.2021.110080
Saha, L. K., Murai, Y., Saha, S., Jo, U., Tsuda, M., Takeda, S., et al. (2021). Replication-dependent Cytotoxicity and Spartan-Mediated Repair of Trapped PARP1-DNA Complexes. Nucleic Acids Res. 49 (18), 10493–10506. doi:10.1093/nar/gkab777
Saha, S., Sun, Y., Huang, S.-y. N., Baechler, S. A., Pongor, L. S., Agama, K., et al. (2020). DNA and RNA Cleavage Complexes and Repair Pathway for TOP3B RNA- and DNA-Protein Crosslinks. Cell Rep. 33 (13), 108569. doi:10.1016/j.celrep.2020.108569
Santi, D. V., Norment, A., and Garrett, C. E. (1984). Covalent Bond Formation between a DNA-Cytosine Methyltransferase and DNA Containing 5-azacytosine. Proc. Natl. Acad. Sci. U.S.A. 81 (22), 6993–6997. doi:10.1073/pnas.81.22.6993
Schellenberg, M. J., Lieberman, J. A., Herrero-Ruiz, A., Butler, L. R., Williams, J. G., Muñoz-Cabello, A. M., et al. (2017). ZATT (ZNF451)-Mediated Resolution of Topoisomerase 2 DNA-Protein Cross-Links. Science 357(6358), 1412, 1416. doi:10.1126/science.aam6468
Semlow, D. R., MacKrell, V. A., and Walter, J. C. (2022). The HMCES DNA-Protein Cross-Link Functions as an Intermediate in DNA Interstrand Cross-Link Repair. Nat. Struct. Mol. Biol. 29 (5), 451–462. doi:10.1038/s41594-022-00764-0
Serbyn, N., Bagdiul, I., Noireterre, A., Michel, A. H., Suhandynata, R. T., Zhou, H., et al. (2021). SUMO Orchestrates Multiple Alternative DNA-Protein Crosslink Repair Pathways. Cell Rep. 37 (8), 110034. doi:10.1016/j.celrep.2021.110034
Serbyn, N., Noireterre, A., Bagdiul, I., Plank, M., Michel, A. H., Loewith, R., et al. (2020). The Aspartic Protease Ddi1 Contributes to DNA-Protein Crosslink Repair in Yeast. Mol. Cell 77 (5), 1066–1079 e9. doi:10.1016/j.molcel.2019.12.007
Shi, Y., Lan, F., Matson, C., Mulligan, P., Whetstine, J. R., Cole, P. A., et al. (2004). Histone Demethylation Mediated by the Nuclear Amine Oxidase Homolog LSD1. Cell 119 (7), 941–953. doi:10.1016/j.cell.2004.12.012
Shu, J., Cui, D., Ma, Y., Xiong, X., Sun, Y., and Zhao, Y. (2020). Scfβ-TrCP-mediated Degradation of TOP2β Promotes Cancer Cell Survival in Response to Chemotherapeutic Drugs Targeting Topoisomerase II. Oncogenesis 9 (2), 8. doi:10.1038/s41389-020-0196-1
Sonneville, R., Bhowmick, R., Hoffmann, S., Mailand, N., Hickson, I. D., and Labib, K. (2019). TRAIP Drives Replisome Disassembly and Mitotic DNA Repair Synthesis at Sites of Incomplete DNA Replication. Elife 8, e48686. doi:10.7554/eLife.48686
Sordet, O., Larochelle, S., Nicolas, E., Stevens, E. V., Zhang, C., Shokat, K. M., et al. (2008). Hyperphosphorylation of RNA Polymerase II in Response to Topoisomerase I Cleavage Complexes and its Association with Transcription- and BRCA1-dependent Degradation of Topoisomerase I. J. Mol. Biol. 381 (3), 540–549. doi:10.1016/j.jmb.2008.06.028
Sparks, J. L., Chistol, G., Gao, A. O., Räschle, M., Larsen, N. B., Mann, M., et al. (2019). The CMG Helicase Bypasses DNA-Protein Cross-Links to Facilitate Their Repair. Cell 176 (1-2), 167–181 e21. doi:10.1016/j.cell.2018.10.053
Steffen, J. D., McCauley, M. M., and Pascal, J. M. (2016). Fluorescent Sensors of PARP-1 Structural Dynamics and Allosteric Regulation in Response to DNA Damage. Nucleic Acids Res. 44 (20), 9771–9783. doi:10.1093/nar/gkw710
Steinacher, R., Osman, F., Lorenz, A., Bryer, C., and Whitby, M. C. (2013). Slx8 Removes Pli1-dependent Protein-SUMO Conjugates Including SUMOylated Topoisomerase I to Promote Genome Stability. PLoS One 8 (8), e71960. doi:10.1371/journal.pone.0071960
Stingele, J., Bellelli, R., Alte, F., Hewitt, G., Sarek, G., Maslen, S. L., et al. (2016). Mechanism and Regulation of DNA-Protein Crosslink Repair by the DNA-dependent Metalloprotease SPRTN. Mol. Cell 64 (4), 688–703. doi:10.1016/j.molcel.2016.09.031
Stingele, J., Bellelli, R., and Boulton, S. J. (2017). Mechanisms of DNA-Protein Crosslink Repair. Nat. Rev. Mol. Cell Biol. 18 (9), 563–573. doi:10.1038/nrm.2017.56
Stingele, J., and Jentsch, S. (2015). DNA-protein Crosslink Repair. Nat. Rev. Mol. Cell Biol. 16 (8), 455–460. doi:10.1038/nrm4015
Stingele, J., Schwarz, M. S., Bloemeke, N., Wolf, P. G., and Jentsch, S. (2014). A DNA-dependent Protease Involved in DNA-Protein Crosslink Repair. Cell 158 (2), 327–338. doi:10.1016/j.cell.2014.04.053
Sun, Y., Chen, J., Huang, S.-y. N., Su, Y. P., Wang, W., Agama, K., et al. (2021). PARylation Prevents the Proteasomal Degradation of Topoisomerase I DNA-Protein Crosslinks and Induces Their Deubiquitylation. Nat. Commun. 12 (1), 5010. doi:10.1038/s41467-021-25252-9
Sun, Y., Miller Jenkins, L. M., Su, Y. P., Nitiss, K. C., Nitiss, J. L., and Pommier, Y. (2020). A Conserved SUMO Pathway Repairs Topoisomerase DNA-Protein Cross-Links by Engaging Ubiquitin-Mediated Proteasomal Degradation. Sci. Adv. 6 (46), eaba6290. doi:10.1126/sciadv.aba6290
Tayri-Wilk, T., Slavin, M., Zamel, J., Blass, A., Cohen, S., Motzik, A., et al. (2020). Mass Spectrometry Reveals the Chemistry of Formaldehyde Cross-Linking in Structured Proteins. Nat. Commun. 11 (1), 3128. doi:10.1038/s41467-020-16935-w
Thompson, P. S., Amidon, K. M., Mohni, K. N., Cortez, D., and Eichman, B. F. (2019). Protection of Abasic Sites during DNA Replication by a Stable Thiazolidine Protein-DNA Cross-Link. Nat. Struct. Mol. Biol. 26 (7), 613–618. doi:10.1038/s41594-019-0255-5
Tian, T., Bu, M., Chen, X., Ding, L., Yang, Y., Han, J., et al. (2021). The ZATT-Top2a-PICH Axis Drives Extensive Replication Fork Reversal to Promote Genome Stability. Mol. Cell 81 (1), 198–211 e6. doi:10.1016/j.molcel.2020.11.007
Topcu, Z. (2001). DNA Topoisomerases as Targets for Anticancer Drugs. J. Clin. Pharm. Ther. 26 (6), 405–416. doi:10.1046/j.1365-2710.2001.00368.x
Tretyakova, N. Y., Groehler, A., and Ji, S. (2015). DNA-protein Cross-Links: Formation, Structural Identities, and Biological Outcomes. Acc. Chem. Res. 48 (6), 1631–1644. doi:10.1021/acs.accounts.5b00056
Vaz, B., Popovic, M., Newman, J. A., Fielden, J., Aitkenhead, H., Halder, S., et al. (2016). Metalloprotease SPRTN/DVC1 Orchestrates Replication-Coupled DNA-Protein Crosslink Repair. Mol. Cell 64 (4), 704–719. doi:10.1016/j.molcel.2016.09.032
Vaz, B., Popovic, M., and Ramadan, K. (2017). DNA-protein Crosslink Proteolysis Repair. Trends Biochem. Sci. 42 (6), 483–495. doi:10.1016/j.tibs.2017.03.005
Wei, Y., Diao, L.-X., Lu, S., Wang, H.-T., Suo, F., Dong, M.-Q., et al. (2017). SUMO-Targeted DNA Translocase Rrp2 Protects the Genome from Top2-Induced DNA Damage. Mol. Cell 66 (5), 581–596 e6. doi:10.1016/j.molcel.2017.04.017
Wu, R. A., Semlow, D. R., Kamimae-Lanning, A. N., Kochenova, O. V., Chistol, G., Hodskinson, M. R., et al. (2019). TRAIP Is a Master Regulator of DNA Interstrand Crosslink Repair. Nature 567 (7747), 267–272. doi:10.1038/s41586-019-1002-0
Yang, K., Park, D., Tretyakova, N. Y., and Greenberg, M. M. (2018). Histone Tails Decrease N7-Methyl-2′-Deoxyguanosine Depurination and Yield DNA-Protein Cross-Links in Nucleosome Core Particles and Cells. Proc. Natl. Acad. Sci. U.S.A. 115 (48), E11212–E11220. doi:10.1073/pnas.1813338115
Yang, K., Sun, H., Lowder, L., Varadarajan, S., and Greenberg, M. M. (2019). Reactivity of N3-Methyl-2′-Deoxyadenosine in Nucleosome Core Particles. Chem. Res. Toxicol. 32 (10), 2118–2124. doi:10.1021/acs.chemrestox.9b00299
Yang, S. W., Burgin, A. B., Huizenga, B. N., Robertson, C. A., Yao, K. C., and Nash, H. A. (1996). A Eukaryotic Enzyme that Can Disjoin Dead-End Covalent Complexes between DNA and Type I Topoisomerases. Proc. Natl. Acad. Sci. U.S.A. 93 (21), 11534–11539. doi:10.1073/pnas.93.21.11534
Yip, M. C. J., Bodnar, N. O., and Rapoport, T. A. (2020). Ddi1 Is a Ubiquitin-dependent Protease. Proc. Natl. Acad. Sci. U.S.A. 117 (14), 7776–7781. doi:10.1073/pnas.1902298117
Yung, T. M. C., Sato, S., and Satoh, M. S. (2004). Poly(ADP-ribosyl)ation as a DNA Damage-Induced Post-translational Modification Regulating poly(ADP-Ribose) Polymerase-1-Topoisomerase I Interaction. J. Biol. Chem. 279 (38), 39686–39696. doi:10.1074/jbc.M402729200
Zhang, H.-F., Tomida, A., Koshimizu, R., Ogiso, Y., Lei, S., and Tsuruo, T. (2004). Cullin 3 Promotes Proteasomal Degradation of the Topoisomerase I-DNA Covalent Complex. Cancer Res. 64 (3), 1114–1121. doi:10.1158/0008-5472.can-03-2858
Keywords: DNA-protein crosslink (DPC), post-translational modifications (PTMs), ubiquitylation, small ubiquitin-related modifier (SUMO), poly(ADP-ribosyl)ation, DNA replication, DNA repair
Citation: Leng X and Duxin JP (2022) Targeting DNA-Protein Crosslinks via Post-Translational Modifications. Front. Mol. Biosci. 9:944775. doi: 10.3389/fmolb.2022.944775
Received: 15 May 2022; Accepted: 03 June 2022;
Published: 04 July 2022.
Edited by:
Yilun Sun, National Institutes of Health (NIH), United StatesReviewed by:
Julian Stingele, Ludwig Maximilian University of Munich, GermanySourav Saha, National Cancer Institute (NIH), United States
Copyright © 2022 Leng and Duxin. This is an open-access article distributed under the terms of the Creative Commons Attribution License (CC BY). The use, distribution or reproduction in other forums is permitted, provided the original author(s) and the copyright owner(s) are credited and that the original publication in this journal is cited, in accordance with accepted academic practice. No use, distribution or reproduction is permitted which does not comply with these terms.
*Correspondence: Julien P. Duxin, julien.duxin@cpr.ku.dk