- 1Department of Bio-Resources, School of Biological Sciences, University of Kashmir, Srinagar, India
- 2Department of Biotechnology, School of Life Sciences, Central University of Kashmir, Ganderbal, India
- 3Department of Life Sciences, National Institute of Technology (NIT), Rourkela, Odisha, India
- 4Department of Family and Community Medicine, Faculty of Medicine, Al Baha University, Al Bahah, Saudi Arabia
- 5Department of Biology, College of Science, Hafr Al Batin University of Hafr Al-Batin, Hafar Al Batin, Saudi Arabia
Antibiotic resistance development and pathogen cross-dissemination are both considered essential risks to human health on a worldwide scale. Antimicrobial resistance genes (AMRs) are acquired, expressed, disseminated, and traded mainly through integrons, the key players capable of transferring genes from bacterial chromosomes to plasmids and their integration by integrase to the target pathogenic host. Moreover, integrons play a central role in disseminating and assembling genes connected with antibiotic resistance in pathogenic and commensal bacterial species. They exhibit a large and concealed diversity in the natural environment, raising concerns about their potential for comprehensive application in bacterial adaptation. They should be viewed as a dangerous pool of resistance determinants from the “One Health approach.” Among the three documented classes of integrons reported viz., class-1, 2, and 3, class 1 has been found frequently associated with AMRs in humans and is a critical genetic element to serve as a target for therapeutics to AMRs through gene silencing or combinatorial therapies. The direct method of screening gene cassettes linked to pathogenesis and resistance harbored by integrons is a novel way to assess human health. In the last decade, they have witnessed surveying the integron-associated gene cassettes associated with increased drug tolerance and rising pathogenicity of human pathogenic microbes. Consequently, we aimed to unravel the structure and functions of integrons and their integration mechanism by understanding horizontal gene transfer from one trophic group to another. Many updates for the gene cassettes harbored by integrons related to resistance and pathogenicity are extensively explored. Additionally, an updated account of the assessment of AMRs and prevailing antibiotic resistance by integrons in humans is grossly detailed—lastly, the estimation of AMR dissemination by employing integrons as potential biomarkers are also highlighted. The current review on integrons will pave the way to clinical understanding for devising a roadmap solution to AMR and pathogenicity.
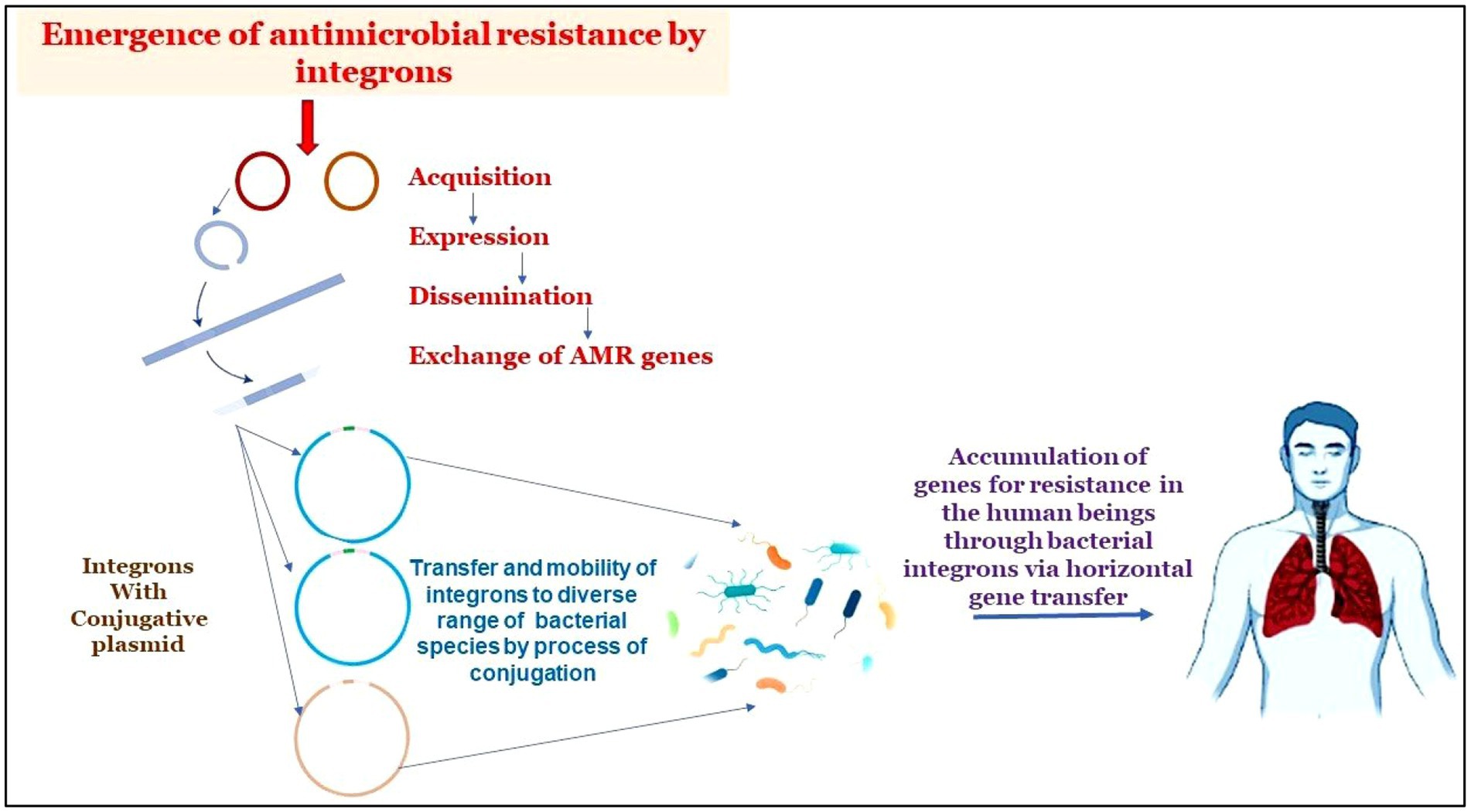
Graphical Abstract. The graphical abstract displays how integron-aided AMRs to humans: Transposons capture integron gene cassettes to yield high mobility integrons that target res sites of plasmids. These plasmids, in turn, promote the mobility of acquired integrons into diverse bacterial species. The acquisitions of resistant genes are transferred to humans through horizontal gene transfer.
Highlights
- We highlighted the detailed molecular mechanism mediated by integrons to serve as natural cloning and expression vectors to disseminate antimicrobial resistance genes in various microbial species.
- The review emphasizes highlighting integrons as genetic markers for estimating AMR existing in microbial species and their existence in humans.
- The current study underlines the worldwide risk to human health posed by integron-mediated antibiotic-resistance gene cassettes and their transmission to humans.
- The review highlights the spread of AMR through integrons as a global issue. Additionally, we raised concerns about devising strategies to hinder the resistance mechanisms evolved by microbes through the aid of integrons.
Introduction
The mounting threat posed by antimicrobial resistance and engraved pathogenicity on humans forced scientific communities to profoundly investigate the evolution of resistance mechanisms and their responsible agents (Hamdani et al., 2020; Larsson and Flach, 2022; Sheikh et al., 2022). Antibiotic abuse and overuse have resulted in the formation and dissemination of antibiotic-resistance genes (ARGs) and antibiotic-resistant bacteria (ARBs), causing widespread worry around the world in human medicine and livestock breeding (Serwecińska, 2020; Mir et al., 2021; Sheikh et al., 2021b). Since ARGs are commonly found in domestic sewage, mud/dirt, and animal waste in these environments, these ecosystems are considered key reservoirs for ARGs (Wellington et al., 2013; Ding et al., 2019) to many bacterial species, particularly to those causing disease. The ultimate, well-known effects of such accumulated evolutionary events are gradually growing challenges in preventing and treating bacterial diseases. Understanding and recognizing human, animal, and environmental microbiota relationships is crucial because bacteria and genes frequently cross habitats and species boundaries “One Health Concept” (Collignon, 2012; Zinsstag et al., 2012; So et al., 2015; Torren-Edo et al., 2015; World Health Organization, 2015; Collignon and McEwen, 2019) to manage this global health challenge (Wellington et al., 2013; Berendonk et al., 2015; Bengtsson-Palme et al., 2018). Antimicrobial resistance is an ecological issue that affects the wellbeing of people, animals, and the environment. It is characterized by complex interactions involving many microbial populations (Fletcher, 2015; Collignon and McEwen, 2019).
The establishment and prevalence of antimicrobial resistance are caused by various resistance mechanisms, which may be divided into genetic mutation occurring at a low frequency and acquisition of different genes mediated resistance to their host microbes. As a result, it has been determined that acquiring resistance genes is a significant factor in antibiotic resistance’s widespread distribution and spread. The resistance is accomplished through vertical or horizontal transfer, with the latter method involving mobile genetic elements like plasmids and transposons (Xu et al., 2011b). Integrons are primarily carried by plasmids or contained within transposons, and their mechanism and function in the movement of microorganisms are well-known and documented (Hall and Collis, 1995; Mazel, 2006), which had also been considered to contribute to the unleashing of “Super Bugs” (Xu et al., 2011b,c).
The exchange of genetic material between bacteria of the same generation is known as horizontal gene transfer (HGT). The heredity of the transferred sequences in the recipient microbe is also crucial for a successful HGT event, in addition to the entry of DNA into the cytoplasm of the recipient cell (Ochman et al., 2000). HGT influences bacterial population’s genetic diversity and evolutionary trajectories. For instance, the main factor causing the establishment, recombination, and spread of multidrug resistance among bacterial pathogens is the HGT of mobile genetic elements (MGEs) (Nakamura et al., 2004; Thomas and Nielsen, 2005). MGEs are widespread in bacterial populations due to their ability to physically migrate between host genomes. MGEs include plasmids, bacteriophages, genomic islands (GIs), transposons (Tns), integrons, insertion sequences (ISs), integrative and conjugative elements (ICEs), and tiny inverted-repeat transposable elements, among others (MITEs) (Stokes and Gillings, 2011).
Mobile genetic elements (GEs), such as integrons, may be critical in transferring ARGs across microbial organisms in their surroundings. Integrons may extract ARGs from their surroundings and subsequently incorporate them into their gene cassettes via location-specific recombination (Zhang et al., 2009; Sheikh et al., 2021a). Integrons are thus crucial in developing antibiotic tolerance and the horizontal gene transfer (HGT) of ARGs between bacterial organisms in diverse settings. The discovery of mobile integrons as critical players in the acquisition, mobilization, shuffle, and initiation of expression of gene cassettes, especially those encoding antibiotic resistance (Domingues et al., 2012; Escudero et al., 2015). The genetic elements known as integrons have a location-specific recombination system that enables them to acquire, express, and transfer particular DNA fragments known as gene cassettes (Hall and Collis, 1995).
Integrons are genetic elements that contain a site-specific recombination system able to integrate, express and exchange specific DNA elements, called gene cassettes (Hall and Collis, 1995). The integron is not regarded as a mobile element because it lacks mobility-related functions. In contrast, the gene cassettes contained in integrons are regarded as mobile, even though the spontaneous exchange of gene cassettes is rarely observed experimentally (Guerin et al., 2009; Baharoglu et al., 2010). Nevertheless, sequence-similar integrons appear widespread among bacterial species and genetic backgrounds, suggesting that they are frequently exposed to mechanisms that allow them to disseminate horizontally through bacterial populations (Stokes and Hall, 1989).
The present review article aimed to update the account of gene cassettes harbored by integrons on resistance and pathogenicity and searched to assess existing AMRs and antibiotic resistance by integrons in humans. Lastly, estimating AMR dissemination by employing integrons as potential biomarkers is also highlighted to have an in-depth role in global AMR dissemination. We believe this review will help to understand better how to develop a plan to minimize the global phenomenon of increasing antimicrobial resistance and pathogenicity related to human pathogenic microbes.
Integrons
An integron is generally defined by the presence of an integrase gene (intI) and a proximal primary recombination site (attI) (Figure 1; Partridge et al., 2009; Xu et al., 2011a). Structurally, all integrons consist of three main components, including 5′ and 3′ conserved segment and a central variable region between the 5′ and 3′ zone, in which integrons are responsible for the capture and expression of exogenous genes, which are part of the gene cassette. Integrons are accountable for capturing and expressing the exogenous genes that form the gene cassette in this central variable region (Mazel, 2006). The first component of the integron is a gene which produces a tyrosine recombinase (integrase, encoded by the intI gene), required for site-specific recombination inside the integron. The second component is an adjacent recombination site (attI), which the integrase recognizes. The third component is a promoter (Pc), which is situated upstream of the integration and required for the effective process of transcription and expression (Mazel, 2006; Labbate et al., 2009). The promoter aids in the expression of any integrated gene(s). The tyrosine-specific recombinase family, which also comprises the well-known integrase of the λ-phage, includes integrase (IntI) (Grainge and Jayaram, 1999; Carattoli, 2001).
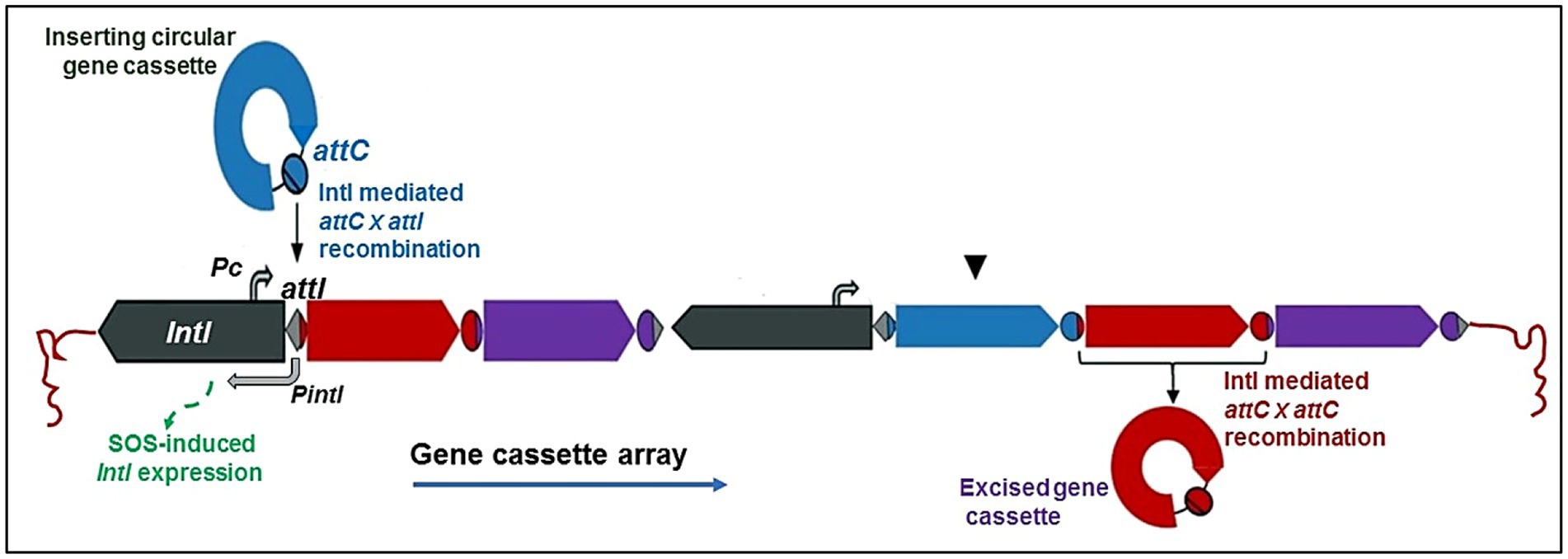
Figure 1. Schematic representation of a class 1 integron. The basic integron platform consists of the following: intI, a gene for the integron integrase; Pc, an integron-carried promoter; attI, the integron-associated recombination site; and gene cassettes, sequentially inserted into an array via recombination between attI and the cassette associated-recombination sites, attC (Gillings, 2014; Ghaly et al., 2020a).
These three components are simple structures with a single open reading frame (ORF) surrounded by a cassette-associated recombination site. They were initially called 59-base elements but are now referred to as attC (Gillings, 2014). While the Pc promoter is believed to be present and active in all forms of integrons, this has not been demonstrated for all of them (Ghaly et al., 2021a). Numerous integrase genes found in integrons have LexA binding sites close to their promoter regions allowing them to be regulated by the host LexA protein, which functions as a transcriptional repressor of the SOS response; as demonstrated for a class 1 integrase and in an integrase present in a CI (chromosomal integron) of Vibrio cholerae (Guerin et al., 2009; Baharoglu et al., 2010). These findings show that SOS induction can result in increased integrase gene transcription and activity and cassette rearrangements in organisms possessing lexA alleles (Baharoglu et al., 2010) The modulation of integrase transcription is unknown without a LexA ortholog. Integrons might have a single gene cassette or many of them (Hall and Collis, 1995). In most clinical bacterial strains, integrons have no more than 5 cassettes (Bennett, 2008), but integrons containing at least 9 genes for antibiotic tolerance have also been found. (Naas et al., 2001). The INTEGRAL database1 containing a list of numbered C1 integrons is available (Moura et al., 2009).
Initially, integrons were grouped into a few categories based on their genetic similarity to the integrase intI gene sequence, with classes 1, 2 and 3 receiving major attention (Nield et al., 2001; Domingues et al., 2012). Nevertheless, more DNA sequencing-based research have revealed more of the integrase gene’s genetic diversity and discovered over 90 distinct gene variations, questioning the initial classification system in doubt (Gillings et al., 2008; Domingues et al., 2012). Class 4 integron was discovered later in a relationship with chromosomal integrons. IntI encodes an integrase (IntI) of the tyrosine recombinase family, which is distinguished by the presence of invariant RHRY (with Y being the catalytic tyrosine) amino-acids in the conserved motifs named box 1 and box 2 (which distinguish intI from other XerC-related integrases) (Xu et al., 2011a). IntI-catalyzed recombination between attI and/or attC sites results in the insertion or excision of cassettes (Figure 1). The class 1 integrase (IntI1) recognizes three types of recombination site: attI1, attC and secondary sites. Binding domains and consensus sequences have been determined for these. The attI1 site is a simple site which contains two inverted sequences that bind the integrase and two additional integrase-binding sites known as strong (DR1) and weak (DR2) (Francia et al., 1999; Hall et al., 1999).
Further, Integrons are categorized into 3 primary categories based on their phylogenetic analyzes of sequenced intI genes: the marine γ-proteobacteria group, the soil/freshwater proteobacteria group, and the inverted integrase group (Domingues et al., 2012). C1 integrons are the most common and pervasive, especially in clinical environments, and significantly contribute to the development of antibiotic tolerance (Amin et al., 2021). Although the origin of C1 integron is unknown, it is hypothesized to have existed in different bacterial species before the “antibiotic age (Stokes et al., 2006).” C1 integrons are present in the environment without antibiotic-resistant genes. The initial source of these genomic structures has been speculated to be beta proteobacteria. Tn402 (also known as Tn5090) characteristic features are absent in such types of environmental integrons suggesting that they were integrated into a plasmid-borne Tn402 transposon carrying a gene cassette imparting host advantage (Gillings et al., 2008). For instance, the qac gene, which imparts resistance to quaternary ammonium compounds and thus biocides, has a long history of use in clinical usage; and the su1I gene, confers resistance to sulphonamides. The early association of the Tn402 transposon’s progenitor with a class 1 integrase and an attI1 site is another possible origin of the C1 integrons. Most C1 integrons have the 5’-CS region in the same location (Toleman and Walsh, 2011). The 3’-CS region is thought to be the result of a fusion of the Tn402 transposon’s qacE gene with the su1I gene, followed by partial deletion of the qacE gene; this fusion occurred simultaneously with a deletion event in the Tn402 transposon’s transposition functions, resulting in a structure incapable of self-mobilization (Toleman and Walsh, 2011). Although a few C1 integrons with a complete transposition module have been found, the bulk of C1 integrons are transposons with incomplete transposition modules (Juan et al., 2010; Marchiaro et al., 2010). After the initial generation of C1 integrons, intense selection for antimicrobial tolerance favored the detection of antibiotic tolerance gene cassettes giving rise to the C1 integron components that are familiar to us today (Bennett, 2008; Stokes and Gillings, 2011).
Integrons are further categorized according to their environment, with two distinct types: mobile integrons (MIs) and chromosomal integrons (CIs) (Liebert et al., 1999). CIs are composed of a variable number of gene cassettes between zero and hundreds, the majority of which do not contribute to antimicrobial drug tolerance. Although migration of gene cassettes from CIs has been recorded, CIs are considered sedentary (Liebert et al., 1999; Domingues et al., 2012). In comparison, MIs like C1 integrons carry a restricted number of gene cassettes and are frequently engaged in antimicrobial tolerance propagation (Domingues et al., 2012).
attC SITES
The attC domain consists of 2 simple sites, R′′ and R′, L′ and L′′, respectively, each made up of two conserved “core sites” (7 or 8 bp) (Bouvier et al., 2005). The RH consensus sequence has several connections to the RH simple site and includes the R′ and R′′ sites. Similarly, the L′ and L′′ parts of the LH consensus sequence are structurally and functionally similar to the LH. The integrase’s ability to distinguish among the LH and RH sites in the attC could describe the position of integrated gene cassettes. Additionally, it looks as though L′′ is more significant in terms of orientation (Bouvier et al., 2005). In addition to being necessary for orientation, the LH simple site promotes RH activity. (Partridge et al., 2009). A framework known as a gene cassette, typically not detectable during integrations but becomes an essential part of the integron once integrated, connects the attC sites to a single ORF in most cases (Deng et al., 2015).
Gene cassettes
Gene cassettes are small movable components carrying a single gene, typically without a promoter or recombination site (attC). Gene cassettes are linear when integrated into the C1 integron but circular when left unintegrated or before site-specific insertion (Domingues et al., 2012). They can appear as a separate circular DNA molecule that cannot be maintained stable throughout cell division or as a linear DNA molecules formed when the free circular element is inserted into the integron in a particular orientation (Mazel, 2006). Prior research has shown that the structural nature of integrons usually lacks cassettes in the variable area (Deng et al., 2015).
Gene cassettes generally lack promoters, although having a coding sequence, which acts as the system’s mobile component, and the majority of cassettes encode resistance to a wide variety of antibiotics, with over 130 different antibiotic resistance genes identified to date through distinctive attC sites (Hall and Collis, 1995). Most antibiotic families, such as -lactams, rifampicin, etc., and antiseptics from the quaternary ammonium compound family, are resistant to such cassettes (Hall and Collis, 1995).
Site-specific insertion of gene cassettes into the integron
The integration of circular gene cassettes occurs by site-specific recombination between attI and attC, assisted by the integron integrase. The first resident gene cassette’s 5′ end should be the target of insertion. The attI site in the 5’-CS conserved segment of the integron and the attC site in the 3’-CS conserved segment of the integron are the two key recombination sites where the integrase interacts with each gene cassette. For four sites inside the attI site, IntI has a high binding affinity (Partridge et al., 2018). It also weakly binds to the cassette’s attC site (Holmes et al., 2003). This procedure is reversible, and cassettes can be removed as free circular DNA elements (Johansson, 2007). Insertion at the attI site enables the expression of an incoming cassette driven by the neighboring Pc promoter (Razavi et al., 2017). As a tool for genomic innovation, the integron system offers two significant benefits. The bacterial genome initially incorporates additional genetic material through a specialized recombination site (attI) that inhibits the breakdown of existing genes. Secondly, the integron promoter (Pc), via which the freshly integrated gene is expressed, triggers the beginning of natural selection. Any newly generated variants will consequently instantly activate genes resulting in beneficial phenotypes in a population of cells with integrons, each with a unique gene cassette (Michael et al., 2004).An additional site in front of a gene not formerly present on cassettes may result in site-specific recombination between the attI and this secondary site, trapping the gene. The acquisition of the attC site, which is most likely achieved by a second stage of recombination from an existing gene cassette, is required to create a full cassette containing the new gene. Almost any gene may become an integron, although secondary-site recombination is uncommon and less effective than primary-site recombination. Integrons are further classified into two classes based on the genomic context of those genes: chromosomal integrons, which are found inside the bacterial chromosome, and mobile integrons, which are associated to transposons (Antelo et al., 2021; Sandoval-Quintana et al., 2022).
Mobility of integron: spreading resistance genes on a global scale
Integrons have been reported to be widespread and found across clinical species, and their movement has been cited as a primary source of concern for clinical antibiotic resistance. Integrons are connected with mobile DNA elements (transposons or plasmids) and antibiotic-tolerance genes, despite their small array dimensions and considerable heterogeneity in the sequence of attC sites (Verraes et al., 2013; Partridge et al., 2018). Although self-transposition is defective, existing integrons (mainly class 1 integrons) were thought to represent a potentially mobile genetic element. They’re typically seen on plasmids to aid conjugative transfer. They include mobile gene cassettes that can move to other integrons or secondary locations in the bacterial genome (Figure 2). Integrons may carry and propagate antibiotic tolerance genes, making them one of the most significant horizontal transfer pathways for transmitting tolerance/resistance genes across bacteria.
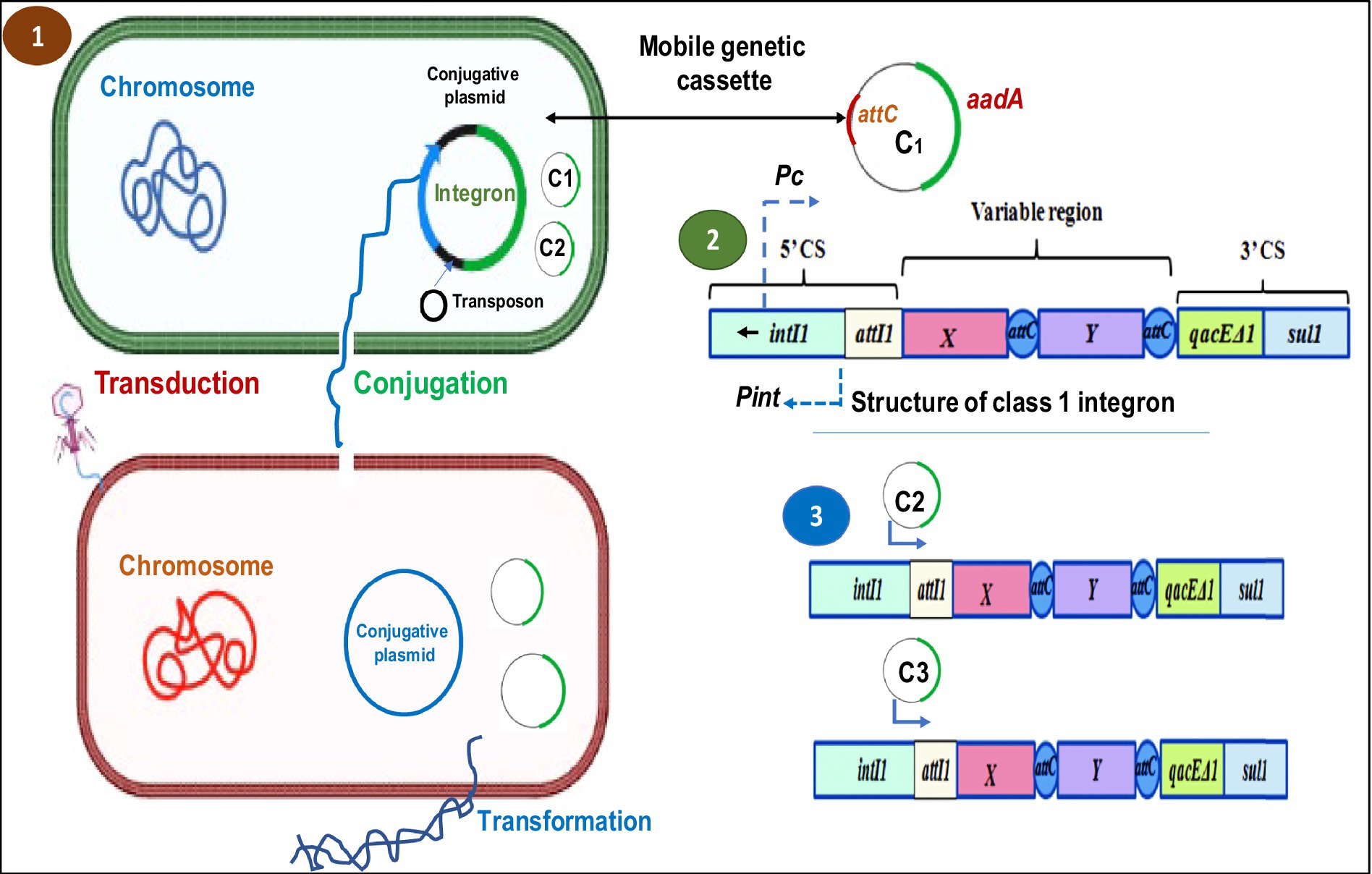
Figure 2. Schematic representation of HGT mechanisms in bacteria and the general structure of an integron and gene cassette. (1) HGT mechanism (conjugation, transduction and transformation) in bacteria. (2) The genetic organization of the class 1 integron DNA sequence includes the integrase gene (IntI) and one cassette gene. While the cassette array is expressed from the PC promoter, the integrase is expressed from the Pint promoter. (3) The orientation of insertion of the following genomic cassettes (C2, C3) into the integron structure. It is believed that as a gene is moved further away from the PC promoter, its expression level within the cassettes will decrease (Racewicz et al., 2020).
Integrons are a naturally occurring system of capturing and construction enabling microbes to incorporate gene cassettes and then correctly express them to transform them into functional proteins. Gene cassettes can be formed from various ORFs, and it is essential to understand how this process works. To allow for quick adaptability to selection pressure and hence boost the host’s overall fitness and advantage, integrons may be able to interchange and stockpile functioning gene cassettes forever (Yu et al., 2013; Deng et al., 2015). Frequent gene cassette arrays in various integron types and their impact on antimicrobial tolerance in various species of bacteria is depicted in Table 1.
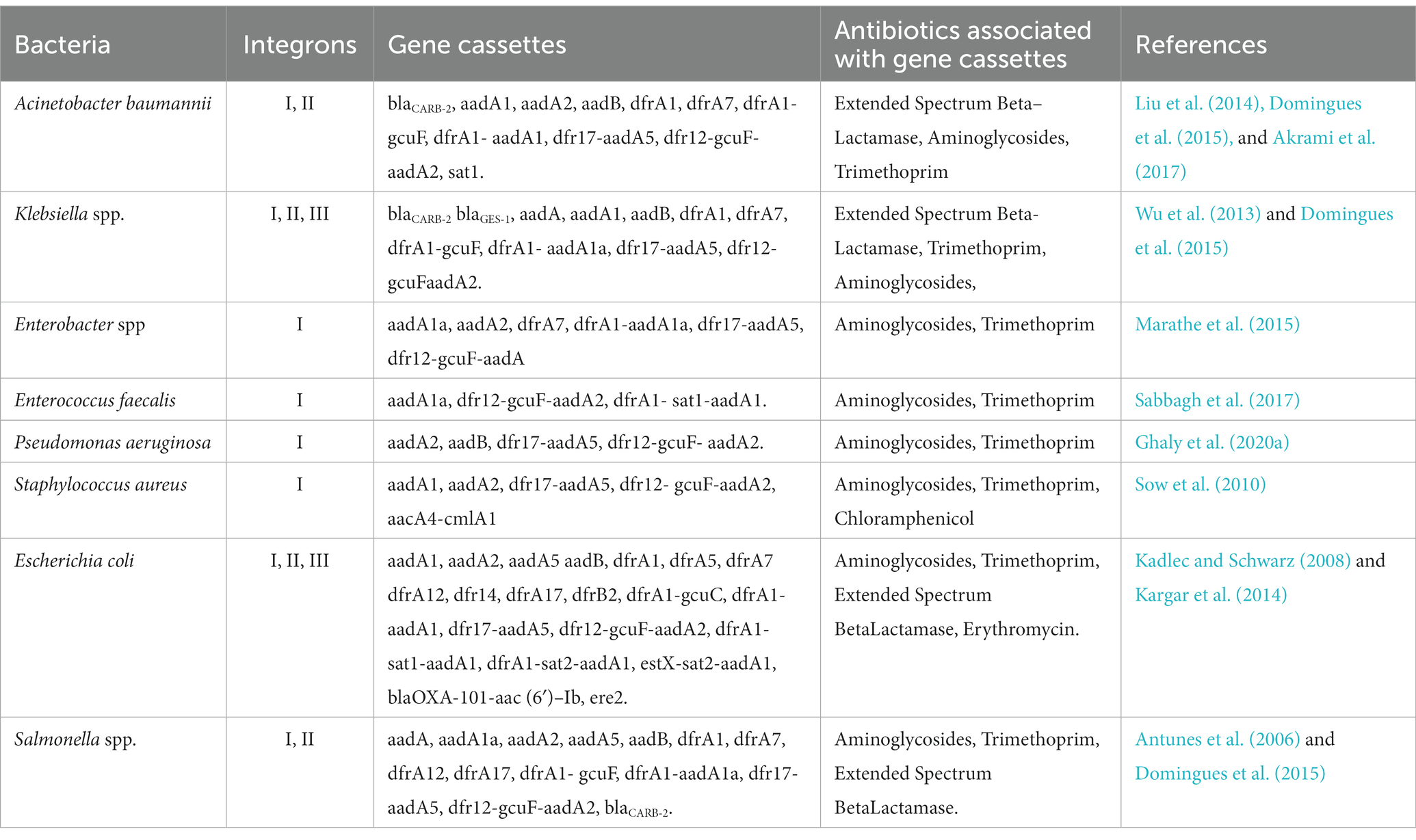
Table 1. Frequent gene cassette arrays in various integron types and their impact on antimicrobial tolerance in distinct species of bacteria (Sabbagh et al., 2021).
Furthermore, several reservoirs and genetic pools for integron, shared between bacteria, may be provided by mobile genetic elements, e.g., transposons, insertion sequences, etc. (Partridge et al., 2018). Integrons are essential for the spread and dispersion of genes associated with resistance. They transfer resistance genes to various drugs between bacteria (Deng et al., 2015). According to numerous findings on integrons from environmental microorganisms, including the high sequence diversity seen and the numerous functional products other than tolerance coded by these cassettes, integrons may have performed significantly in development and expansion for an extended period (Rendueles et al., 2018).
Horizontal gene transfer and integrons
The primary mechanism by which antibiotic resistance spreads is horizontal genetic transfer (Sundström, 1998). Antibiotic resistance genes can also be transmitted via a variety of other mechanisms. One of the most significant forces influencing the development of microorganisms is horizontal gene transfer, or the acquiring of foreign deoxyribonucleic acid by microbes (Il’ina, 2003). It frequently causes the emergence of antibiotic tolerance (Huddleston, 2014; Ma et al., 2017). In clinical practice, most antimicrobial drugs come from or are derived from substances naturally found in the environment, primarily soil. Bacteria in the same domain as these compounds can pick up environmental genes for antibiotic tolerance. There is compelling evidence that this “environmental resistome” can be a rich source for acquiring antibiotic-tolerance genes in bacteria that are relevant to human health (Munita and Arias, 2016). This exchange of genetic material has been particularly linked to the spread of antibiotic tolerance to several widely used antibiotic drugs. The traditional methods by which bacteria acquire external genetic material are (i) transformation (the integration of bare DNA) (Venema, 1979; Johnston et al., 2014), (ii) transduction (mediated by phages) (Ozeki and Ikeda, 1968), and (iii) conjugation (Curtiss Iii, 1969). HGT, which involves transformation, is by far the most straightforward. However, only a few clinically significant bacterial species can “naturally” incorporate bare DNA to evolve antibiotic tolerance. Conjugation, a reliable method of DNA transfer that includes cell-to-cell contact and is expected to happen often in the human intestinal tract undergoing antibiotic therapy, is a prevalent reason for tolerance in the hospital setting. Although direct chromosome-to-chromosome transfer has been widely characterized, conjugation typically uses Mobile Genetic Components (MGEs) to convey important genetic information (Nwosu, 2001). Plasmids and transposons are the two most important MGEs because they substantially contribute to developing and expanding antibiotic resistance in therapeutically relevant organisms. The most effective methods for assembling antimicrobial tolerance genes are integrons, site-specific recombination systems that can attract open reading frames within mobile gene cassettes. Integrons offer a powerful and relatively straightforward method for adding novel genes to bacterial chromosomes and the tools needed to monitor their expression. They also promote beneficial genetic exchange and are a significant force in the development of bacteria (Munita and Arias, 2016).
Integrons are a type of genetic component which allow exogenous genes to be efficiently captured and expressed and transfer the resistance genes through transposons and plasmids into bacterial species, which can inhabit humans (Figure 3). Their contribution to the antibiotic resistance expansion, particularly among bacteria, is well established. (Boucher et al., 2007). However, it is clear that integrons have a long evolutionary history dating back to their discovery in clinical settings and are a typical component of bacterial genomes. Integrons have been found in many habitats, can transfer between different species and lineages throughout evolutionary history, and can access a sizable number of new genes whose roles are primarily unknown. Integrons are much more significant than just being a characteristic of infections resistant to antibiotics, as evidenced by their function in bacterial adaptability and genome development in natural settings (Boucher et al., 2007).
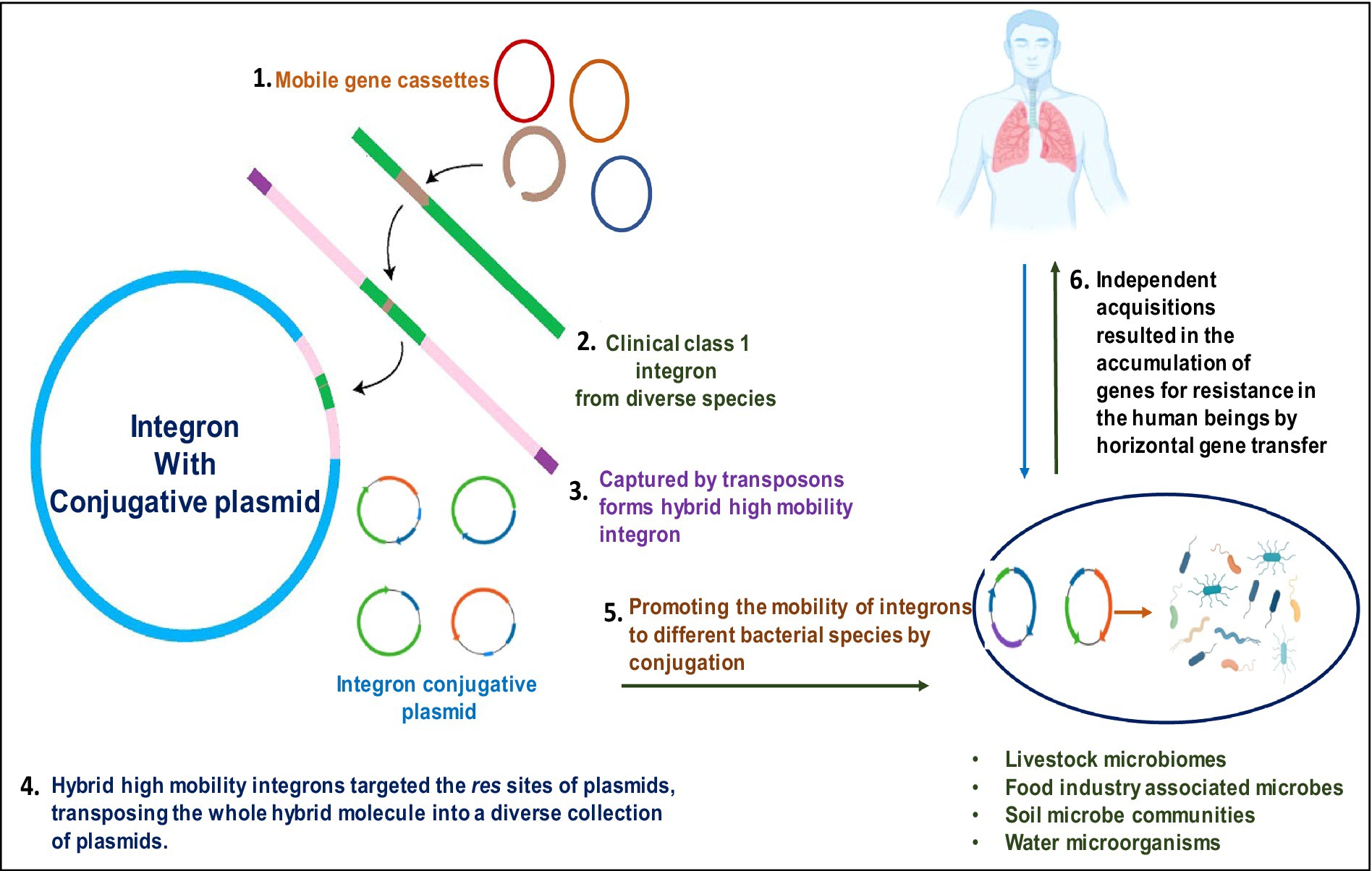
Figure 3. Pictorial representation of integron-aided AMRs to humans: (1) transposons capture integron gene cassettes to yield high mobility integrons that target res sites of plasmids. (2). These plasmids, in turn, promote the mobility of acquired integrons into diverse bacterial species. (3). The acquisitions of resistant genes are transferred to humans through horizontal gene transfer.
Human integron-antibiotic resistance gene cassettes: a rising threat
The resistance to antibiotics by microbe’s accounts for thousands of human deaths annually on a global scale and is continually increasing as a major threat to humans. Predictably, all the sections of researchers initiate the fight against antibiotic resistance, be it clinical settings, communities, or even agricultural systems, targeted to lower the prevention and transmission of antibiotic tolerance from one interface to another. To overcome this issue, several researchers have suggested initiating a “One-Health approach” to have a holistic viewpoint on antibiotic resistance at the level of animals, including humans, and dissemination patterns or routes in the environment (Collignon, 2013; So et al., 2015; Figure 4). Immense variability in genetic makeup, the higher opportunity for mutations, gene rearrangements, and horizontal gene transfer in microbes result in the higher establishment of microbial tolerance. These resistance genes are mobilized by mobile genetic factors, e.g., integrons. They are found in diverse habitats carried by various species of bacteria (Cury et al., 2016; Ghaly et al., 2019; Antelo et al., 2021; Dias et al., 2021). For instance, Dias et al. (2021) employed deep sequencing to explore the integron gene cassettes through studies of intI-attC amplicons. Integrons harboring antimicrobial resistance are critical in disseminating AMR genes through horizontal transfer via transposons and plasmids to develop multi-resistance (Roe et al., 2003; Gaze et al., 2013). The importance of tracing the resistome in colonizing the human gastrointestinal tract and cattle was investigated by the “One Health” approach, Chainier et al. (2017) by employing integrons as instrumental tools to assess the AMR carriage. The “One-Health approach” approach aims to promote the investigation of AMR dissemination through the integration of human health in conjunction with other animals (Chainier et al., 2017). Human feces samples are submitted to a high-throughput sequencing-based metagenomic analysis to study the prevalence and range of AMRs (Zhang et al., 2011; Ma et al., 2014, 2016).
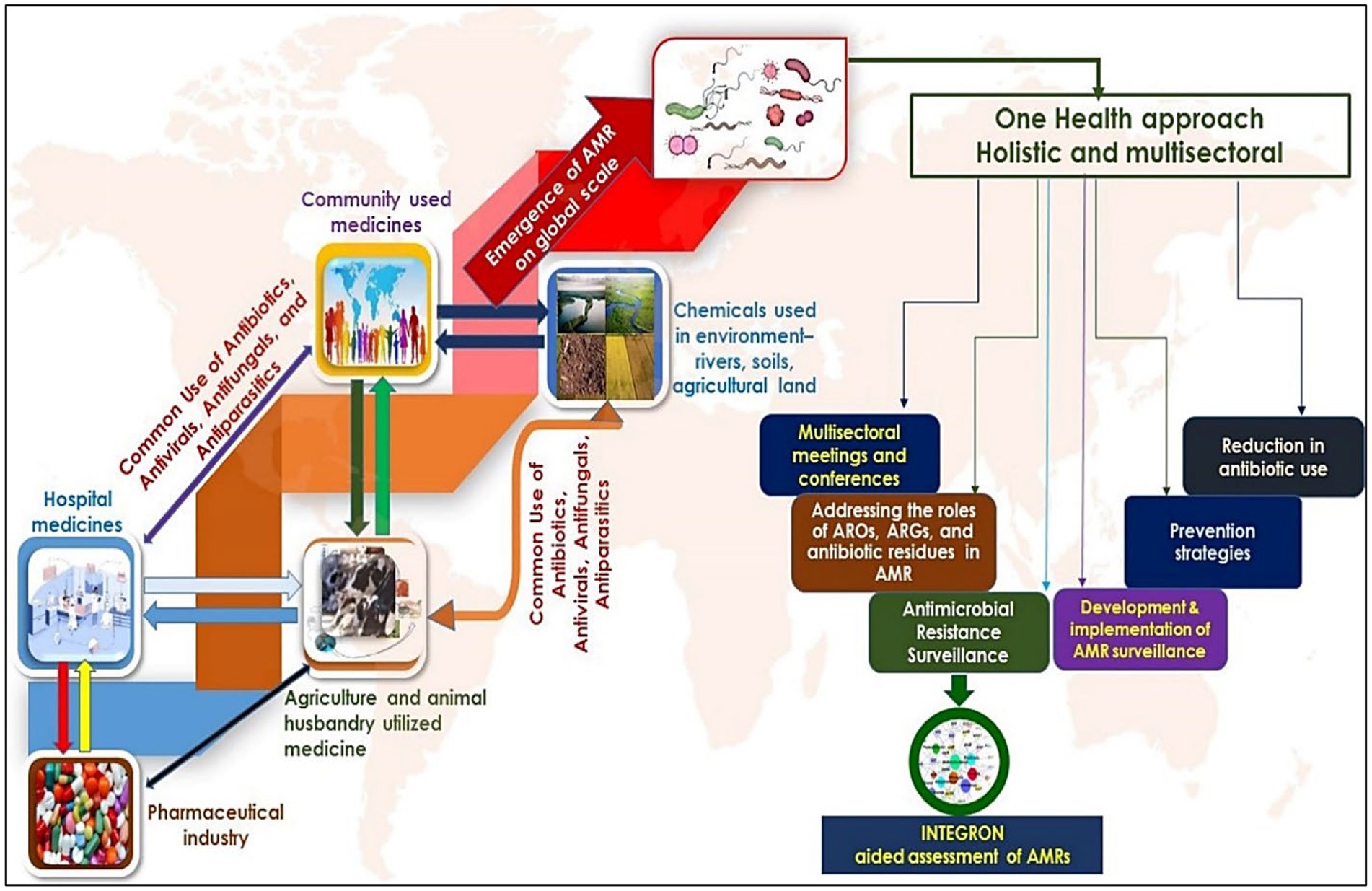
Figure 4. Dissemination and evolution of AMRs- the left side of the figure shows the routes through which antimicrobial resistance is developed and transmitted to different levels. Environmental habitats such as rivers, streams, and agricultural settings play a critical role in acquiring additional AMRs mainly disseminated by humans. The right panel of the figure shows the significance of the ‘One Health Approach’ in addressing the systematic assessment and clinical measures to control the AMRs, most especially by using integrons.
Moreover, culture-dependent and culture-independent approaches are employed in checking the dissemination and surveillance of AMR (Chainier et al., 2017). Since integrons have remarkably evolved as potential markers to identify the AMRs in human digestive carriages, it is reported that integrons coding antibiotic resistance genes are copiously reported in commensal E. coli from human hosts on antibiotics doses (Kheiri and Akhtari, 2016).
Class 1 integrons are found most commonly out of all the different types of integrons. Several studies suggest that integrons are detected in various phyla such as Actinobacteriota, Acidobacteriota, Cyanobacteria, Bacteroidota, Chloroflexota, Firmicutes, Campylobacterota, Chrysiogenetota, Desulfobacterota, Gemmatimonadota, Proteobacteria, Planctomycetota, Spirochaetota, and Verrucomicrobiota (Cury et al., 2016; Parks et al., 2018; Table 2).
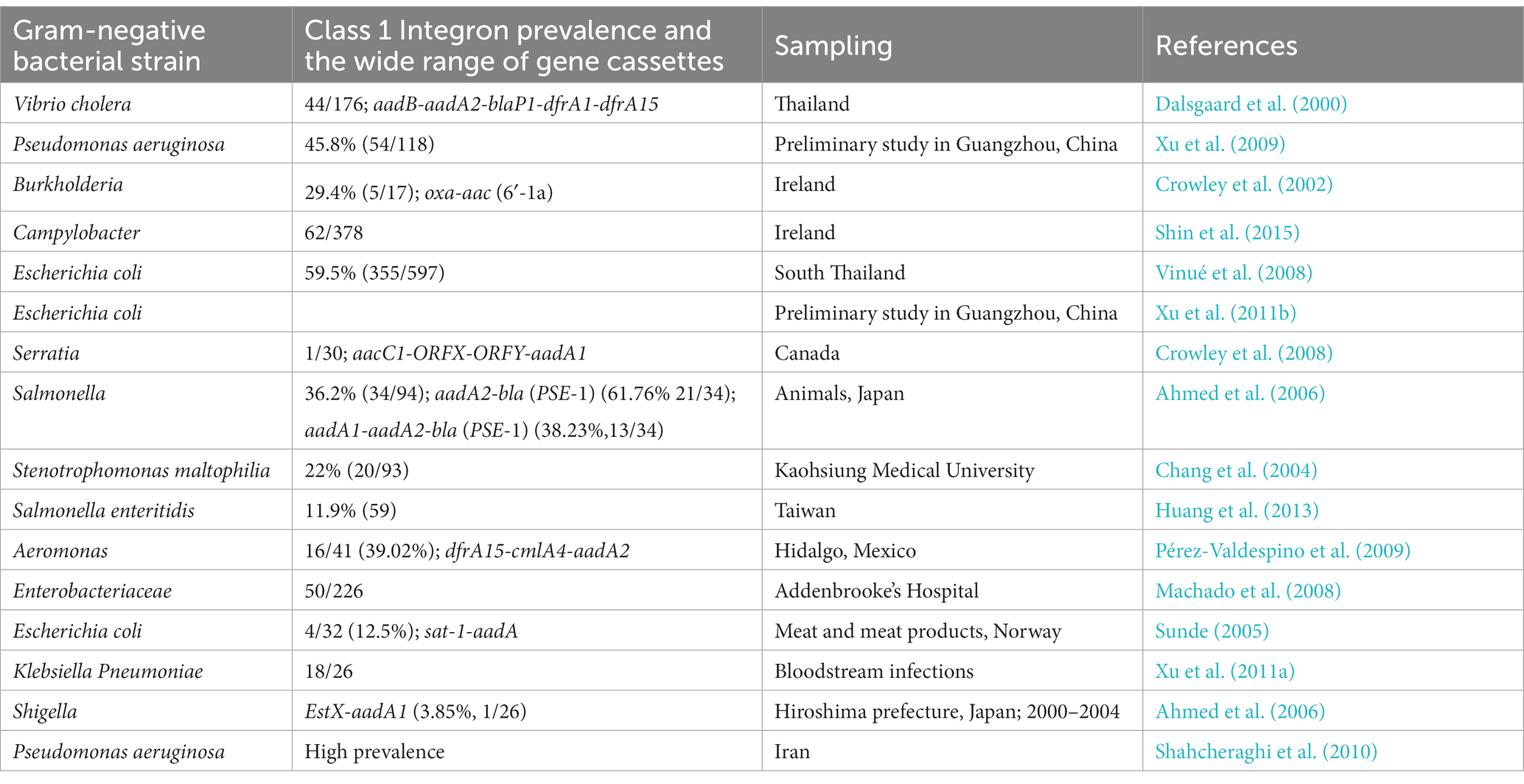
Table 2. Class 1 integron incidence and predominance of Gram-positive and Gram-negative microbial species (Deng et al., 2015).
Class 1 integron is the most abundant and clinically significant. Class 1 integrons are crucial in disseminating AMR genes due to their higher mobility, distribution, and abundance than class 2 and 3 (Table 3; Gillings et al., 2008; Ghaly et al., 2021b). The present research on integrons has been on class 1 integrons, with gram-negative bacteria being the primary importance. The earliest multidrug resistance plasmids identified in the 1950s contained class-1 MIs. Latest research has shown that a significant proportion of isolates of Acinetobacter baumanii, Pseudomonas aeruginosa, E. coli, and Klebsiella pneumoniae have class 1 integrons (Cambray et al., 2010).
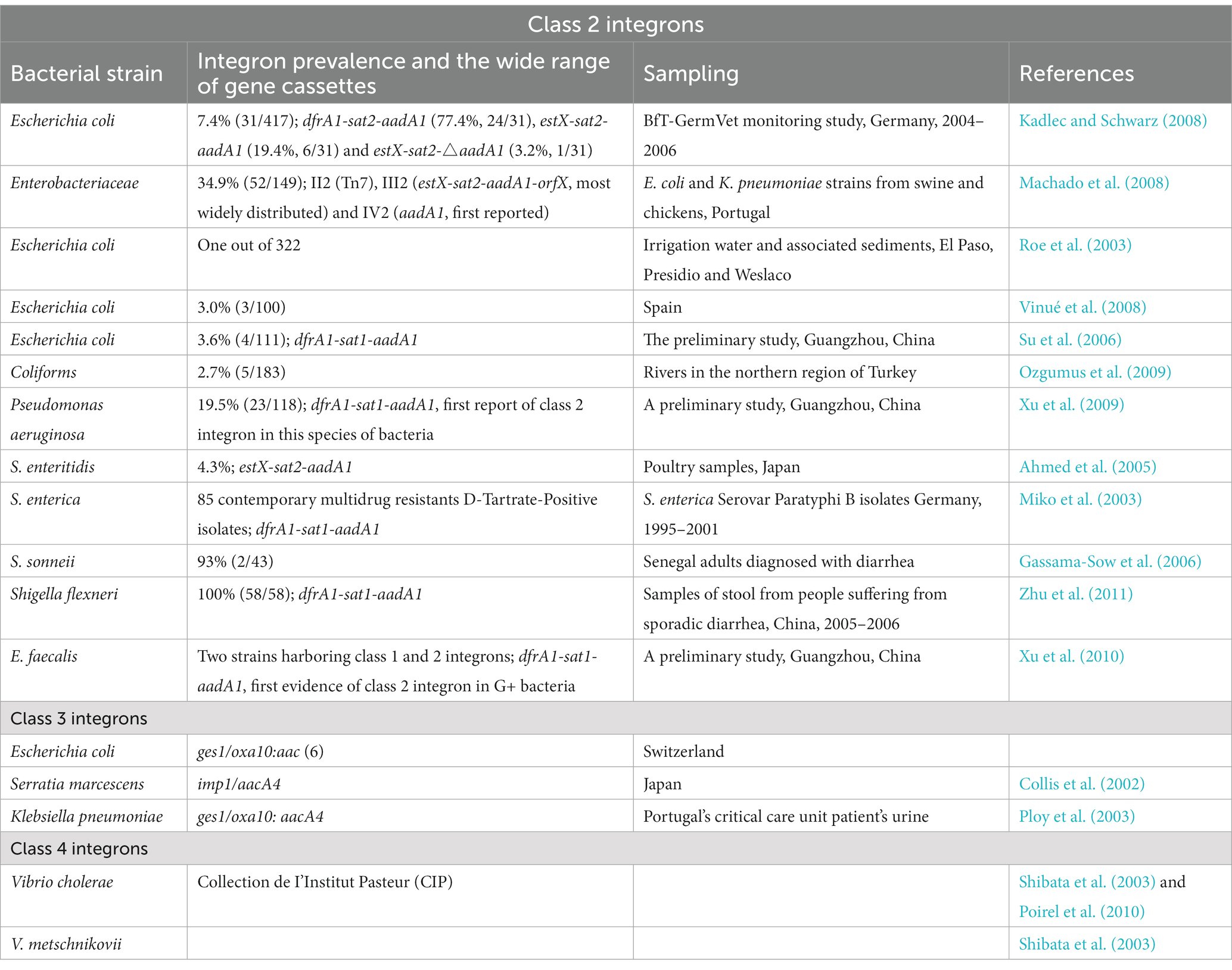
Table 3. Class 2, 3, and 4 integron incidence and predominance in Gram-positive and Gram-negative microbial species (Deng et al., 2015).
The clinical class 1 integron-integrase gene, intI1, is a good indicator of pollution because: (1) it is connected to genes that confer resistance to antibiotics, disinfectants, and heavy metals; (2) it is found in a wide range of pathogenic and nonpathogenic bacteria; (3) its abundance can change quickly because its host cells can have quick generation times; and (4) a single DNA sequence can measure the relative abundance of multiple bacteria (Gillings et al., 2015).
Integrons gene cassettes evolve antimicrobial resistance in primary clinical settings (Zhu et al., 2017; Ghaly et al., 2020b). These cassettes connected to integrons have been gathered from various genomes from different microbial environments. Despite claims to the contrary, it is believed that not all resistance genes found in microbial systems are represented by the resistance cassettes found in integrons and relatively encode resistance genes coding for nucleotidyl transferases, acetyltransferases, and β-lactamases (Partridge et al., 2018). Systematic screening of integron cassettes, which encode novel virulence, antimicrobial-resistance mechanisms, and pathogenicity, unravels the mechanism of invasion and colonization in human hosts (Bengtsson-Palme et al., 2018). For instance, Oliver et al. (2015) stated that integrons are essential in evolving antibiotic tolerance in P. aeruginosa, an opportunistic human pathogenic microbe.
Similarly, Class 4 integron was initially discovered in specific Vibrio cholerae chromosomes as a special form of integron. Additionally, it was a part of γ-proteobacterial genomes (Sabbagh et al., 2021). Class 4 integrons have also been a significant source of antibiotic tolerance and bacterial genome development (Poirel et al., 2018). Class 1 integrons were discovered in 1989 and predominate, especially in clinical contexts (Bush and Bradford, 2020; Ghaly et al., 2021a). Complete integrons are not self-moving components in and of themselves.
Furthermore, resistant integrons share many characteristics, including their ability to move, short cassette arrangements, and usually carrying only antibiotic-tolerance genes. On the other hand, these shared characteristics have not inherited traits of integron ancestors but rather the outcome of strong selection pressure during human antibiotic usage. Given the growing issue of antibiotic resistance, understand how bacteria evolve antibiotic resistance. The bulk of gene cassettes containing genes for antibiotic tolerance is acquired, reorganized, and expressed using mobile integrons (MIs), standard components (Qadri et al., 2021; Souque et al., 2021; Sheikh et al., 2021c). These elements, commonly coupled with transposons and conjugative plasmids, have aided pathogenic bacteria to evolve resistance.
Genetic platforms aid the molecular mechanism regarding the integron-mediated shuffling of antibiotic-tolerance genes. For instance, it has been demonstrated that constitutive amplification of the integrase enzyme speeds up the emergence of chloramphenicol tolerance by causing the deletion of cassettes between Pc and the resistance cassette and the development of co-integrates among copies of the integron (Barraud and Ploy, 2015). Molecular studies revealed that carbapenem resistance in P. aeruginosa is known to be a significant threat globally by the world health organization (WHO) and is set as an ‘extremely significant for scientists to develop novel antibiotic agents (San Millan et al., 2015). Specific reports suggest that integrons such as IntI1 catalysis of cassette insertion depend on specific factors (Vit et al., 2021). For instance, in Vibrio cholerae, the endogenous IntI was inactive in other bacterial hosts, proving the role of host factors needed for integron integrases (Vit et al., 2021).
Integrons as genetic markers to provide an estimation of antimicrobial resistance dissemination
AMR is a serious health problem for humans and animals, and it is spread over various environmental environments. It is believed to be a continuing phenomenon that will pose severe threats to all dimensions of life, including animals, humans, and other organisms found environment. It is emerging as the prime focus of an investigation by scientific communities around the globe. The key player in antibiotic tolerance/resistance genes is integrons, which are promoter-less genetic factors carried from one pathogen to another (Cambray et al., 2010). To initiate a broad-spectrum approach, there is an urgent need to evolve methods to detect antibiotic resistance timely. Forefront to tackle these issues, a conjoint approach such as the “One Health approach” relies on integrated surveillance based on analysis of food, veterinary disease, public health, and the environment (Sikkema and Koopmans, 2016).
Regarding recent studies, integrons are potentially employed as molecular markers to deliver an exact, reliable estimation of AMR dissemination across diverse habitats. Integrons are pivotal potential biomarkers currently employed to identify microbes harboring AMR against a range of antibiotics/drugs (Figure 5). For instance, analysis of integron-associated gene cassettes by targeted sequencing validated the role of integrons to identify the diversity of AMRs from complex microbial communities (Ghaly et al., 2020b). Reports suggest that integrons strongly provide a reliable and complete assessment of AMR expansion (Amos et al., 2015; Gillings et al., 2015). As mentioned in the introduction, integrons are of three main classes, viz., grossly involved in AMR spread. In particular, they are strongly linked to gram-negative bacteria (GNB) multidrug tolerance/resistance (Leverstein-van Hall et al., 2003; Barraud et al., 2014).
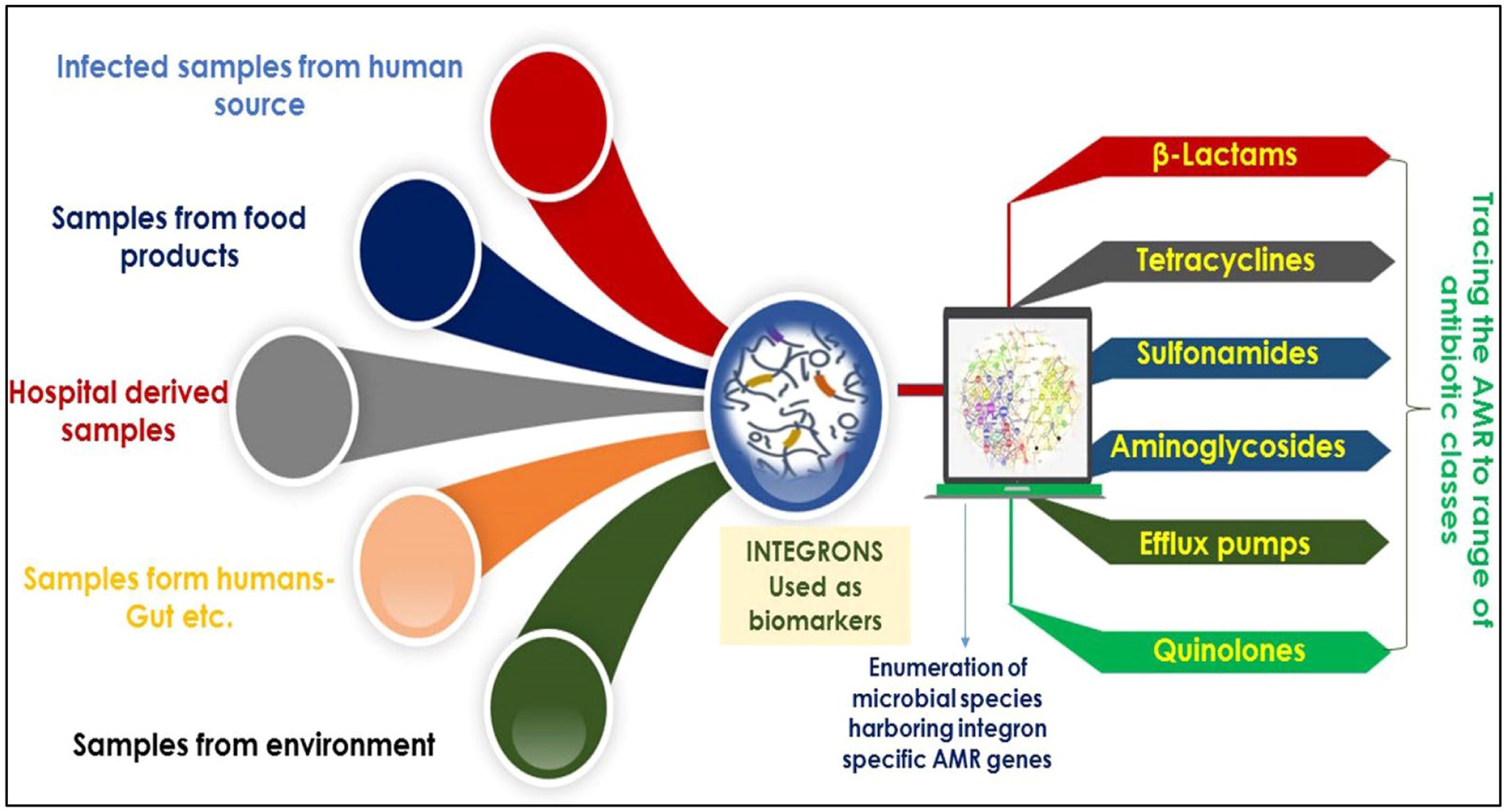
Figure 5. An overview showing the pivotal potential of integrons as biomarkers for the identification of AMR against a range of antibiotics/drugs.
The class 1 integron is majorly found in humans and animals, whereas class 3 is found in samples collected from diverse environments (Tchuinte et al., 2016). Class 1 integrons mediate lateral transfer of DNA in-between pathogenic and commensal bacteria and thus accumulate antibiotic resistance genes in humans relying on the consumption of antibiotics. For example, in Betaproteobacteria based, class 1 integrons intersect the human food chains and can move between different locations among other bacterial species (Gillings et al., 2008). Moreover, Chainier et al. (2017) assessed the carriage of GNB in human and cattle samples by using the “One Health” approach based on cultivation-independent and cultivation-dependent approaches to check for the existence of integrons. They found class 1 integrons as expected, followed by class 2, with class 3 being more common in cattle than humans (Chainier et al., 2017). The study by Gianluca Corno et al., which examined a freshwater system of a lake–river–lake continuum, showed that class 1 integrons are a good proxy for anthropogenic pollution. They also suggest that this genetic platform is a significant driver of aminoglycoside resistance genes, including high-risk ARGs, a grave concern for human health (Corno et al., 2023).
Similarly, Barraud et al. recently reported the potential of integrons as predictive biomarkers for identifying antibiotic resistance in acute sepsis conditions (Barraud et al., 2022). In conjunction with previous reports, the study suggests integrons as a successful predictive marker for acquired antibiotic resistance through the direction in GNB-positive blood cultures (Barraud et al., 2014). Azizi et al. (2021) evaluated the identification and classification of integrons (class 1, 2, and 3) in strains of Acinetobacter baumannii, posing a severe problem for public health in Iran. The finding identified class 1 integrons are predictive biomarkers to validate the existence of MDR phenotypes in the clinical situation. Earlier, PCR-based amplification in Southern Taiwan identified class 1 integrons associated with resistance gene cassettes in Pseudomonas aeruginosa (Hsiao et al., 2014). De Paula et al. (2018) identified 40 resistance markers of clinical relevance to humans by subjecting the metagenomic samples to PCR amplification using markers specific to class 1, 2, and 3 integrons. They identified resistance markers against antibiotic agents like, sulfonamide, and quinolones.
Conclusion and future perspectives
The serious global issue of AMR has put immense health-related pressure on humans and forced specialists to devise strategies to cope with resistance mechanisms evolved by microorganisms. Amid the heavy flow of resistance mechanisms from the environment to humans or vice versa, integrons have played a pivotal part in worsening the growth of tolerance through the expression and interchange of tolerance gene cassettes among different species of bacteria. The last decade has revealed the natural face of integrons and their development as the enquiring spectacle of curious clinical distress. These segments of microbial DNA are diverse in their existence and mechanisms and are reported to generate novel microbial genomes that trigger AMR dissemination and complexity. As explained, this immense health importance of integrons prompted us to provide a holistic view of AMRs with special reference to antibiotic resistance, its dissemination, and mobility through integrons. We propose that understanding the structure, functions, mechanism of AMR dissemination, and diversity of microbes identified through integrons will speed up our efforts to overcome AMR globally. As a result, successful mitigation techniques for AMR in humans and the environment are grossly limited due to a poor understanding of integron diversity, its dissemination mechanism, cross-mobility, and complexity. Moreover, we propose the following strategies to combat the microbial resistance mechanism:
• In-depth understanding of molecular structure and functions of integrons by in-silco analysis.
• In-depth investigation of integron evolution mechanism that drives the acquisition of AMR gene cassettes.
• Decrease the routes through which the resistant bacterial species contaminate humans.
• Reduce a load of antibiotics on the microbial pathogens to avoid selection pressure.
• Enumerate the realistic data on antibiotic resistance genes among major environmental habitats such as rivers, agricultural fields, hospitals, communities, etc.
Timely intervention accounting for mobile resistance gene evolution and mobility will prevent the devastating emergence of microbial resistance species threatening humans throughout the globe. We believe rising novel resistance genes in diverse microbial habitats through integrons would further hinder our efforts to devise treatment strategies, such as targeting integrons by antibiotics with adjuvants to inhibit SOS response. Development of new synthetic as well nature-based antimicrobials to combat the AMR (Chakravorty et al., 2020; Terreni et al., 2021; Bhat et al., 2022a,b; Mir et al., 2022a,b; Ashraf et al., 2023). At the end of 2020, there were 43 antibiotics in clinical development, of which, 15 were Phase I, 13 in Phase II, and 13 in Phase III to combat AMR. They include tetracycline derivatives (eravacycline), fourth generation fluoroquinolones (delafloxacin), new combinations between one β-lactam and one β-lactamase inhibitor (meropenem and vaborbactam), siderophore cephalosporins (cefiderocol), new aminoglycosides (plazomicin), and agents in development for treating drug resistant TB (pretomanid) (Terreni et al., 2021). Our review further bolsters the idea that successful therapeutic plans should focus on integrons, merging antibiotics with additives that restrict integrase function by suppressing the SOS response, favoring resistant bacterial species (Hocquet et al., 2012), limiting the selective pressure on the evolution of integrons. Furthermore, If we successfully manage integron and cassette creation, we may be able to create new biochemical routes for antimicrobial resistance employing integrons as a base to identify new enzymes. To effectively treat patients and employ antibiotics, it is helpful to be aware of the existence of integrons and gene cassettes.
Author contributions
MM and BB designed the work. BB and RM wrote the manuscript and designed the figures. MM, BB, RM, HQ, and RD critically revised and edited the manuscript. All authors have read and approved the manuscript.
Funding
The study was financially supported by Science and Engineering Research Board, Department of Science and Technology (SERB-DST) Govt. of India New Delhi; vide Project Grant No: TAR/001213/2018. We highly appreciate SERB-DST for financial assistance.
Acknowledgments
The authors are thankful to the JK Science Technology and Innovation council DST India, Govt. of India.
Conflict of interest
The authors declare that the research was conducted in the absence of any commercial or financial relationships that could be construed as a potential conflict of interest.
Publisher’s note
All claims expressed in this article are solely those of the authors and do not necessarily represent those of their affiliated organizations, or those of the publisher, the editors and the reviewers. Any product that may be evaluated in this article, or claim that may be made by its manufacturer, is not guaranteed or endorsed by the publisher.
Footnotes
References
Ahmed, A. M., Furuta, K., Shimomura, K., Kasama, Y., and Shimamoto, T. (2006). Genetic characterization of multidrug resistance in Shigella spp. from Japan. J. Med. Microbiol. 55, 1685–1691. doi: 10.1099/jmm.0.46725-0
Ahmed, A. M., Nakano, H., and Shimamoto, T. (2005). Molecular characterization of integrons in non-typhoid Salmonella serovars isolated in Japan: description of an unusual class 2 integron. J. Antimicrob. Chemother. 55, 371–374. doi: 10.1093/jac/dkh534
Akrami, F., Shahandashti, E. F., Yahyapour, Y., Sadeghi, M., Khafri, S., Pournajaf, A., et al. (2017). Integron types, gene cassettes and antimicrobial resistance profile of Acinetobacter baumannii isolated from BAL samples in Babol, North of Iran. Microb. Pathog. 109, 35–38. doi: 10.1016/j.micpath.2017.05.005
Amin, M. B., Saha, S. R., Islam, M. R., Haider, S. M. A., Hossain, M. I., Chowdhury, A. S. M. H. K., et al. (2021). High prevalence of plasmid-mediated quinolone resistance (PMQR) among E. coli from aquatic environments in Bangladesh. PLoS One 16:e0261970. doi: 10.1371/journal.pone.0261970
Amos, G. C. A., Gozzard, E., Carter, C. E., Mead, A., Bowes, M. J., Hawkey, P. M., et al. (2015). Validated predictive modelling of the environmental resistome. ISME J. 9, 1467–1476. doi: 10.1038/ismej.2014.237
Antelo, V., Giménez, M., Azziz, G., Valdespino-Castillo, P., Falcón, L. I., Ruberto, L. A. M., et al. (2021). Metagenomic strategies identify diverse integron-integrase and antibiotic resistance genes in the Antarctic environment. MicrobiologyOpen 10:e1219. doi: 10.1002/mbo3.1219
Antunes, P., Machado, J., and Peixe, L. (2006). Characterization of antimicrobial resistance and class 1 and 2 integrons in Salmonella enterica isolates from different sources in Portugal. J. Antimicrob. Chemother. 58, 297–304. doi: 10.1093/jac/dkl242
Ashraf, M. V., Pant, S., Khan, M. A. H., Shah, A. A., Siddiqui, S., Jeridi, M., et al. (2023). Phytochemicals as antimicrobials: prospecting Himalayan medicinal plants as source of alternate medicine to combat antimicrobial resistance. Pharmaceuticals 16:881. doi: 10.3390/ph16060881
Azizi, O., Fereshteh, S., Nasiri, O., Ghorbani, M., Barzi, S. M., and Badmasti, F. (2021). The occurrence and characterization of class i, ii, and iii integrons among carbapenemase-producing clinical strains of Acinetobacter baumannii in Tehran, Iran. Jundishapur J. Microbiol. 14, 1–13. doi: 10.5812/jjm.117766
Baharoglu, Z., Bikard, D., and Mazel, D. (2010). Conjugative DNA transfer induces the bacterial SOS response and promotes antibiotic resistance development through integron activation. PLoS Genet. 6:e1001165. doi: 10.1371/journal.pgen.1001165
Barraud, O., François, B., Chainier, D., Vignaud, J., and Ploy, M.-C. (2014). Value of integron detection for predicting antibiotic resistance in patients with gram-negative septicaemia. Int. J. Antimicrob. Agents 44, 351–353. doi: 10.1016/j.ijantimicag.2014.06.008
Barraud, O., Guichard, E., Chainier, D., Postil, D., Chimot, L., Mercier, E., et al. (2022). Integrons, a predictive biomarker for antibiotic resistance in acute sepsis: the IRIS study. J. Antimicrob. Chemother. 77, 213–217. doi: 10.1093/jac/dkab348
Barraud, O., and Ploy, M.-C. (2015). Diversity of class 1 integron gene cassette rearrangements selected under antibiotic pressure. J. Bacteriol. 197, 2171–2178. doi: 10.1128/JB.02455-14
Bengtsson-Palme, J., Kristiansson, E., and Larsson, D. G. J. (2018). Environmental factors influencing the development and spread of antibiotic resistance. FEMS Microbiol. Rev. 42:fux053. doi: 10.1093/femsre/fux053
Bennett, P. M. (2008). Plasmid encoded antibiotic resistance: acquisition and transfer of antibiotic resistance genes in bacteria. Br. J. Pharmacol. 153, S347–S357. doi: 10.1038/sj.bjp.0707607
Berendonk, T. U., Manaia, C. M., Merlin, C., Fatta-Kassinos, D., Cytryn, E., Walsh, F., et al. (2015). Tackling antibiotic resistance: the environmental framework. Nat. Rev. Microbiol. 13, 310–317. doi: 10.1038/nrmicro3439
Bhat, B. A., Mir, W. R., Sheikh, B. A., Alkanani, M., and Mir, M. A. (2022a). Metabolite fingerprinting of phytoconstituents from Fritillaria cirrhosa D. Don and molecular docking analysis of bioactive peonidin with microbial drug target proteins. Sci. Rep. 12:7296. doi: 10.1038/s41598-022-10796-7
Bhat, B. A., Mir, W. R., Sheikh, B. A., Rather, M. A., and Mir, M. A. (2022b). In vitro and in silico evaluation of antimicrobial properties of Delphinium cashmerianum L., a medicinal herb growing in Kashmir, India. J. Ethnopharmacol. 291:115046. doi: 10.1016/j.jep.2022.115046
Boucher, Y., Labbate, M., Koenig, J. E., and Stokes, H. W. (2007). Integrons: mobilizable platforms that promote genetic diversity in bacteria. Trends Microbiol. 15, 301–309. doi: 10.1016/j.tim.2007.05.004
Bouvier, M., Demarre, G., and Mazel, D. (2005). Integron cassette insertion: a recombination process involving a folded single strand substrate. EMBO J. 24, 4356–4367. doi: 10.1038/sj.emboj.7600898
Bush, K., and Bradford, P. A. (2020). Epidemiology of β-lactamase-producing pathogens. Clin. Microbiol. Rev. 33, e00047–e00019. doi: 10.1128/CMR.00047-19
Cambray, G., Guerout, A.-M., and Mazel, D. (2010). Integrons. Annu. Rev. Genet. 44, 141–166. doi: 10.1146/annurev-genet-102209-163504
Carattoli, A. (2001). Importance of integrons in the diffusion of resistance. Vet. Res. 32, 243–259. doi: 10.1051/vetres:2001122
Chainier, D., Barraud, O., Masson, G., Couve-Deacon, E., François, B., Couquet, C.-Y., et al. (2017). Integron digestive carriage in human and cattle: a “one Health” cultivation-independent approach. Front. Microbiol. 8:1891. doi: 10.3389/fmicb.2017.01891
Chakravorty, A., Rather, G. A., Ali, A., Bhat, B. A., Sana, S. S., Abhishek, N., et al. (2020). “Nano approach: Indian spices as antimicrobial agents” in Ethnopharmacological investigation of Indian spices (Pennsylvania, United States: IGI Global), 205–241.
Chang, L.-L., Chen, H.-F., Chang, C.-Y., Lee, T.-M., and Wu, W.-J. (2004). Contribution of integrons, and SmeABC and SmeDEF efflux pumps to multidrug resistance in clinical isolates of Stenotrophomonas maltophilia. J. Antimicrob. Chemother. 53, 518–521. doi: 10.1093/jac/dkh094
Collignon, P. (2012). The importance of a one Health approach to preventing the development and spread of antibiotic resistance. Curr. Top. Microbiol. Immunol. 366, 19–36. doi: 10.1007/82_2012_224
Collignon, P. (2013). Ban routine use of critically important antibiotics in food animals. BMJ 347:f4976. doi: 10.1136/bmj.f4976
Collignon, P. J., and Mcewen, S. A. (2019). One health–its importance in helping to better control antimicrobial resistance. Trop. Med. Infect. Dis. 4:22. doi: 10.3390/tropicalmed4010022
Collis, C. M., Kim, M.-J., Partridge, S. R., Stokes, H. W., and Hall, R. M. (2002). Characterization of the class 3 integron and the site-specific recombination system it determines. J. Bacteriol. 184, 3017–3026. doi: 10.1128/JB.184.11.3017-3026.2002
Corno, G., Ghaly, T., Sabatino, R., Eckert, E. M., Galafassi, S., Gillings, M. R., et al. (2023). Class 1 integron and related antimicrobial resistance gene dynamics along a complex freshwater system affected by different anthropogenic pressures. Environ. Pollut. 316:120601. doi: 10.1016/j.envpol.2022.120601
Crowley, D., Cryan, B., and Lucey, B. (2008). First detection of a class 2 integron among clinical isolates of Serratia marcescens. Br. J. Biomed. Sci. 65, 86–89. doi: 10.1080/09674845.2008.11732803
Crowley, D., Daly, M., Lucey, B., Shine, P., Collins, J. J., Cryan, B., et al. (2002). Molecular epidemiology of cystic fibrosis-linked Burkholderia cepacia complex isolates from three national referral centres in Ireland. J. Appl. Microbiol. 92, 992–1004. doi: 10.1046/j.1365-2672.2002.01612.x
Curtiss Iii, R. (1969). Bacterial conjugation. Annu. Rev. Microbiol. 23, 69–136. doi: 10.1146/annurev.mi.23.100169.000441
Cury, J., Jové, T., Touchon, M., Néron, B., and Rocha, E. P. C. (2016). Identification and analysis of integrons and cassette arrays in bacterial genomes. Nucleic Acids Res. 44, 4539–4550. doi: 10.1093/nar/gkw319
Dalsgaard, A., Forslund, A., Serichantalergs, O., and Sandvang, D. (2000). Distribution and content of class 1 integrons in different Vibrio cholerae O-serotype strains isolated in Thailand. Antimicrob. Agents Chemother. 44, 1315–1321. doi: 10.1128/AAC.44.5.1315-1321.2000
De Paula, A. C. L., Medeiros, J. D., De Azevedo, A. C., De Assis Chagas, J. M., Da Silva, V. L., and Diniz, C. G. (2018). Antibiotic resistance genetic markers and integrons in white soft cheese: aspects of clinical resistome and potentiality of horizontal gene transfer. Genes 9:106. doi: 10.3390/genes9020106
Deng, Y., Bao, X., Ji, L., Chen, L., Liu, J., Miao, J., et al. (2015). Resistance integrons: class 1, 2 and 3 integrons. Ann. Clin. Microbiol. Antimicrob. 14:45. doi: 10.1186/s12941-015-0100-6
Dias, M. F., De Castro, G. M., De Paiva, M. C., De Paula Reis, M., Facchin, S., Do Carmo, A. O., et al. (2021). Exploring antibiotic resistance in environmental integron-cassettes through intI-attC amplicons deep sequencing. Braz. J. Microbiol. 52, 363–372. doi: 10.1007/s42770-020-00409-8
Ding, J., Zhu, D., Hong, B., Wang, H. T., Li, G., Ma, Y. B., et al. (2019). Long-term application of organic fertilization causes the accumulation of antibiotic resistome in earthworm gut microbiota. Environ. Int. 124, 145–152. doi: 10.1016/j.envint.2019.01.017
Domingues, S., Da Silva, G. J., and Nielsen, K. M. (2012). Integrons: vehicles and pathways for horizontal dissemination in bacteria. Mob. Genet. Elem. 2, 211–223. doi: 10.4161/mge.22967
Domingues, S., Da Silva, G. J., and Nielsen, K. M. (2015). Global dissemination patterns of common gene cassette arrays in class 1 integrons. Microbiology 161, 1313–1337. doi: 10.1099/mic.0.000099
Escudero, J. A., Loot, C., Nivina, A., and Mazel, D. (2015). The integron: adaptation on demand. Microbiol. Spectr. 3, 3–2. doi: 10.1128/microbiolspec.MDNA3-0019-2014
Fletcher, S. (2015). Understanding the contribution of environmental factors in the spread of antimicrobial resistance. Environ. Health Prev. Med. 20, 243–252. doi: 10.1007/s12199-015-0468-0
Francia, M. V., Zabala, J. C., De La Cruz, F., and García Lobo, J. M. (1999). The IntI1 integron integrase preferentially binds single-stranded DNA of the attC site. J. Bacteriol. 181, 6844–6849. doi: 10.1128/JB.181.21.6844-6849.1999
Gassama-Sow, A., Diallo, M. H., Boye, C. S., Garin, B., Sire, J. M., Sow, A. I., et al. (2006). Class 2 integron-associated antibiotic resistance in Shigella sonnei isolates in Dakar, Senegal. Int. J. Antimicrob. Agents 27, 267–270. doi: 10.1016/j.ijantimicag.2005.10.016
Gaze, W. H., Krone, S. M., Larsson, D. G. J., Li, X.-Z., Robinson, J. A., Simonet, P., et al. (2013). Influence of humans on evolution and mobilization of environmental antibiotic resistome. Emerg. Infect. Dis. 19:e120871. doi: 10.3201/eid1907.120871
Ghaly, T. M., Geoghegan, J. L., Alroy, J., and Gillings, M. R. (2019). High diversity and rapid spatial turnover of integron gene cassettes in soil. Environ. Microbiol. 21, 1567–1574. doi: 10.1111/1462-2920.14551
Ghaly, T. M., Geoghegan, J. L., Tetu, S. G., and Gillings, M. R. (2020a). The peril and promise of integrons: beyond antibiotic resistance. Trends Microbiol. 28, 455–464. doi: 10.1016/j.tim.2019.12.002
Ghaly, T. M., Paulsen, I. T., Sajjad, A., Tetu, S. G., and Gillings, M. R. (2020b). A novel family of Acinetobacter mega-plasmids are disseminating multi-drug resistance across the globe while acquiring location-specific accessory genes. Front. Microbiol. 11:605952. doi: 10.3389/fmicb.2020.605952
Ghaly, T. M., Penesyan, A., Pritchard, A., Qi, Q., Rajabal, V., Tetu, S. G., et al. (2021a). Methods for the targeted sequencing and analysis of integrons and their gene cassettes from complex microbial communities. Microb. Genom. 8:788. doi: 10.1099/mgen.0.000788
Ghaly, T. M., Tetu, S. G., and Gillings, M. R. (2021b). Predicting the taxonomic and environmental sources of integron gene cassettes using structural and sequence homology of attC sites. Commun. Biol. 4, 1–8. doi: 10.1038/s42003-021-02489-0
Gillings, M. R. (2014). Integrons: past, present, and future. Microbiol. Mol. Biol. Rev. 78, 257–277. doi: 10.1128/MMBR.00056-13
Gillings, M., Boucher, Y., Labbate, M., Holmes, A., Krishnan, S., Holley, M., et al. (2008). The evolution of class 1 integrons and the rise of antibiotic resistance. J. Bacteriol. 190, 5095–5100. doi: 10.1128/JB.00152-08
Gillings, M. R., Gaze, W. H., Pruden, A., Smalla, K., Tiedje, J. M., and Zhu, Y.-G. (2015). Using the class 1 integron-integrase gene as a proxy for anthropogenic pollution. ISME J. 9, 1269–1279. doi: 10.1038/ismej.2014.226
Grainge, I., and Jayaram, M. (1999). The integrase family of recombinases: organization and function of the active site. Mol. Microbiol. 33, 449–456. doi: 10.1046/j.1365-2958.1999.01493.x
Guerin, É., Cambray, G., Sanchez-Alberola, N., Campoy, S., Erill, I., Da Re, S., et al. (2009). The SOS response controls integron recombination. Science 324:1034. doi: 10.1126/science.1172914
Hall, R. M., and Collis, C. M. (1995). Mobile gene cassettes and integrons: capture and spread of genes by site-specific recombination. Mol. Microbiol. 15, 593–600. doi: 10.1111/j.1365-2958.1995.tb02368.x
Hall, R. M., Collis, C. M., Kim, M. J., Partridge, S. R., Recchia, G. D., and Stokes, H. W. (1999). Mobile gene cassettes and integrons in evolution. Ann. N. Y. Acad. Sci. 870, 68–80. doi: 10.1111/j.1749-6632.1999.tb08866.x
Hamdani, S. S., Bhat, B. A., Tariq, L., Yaseen, S. I., Ara, I., Rafi, B., et al. (2020). Antibiotic resistance: the future disaster. Int. J. Res. Appl. Sci. Biotechnol. 7:2888 doi: 10.31033/ijrasb.7.4.16
Hocquet, D., Llanes, C., Thouverez, M., Kulasekara, H. D., Bertrand, X., Plésiat, P., et al. (2012). Evidence for induction of integron-based antibiotic resistance by the SOS response in a clinical setting. PLoS Pathog. 8:e1002778. doi: 10.1371/journal.ppat.1002778
Holmes, A. J., Holley, M. P., Mahon, A., Nield, B., Gillings, M., and Stokes, H. W. (2003). Recombination activity of a distinctive integron-gene cassette system associated with Pseudomonas stutzeri populations in soil. J. Bacteriol. 185, 918–928. doi: 10.1128/JB.185.3.918-928.2003
Hsiao, K.-Y., Lee, M.-F., and Peng, C.-F. (2014). Detection and characterization of class 1 integron-associated gene cassettes from Pseudomonas aeruginosa isolates in southern Taiwan. Biomarkers Genom. Med. 6, 74–78. doi: 10.1016/j.bgm.2014.02.004
Huang, S.-C., Chiu, C.-H., Chiou, C.-S., and Yang, Y.-J. (2013). Multidrug-resistant Salmonella enterica serovar Panama carrying class 1 integrons is invasive in Taiwanese children. J. Formos. Med. Assoc. 112, 269–275. doi: 10.1016/j.jfma.2012.02.011
Huddleston, J. R. (2014). Horizontal gene transfer in the human gastrointestinal tract: potential spread of antibiotic resistance genes. Infect. Drug Resist. 7:167. doi: 10.2147/IDR.S48820
Il’ina, T. S. (2003). The mechanisms of horizontal gene transfer: The role of bacteriophages and integrons in the evolution of pathogenic bacteria. Mol. Gen. Mikrobiol. Virusol. 4, 3–10.
Johansson, C. (2007). Mechanisms and DNA specificity in site-specific recombination of integron cassettes. Carolina: Uppsala University
Johnston, C., Martin, B., Fichant, G., Polard, P., and Claverys, J.-P. (2014). Bacterial transformation: distribution, shared mechanisms and divergent control. Nat. Rev. Microbiol. 12, 181–196. doi: 10.1038/nrmicro3199
Juan, C., Zamorano, L., Mena, A., Albertí, S., Pérez, J. L., and Oliver, A. (2010). Metallo-β-lactamase-producing Pseudomonas putida as a reservoir of multidrug resistance elements that can be transferred to successful Pseudomonas aeruginosa clones. J. Antimicrob. Chemother. 65, 474–478. doi: 10.1093/jac/dkp491
Kadlec, K., and Schwarz, S. (2008). Analysis and distribution of class 1 and class 2 integrons and associated gene cassettes among Escherichia coli isolates from swine, horses, cats and dogs collected in the BfT-GermVet monitoring study. J. Antimicrob. Chemother. 62, 469–473. doi: 10.1093/jac/dkn233
Kargar, M., Mohammadalipour, Z., Doosti, A., Lorzadeh, S., and Japoni-Nejad, A. (2014). High prevalence of class 1 to 3 integrons among multidrug-resistant diarrheagenic Escherichia coli in southwest of Iran. Osong. Public Health Res. Perspect. 5, 193–198. doi: 10.1016/j.phrp.2014.06.003
Kheiri, R., and Akhtari, L. (2016). Antimicrobial resistance and integron gene cassette arrays in commensal Escherichia coli from human and animal sources in IRI. Gut Pathog. 8, 1–10. doi: 10.1186/s13099-016-0123-3
Labbate, M., Case, R. J., and Stokes, H. W. (2009). The integron/gene cassette system: an active player in bacterial adaptation. Methods Mol. Biol. 532, 103–125. doi: 10.1007/978-1-60327-853-9_6
Larsson, D. G. J., and Flach, C.-F. (2022). Antibiotic resistance in the environment. Nat. Rev. Microbiol. 20, 257–269. doi: 10.1038/s41579-021-00649-x
Leverstein-Van Hall, M. A., Blok, H. E. M., Donders, A. R. T., Paauw, A., Fluit, A. C., and Verhoef, J. (2003). Multidrug resistance among Enterobacteriaceae is strongly associated with the presence of integrons and is independent of species or isolate origin. J. Infect. Dis. 187, 251–259. doi: 10.1086/345880
Liebert, C. A., Hall, R. M., and Summers, A. O. (1999). Transposon Tn 21, flagship of the floating genome. Microbiol. Mol. Biol. Rev. 63, 507–522. doi: 10.1128/MMBR.63.3.507-522.1999
Liu, C.-C., Tang, C. Y., Chang, K.-C., Kuo, H.-Y., and Liou, M.-L. (2014). A comparative study of class 1 integrons in Acinetobacter baumannii. Gene 544, 75–82. doi: 10.1016/j.gene.2014.04.047
Ma, L., Li, A.-D., Yin, X.-L., and Zhang, T. (2017). The prevalence of integrons as the carrier of antibiotic resistance genes in natural and man-made environments. Environ. Sci. Technol. 51, 5721–5728. doi: 10.1021/acs.est.6b05887
Ma, L., Li, B., and Zhang, T. (2014). Abundant rifampin resistance genes and significant correlations of antibiotic resistance genes and plasmids in various environments revealed by metagenomic analysis. Appl. Microbiol. Biotechnol. 98, 5195–5204. doi: 10.1007/s00253-014-5511-3
Ma, L., Xia, Y., Li, B., Yang, Y., Li, L.-G., Tiedje, J. M., et al. (2016). Metagenomic assembly reveals hosts of antibiotic resistance genes and the shared resistome in pig, chicken, and human feces. Environ. Sci. Technol. 50, 420–427. doi: 10.1021/acs.est.5b03522
Machado, E., Coque, T. M., Canton, R., Sousa, J. C., and Peixe, L. (2008). Antibiotic resistance integrons and extended-spectrum β-lactamases among Enterobacteriaceae isolates recovered from chickens and swine in Portugal. J. Antimicrob. Chemother. 62, 296–302. doi: 10.1093/jac/dkn179
Marathe, N. P., Nagarkar, S. S., Vaishampayan, A. A., Rasane, M. H., Samant, S. A., Dohe, V., et al. (2015). High prevalence of class 1 integrons in clinical isolates of methicillin-resistant Staphylococcus aureus from India. Indian J. Med. Microbiol. 33, 231–236. doi: 10.4103/0255-0857.154905
Marchiaro, P., Viale, A. M., Ballerini, V., Rossignol, G., Vila, A. J., and Limansky, A. (2010). First report of a Tn402-like class 1 integron carrying blaVIM-2 in Pseudomonas putida from Argentina. J. Infect. Dev. Countr. 4, 412–416. doi: 10.3855/jidc.1012
Mazel, D. (2006). Integrons: agents of bacterial evolution. Nat. Rev. Microbiol. 4, 608–620. doi: 10.1038/nrmicro1462
Michael, C. A., Gillings, M. R., Holmes, A. J., Hughes, L., Andrew, N. R., Holley, M. P., et al. (2004). Mobile gene cassettes: a fundamental resource for bacterial evolution. Am. Nat. 164, 1–12. doi: 10.1086/421733
Miko, A., Pries, K., Schroeter, A., and Helmuth, R. (2003). Multiple-drug resistance in D-tartrate-positive Salmonella enterica serovar Paratyphi B isolates from poultry is mediated by class 2 integrons inserted into the bacterial chromosome. Antimicrob. Agents Chemother. 47, 3640–3643. doi: 10.1128/AAC.47.11.3640-3643.2003
Mir, W. R., Bhat, B. A., Almilaibary, A., Asdaq, S. M. B., and Mir, M. A. (2022a). Evaluation of the in vitro antimicrobial activities of Delphinium roylei: an insight from molecular docking and MD-simulation studies. Med. Chem. 18, 1109–1121. doi: 10.2174/1573406418666220429093956
Mir, W. R., Bhat, B. A., Rather, M. A., Muzamil, S., Almilaibary, A., Alkhanani, M., et al. (2022b). Molecular docking analysis and evaluation of the antimicrobial properties of the constituents of Geranium wallichianum D. Don ex Sweet from Kashmir Himalaya. Sci. Rep. 12:12547. doi: 10.1038/s41598-022-16102-9
Mir, M. A., Bhat, B. A., Sheikh, B. A., Rather, G. A., Mehraj, S., and Mir, W. R. (2021). “Nanomedicine in human Health therapeutics and drug delivery: Nanobiotechnology and Nanobiomedicine” in Applications of nanomaterials in agriculture, food science, and medicine (Pennsylvania, United States: IGI Global), 229–251.
Moura, A., Soares, M., Pereira, C., Leitão, N., Henriques, I., and Correia, A. (2009). INTEGRALL: a database and search engine for integrons, integrases and gene cassettes. Bioinformatics 25, 1096–1098. doi: 10.1093/bioinformatics/btp105
Munita, J. M., and Arias, C. A. (2016). Mechanisms of antibiotic resistance. Microbiol. Spectr. 4:16. doi: 10.1128/microbiolspec.VMBF-0016-2015
Naas, T., Mikami, Y., Imai, T., Poirel, L., and Nordmann, P. (2001). Characterization of In53, a class 1 plasmid-and composite transposon-located integron of Escherichia coli which carries an unusual array of gene cassettes. J. Bacteriol. 183, 235–249. doi: 10.1128/JB.183.1.235-249.2001
Nakamura, Y., Itoh, T., Matsuda, H., and Gojobori, T. (2004). Biased biological functions of horizontally transferred genes in prokaryotic genomes. Nat. Genet. 36, 760–766. doi: 10.1038/ng1381
Nield, B. S., Holmes, A. J., Gillings, M. R., Recchia, G. D., Mabbutt, B. C., Nevalainen, K. M. H., et al. (2001). Recovery of new integron classes from environmental DNA. FEMS Microbiol. Lett. 195, 59–65. doi: 10.1111/j.1574-6968.2001.tb10498.x
Nwosu, V. C. (2001). Antibiotic resistance with particular reference to soil microorganisms. Res. Microbiol. 152, 421–430. doi: 10.1016/S0923-2508(01)01215-3
Ochman, H., Lawrence, J. G., and Groisman, E. A. (2000). Lateral gene transfer and the nature of bacterial innovation. Nature 405, 299–304. doi: 10.1038/35012500
Oliver, A., Mulet, X., López-Causapé, C., and Juan, C. (2015). The increasing threat of Pseudomonas aeruginosa high-risk clones. Drug Resist. Updat. 21, 41–59. doi: 10.1016/j.drup.2015.08.002
Ozeki, H., and Ikeda, H. (1968). Transduction mechanisms. Annu. Rev. Genet. 2, 245–278. doi: 10.1146/annurev.ge.02.120168.001333
Ozgumus, O. B., Sandalli, C., Sevim, A., Celik-Sevim, E., and Sivri, N. (2009). Class 1 and class 2 integrons and plasmid-mediated antibiotic resistance in coliforms isolated from ten rivers in northern Turkey. J. Microbiol. 47, 19–27. doi: 10.1007/s12275-008-0206-z
Parks, D. H., Chuvochina, M., Waite, D. W., Rinke, C., Skarshewski, A., Chaumeil, P.-A., et al. (2018). A standardized bacterial taxonomy based on genome phylogeny substantially revises the tree of life. Nat. Biotechnol. 36, 996–1004. doi: 10.1038/nbt.4229
Partridge, S. R., Kwong, S. M., Firth, N., and Jensen, S. O. (2018). Mobile genetic elements associated with antimicrobial resistance. Clin. Microbiol. Rev. 31, e00088–e00017. doi: 10.1128/CMR.00088-17
Partridge, S. R., Tsafnat, G., Coiera, E., and Iredell, J. R. (2009). Gene cassettes and cassette arrays in mobile resistance integrons. FEMS Microbiol. Rev. 33, 757–784. doi: 10.1111/j.1574-6976.2009.00175.x
Pérez-Valdespino, A., Fernández-Rendón, E., and Curiel-Quesada, E. (2009). Detection and characterization of class 1 integrons in Aeromonas spp. isolated from human diarrheic stool in Mexico. J. Basic Microbiol. 49, 572–578. doi: 10.1002/jobm.200900095
Ploy, M.-C., Chainier, D., Tran Thi, N. H., Poilane, I., Cruaud, P., Denis, F., et al. (2003). Integron-associated antibiotic resistance in Salmonella enterica serovar Typhi from Asia. Antimicrob. Agents Chemother. 47, 1427–1429. doi: 10.1128/AAC.47.4.1427-1429.2003
Poirel, L., Carattoli, A., Bernabeu, S., Bruderer, T., Frei, R., and Nordmann, P. (2010). A novel IncQ plasmid type harbouring a class 3 integron from Escherichia coli. J. Antimicrob. Chemother. 65, 1594–1598. doi: 10.1093/jac/dkq166
Poirel, L., Madec, J.-Y., Lupo, A., Schink, A.-K., Kieffer, N., Nordmann, P., et al. (2018). Antimicrobial resistance in Escherichia coli. Microbiol. Spectr. 6:26. doi: 10.1128/microbiolspec.ARBA-0026-2017
Qadri, H., Shah, A. H., and Mir, M. (2021). Novel strategies to combat the emerging drug resistance in human pathogenic microbes. Curr. Drug Targets 22, 1424–1436. doi: 10.2174/1389450121666201228123212
Racewicz, P., Majewski, M., Madeja, Z. E., Łukomska, A., and Kubiak, M. (2020). Role of integrons in the proliferation of multiple drug resistance in selected bacteria occurring in poultry production. Br. Poult. Sci. 61, 122–131. doi: 10.1080/00071668.2019.1697426
Razavi, M., Marathe, N. P., Gillings, M. R., Flach, C.-F., Kristiansson, E., and Joakim Larsson, D. G. (2017). Discovery of the fourth mobile sulfonamide resistance gene. Microbiome 5:160. doi: 10.1186/s40168-017-0379-y
Rendueles, O., De Sousa, J. A. M., Bernheim, A., Touchon, M., and Rocha, E. P. C. (2018). Genetic exchanges are more frequent in bacteria encoding capsules. PLoS Genet. 14:e1007862. doi: 10.1371/journal.pgen.1007862
Roe, M. T., Vega, E., and Pillai, S. D. (2003). Antimicrobial resistance markers of class 1 and class 2 integron-bearing Escherichia coli from irrigation water and sediments. Emerg. Infect. Dis. 9:822. doi: 10.3201/eid0907.020529
Sabbagh, P., Ebrahimzadeh-Namvar, A., Ferdosi-Shahandashti, E., Javanian, M., Khafri, S., and Rajabnia, M. (2017). Molecular characterization of Staphylococcus aureus strains isolated among hospital staff nasal carriers of Babol, Iran. Caspian J. Intern. Med. 8:311. doi: 10.22088/cjim.8.4.311
Sabbagh, P., Rajabnia, M., Maali, A., and Ferdosi-Shahandashti, E. (2021). Integron and its role in antimicrobial resistance: A literature review on some bacterial pathogens. Iran. J. Basic Med. Sci. 24:136. doi: 10.22038/ijbms.2020.48905.11208
San Millan, A., Toll-Riera, M., Escudero, J. A., Cantón, R., Coque, T. M., and Maclean, R. C. (2015). Sequencing of plasmids pAMBL1 and pAMBL2 from Pseudomonas aeruginosa reveals a Bla VIM-1 amplification causing high-level carbapenem resistance. J. Antimicrob. Chemother. 70, 3000–3003. doi: 10.1093/jac/dkv222
Sandoval-Quintana, E., Lauga, B., and Cagnon, C. (2022). Environmental integrons: the dark side of the integron world. Trends Microbiol. 31, 432–434. doi: 10.1016/j.tim.2022.01.009
Serwecińska, L. (2020). Antimicrobials and antibiotic-resistant bacteria: a risk to the environment and to public health. Water 12:3313. doi: 10.3390/w12123313
Shahcheraghi, F., Badmasti, F., and Feizabadi, M. M. (2010). Molecular characterization of class 1 integrons in MDR Pseudomonas aeruginosa isolated from clinical settings in Iran, Tehran. FEMS Immunol. Med. Microbiol. 58, 421–425. doi: 10.1111/j.1574-695X.2009.00636.x
Sheikh, B. A., Bhat, B. A., Ahmad, Z., and Mir, M. A. (2021a). Strategies employed to evade the host immune response and the mechanism of drug resistance in Mycobacterium tuberculosis: in search of finding new targets. Curr. Pharm. Biotechnol. doi: 10.2174/1389201023666211222164938
Sheikh, B. A., Bhat, B. A., Alshehri, B., Mir, R. A., Mir, W. R., Parry, Z. A., et al. (2021b). Nano-drug delivery systems: possible end to the rising threats of tuberculosis. J. Biomed. Nanotechnol. 17, 2298–2318. doi: 10.1166/jbn.2021.3201
Sheikh, B. A., Bhat, B. A., Mehraj, U., Mir, W., Hamadani, S., and Mir, M. A. (2021c). Development of new therapeutics to meet the current challenge of drug resistant tuberculosis. Curr. Pharm. Biotechnol. 22, 480–500. doi: 10.2174/1389201021666200628021702
Sheikh, B. A., Bhat, B. A., and Mir, M. A. (2022). Antimicrobial resistance: new insights and therapeutic implications. Appl. Microbiol. Biotechnol. 106, 6427–6440. doi: 10.1007/s00253-022-12175-8
Shibata, N., Doi, Y., Yamane, K., Yagi, T., Kurokawa, H., Shibayama, K., et al. (2003). PCR typing of genetic determinants for metallo-β-lactamases and integrases carried by gram-negative bacteria isolated in Japan, with focus on the class 3 integron. J. Clin. Microbiol. 41, 5407–5413. doi: 10.1128/JCM.41.12.5407-5413.2003
Shin, E., Hong, H., Oh, Y., and Lee, Y. (2015). First report and molecular characterization of a Campylobacter jejuni isolate with extensive drug resistance from a travel-associated human case. Antimicrob. Agents Chemother. 59, 6670–6672. doi: 10.1128/AAC.01395-15
Sikkema, R., and Koopmans, M. (2016). One Health training and research activities in Western Europe. Infect. Ecol. Epidemiol. 6:33703. doi: 10.3402/iee.v6.33703
So, A. D., Shah, T. A., Roach, S., Chee, Y. L., and Nachman, K. E. (2015). An integrated systems approach is needed to ensure the sustainability of antibiotic effectiveness for both humans and animals. J. Law Med. Ethics 43, 38–45. doi: 10.1111/jlme.12273
Souque, C., Escudero, J. A., and Maclean, R. C. (2021). Integron activity accelerates the evolution of antibiotic resistance. elife 10:e62474. doi: 10.7554/eLife.62474
Sow, A. G., Aïdara-Kane, A., Barraud, O., Gatet, M., Denis, F., and Ploy, M.-C. (2010). High prevalence of trimethoprim-resistance cassettes in class 1 and 2 integrons in Senegalese Shigella spp isolates. J. Infect. Dev. Ctries. 4, 207–212. doi: 10.3855/jidc.583
Stokes, H. W., and Gillings, M. R. (2011). Gene flow, mobile genetic elements and the recruitment of antibiotic resistance genes into gram-negative pathogens. FEMS Microbiol. Rev. 35, 790–819. doi: 10.1111/j.1574-6976.2011.00273.x
Stokes, H. W. T., and Hall, R. M. (1989). A novel family of potentially mobile DNA elements encoding site-specific gene-integration functions: integrons. Mol. Microbiol. 3, 1669–1683. doi: 10.1111/j.1365-2958.1989.tb00153.x
Stokes, H. W., Nesbø, C. L., Holley, M., Bahl, M. I., Gillings, M. R., and Boucher, Y. (2006). Class 1 integrons potentially predating the association with Tn 402-like transposition genes are present in a sediment microbial community. J. Bacteriol. 188, 5722–5730. doi: 10.1128/JB.01950-05
Su, J., Shi, L., Yang, L., Xiao, Z., Li, X., and Yamasaki, S. (2006). Analysis of integrons in clinical isolates of Escherichia coli in China during the last six years. FEMS Microbiol. Lett. 254, 75–80. doi: 10.1111/j.1574-6968.2005.00025.x
Sunde, M. (2005). Prevalence and characterization of class 1 and class 2 integrons in Escherichia coli isolated from meat and meat products of Norwegian origin. J. Antimicrob. Chemother. 56, 1019–1024. doi: 10.1093/jac/dki377
Sundström, L. (1998). The potential of integrons and connected programmed rearrangements for mediating horizontal gene transfer. APMIS Suppl. 84, 37–42. doi: 10.1111/j.1600-0463.1998.tb05646.x
Tchuinte, P. L. S., Stalder, T., Venditti, S., Ngandjio, A., Dagot, C., Ploy, M.-C., et al. (2016). Characterisation of class 3 integrons with oxacillinase gene cassettes in hospital sewage and sludge samples from France and Luxembourg. Int. J. Antimicrob. Agents 48, 431–434. doi: 10.1016/j.ijantimicag.2016.06.018
Terreni, M., Taccani, M., and Pregnolato, M. (2021). New antibiotics for multidrug-resistant bacterial strains: latest research developments and future perspectives. Molecules 26:2671. doi: 10.3390/molecules26092671
Thomas, C. M., and Nielsen, K. M. (2005). Mechanisms of, and barriers to, horizontal gene transfer between bacteria. Nat. Rev. Microbiol. 3, 711–721. doi: 10.1038/nrmicro1234
Toleman, M. A., and Walsh, T. R. (2011). Combinatorial events of insertion sequences and ICE in gram-negative bacteria. FEMS Microbiol. Rev. 35, 912–935. doi: 10.1111/j.1574-6976.2011.00294.x
Torren-Edo, J., Grave, K., and Mackay, D. (2015). One Health: the regulation and consumption of antimicrobials for animal use in the EU. IHAJ 2, 14–16.
Venema, G. (1979). Bacterial transformation. Adv. Microb. Physiol. 19, 245–331. doi: 10.1016/s0065-2911(08)60200-3
Verraes, C., Van Boxstael, S., Van Meervenne, E., Van Coillie, E., Butaye, P., Catry, B., et al. (2013). Antimicrobial resistance in the food chain: a review. Int. J. Environ. Res. Public Health 10, 2643–2669. doi: 10.3390/ijerph10072643
Vinué, L., Saenz, Y., Somalo, S., Escudero, E., Moreno, M. A., Ruiz-Larrea, F., et al. (2008). Prevalence and diversity of integrons and associated resistance genes in faecal Escherichia coli isolates of healthy humans in Spain. J. Antimicrob. Chemother. 62, 934–937. doi: 10.1093/jac/dkn331
Vit, C., Richard, E., Fournes, F., Whiteway, C., Eyer, X., Lapaillerie, D., et al. (2021). Cassette recruitment in the chromosomal Integron of Vibrio cholerae. Nucleic Acids Res. 49, 5654–5670. doi: 10.1093/nar/gkab412
Wellington, E. M. H., Boxall, A. B. A., Cross, P., Feil, E. J., Gaze, W. H., Hawkey, P. M., et al. (2013). The role of the natural environment in the emergence of antibiotic resistance in gram-negative bacteria. Lancet Infect. Dis. 13, 155–165. doi: 10.1016/S1473-3099(12)70317-1
World Health Organization. (2015). Global action plan on antimicrobial resistance. Geneva: World Health Organization
Wu, Y.-W., Doak, T. G., and Ye, Y. (2013). The gain and loss of chromosomal integron systems in the Treponemaspecies. BMC Evol. Biol. 13:16. doi: 10.1186/1471-2148-13-16
Xu, Z., Li, L., Shi, L., and Shirtliff, M. E. (2011a). Class 1 integron in staphylococci. Mol. Biol. Rep. 38, 5261–5279. doi: 10.1007/s11033-011-0676-7
Xu, Z., Li, L., Shirtliff, M. E., Alam, M. J., Yamasaki, S., and Shi, L. (2009). Occurrence and characteristics of class 1 and 2 integrons in Pseudomonas aeruginosa isolates from patients in Southern China. J. Clin. Microbiol. 47, 230–234. doi: 10.1128/JCM.02027-08
Xu, Z., Li, L., Shirtliff, M. E., Peters, B. M., Li, B., Peng, Y., et al. (2011b). Resistance class 1 integron in clinical methicillin-resistant Staphylococcus aureus strains in southern China, 2001–2006. Clin. Microbiol. Infect. 17, 714–718. doi: 10.1111/j.1469-0691.2010.03379.x
Xu, Z., Li, L., Shirtliff, M. E., Peters, B. M., Peng, Y., Alam, M. J., et al. (2010). First report of class 2 integron in clinical enterococcus faecalis and class 1 integron in Enterococcus faecium in South China. Diagn. Microbiol. Infect. Dis. 68, 315–317. doi: 10.1016/j.diagmicrobio.2010.05.014
Xu, Z., Li, L., Zhao, X., Chu, J., Li, B., Shi, L., et al. (2011c). Development and application of a novel multiplex polymerase chain reaction (PCR) assay for rapid detection of various types of staphylococci strains. Afr. J. Microbiol. Res. 5, 1869–1873. doi: 10.5897/AJMR11.437
Yu, G., Li, Y., Liu, X., Zhao, X., and Li, Y. (2013). Role of integrons in antimicrobial resistance: A review. Afr. J. Microbiol. Res. 7, 1301–1310. doi: 10.22038/ijbms.2020.48905.11208
Zhang, X., Wu, B., Zhang, Y., Zhang, T., Yang, L., Fang, H. H. P., et al. (2009). Class 1 integronase gene and tetracycline resistance genes tetA and tetC in different water environments of Jiangsu Province, China. Ecotoxicology 18, 652–660. doi: 10.1007/s10646-009-0332-3
Zhang, T., Zhang, X.-X., and Ye, L. (2011). Plasmid metagenome reveals high levels of antibiotic resistance genes and mobile genetic elements in activated sludge. PLoS One 6:e26041. doi: 10.1371/journal.pone.0026041
Zhu, J. Y., Duan, G. C., Yang, H. Y., Fan, Q. T., and Xi, Y. L. (2011). Atypical class 1 integron coexists with class 1 and class 2 integrons in multi-drug resistant Shigella flexneri isolates from China. Curr. Microbiol. 62, 802–806. doi: 10.1007/s00284-010-9790-3
Zhu, Y.-G., Zhao, Y. I., Li, B., Huang, C.-L., Zhang, S.-Y., Yu, S., et al. (2017). Continental-scale pollution of estuaries with antibiotic resistance genes. Nat. Microbiol. 2:16270. doi: 10.1038/nmicrobiol.2016.270
Keywords: antimicrobial resistance, antibiotic stewardship, horizontal gene transfer, integrons, pathogenicity
Citation: Bhat BA, Mir RA, Qadri H, Dhiman R, Almilaibary A, Alkhanani M and Mir MA (2023) Integrons in the development of antimicrobial resistance: critical review and perspectives. Front. Microbiol. 14:1231938. doi: 10.3389/fmicb.2023.1231938
Edited by:
Saurabh Mishra, Cornell University, United StatesReviewed by:
Deepika Prasad, Memorial Sloan Kettering Cancer Center, United StatesBrendon Lee, Cornell University, United States
Copyright © 2023 Bhat, Mir, Qadri, Dhiman, Almilaibary, Alkhanani and Mir. This is an open-access article distributed under the terms of the Creative Commons Attribution License (CC BY). The use, distribution or reproduction in other forums is permitted, provided the original author(s) and the copyright owner(s) are credited and that the original publication in this journal is cited, in accordance with accepted academic practice. No use, distribution or reproduction is permitted which does not comply with these terms.
*Correspondence: Manzoor Ahmad Mir, drmanzoor@kashmiruniversity.ac.in