- 1Department of Microbial and Plant Biotechnology, Centro de Investigaciones Biológicas Margarita Salas-Consejo Superior de Investigaciones Científicas, Madrid, Spain
- 2Research and Development Center, Saudi Aramco, Dhahran, Saudi Arabia
A synthetic dibenzothiophene (DBT) mineralization pathway has been engineered in recombinant cells of Pseudomonas azelaica Aramco J strain for its use in biodesulfurization of thiophenic compounds and crude oil. This functional pathway consists of a combination of a recombinant 4S pathway responsible for the conversion of DBT into 2-hydroxybiphenyl (2HBP) and a 2HBP mineralization pathway that is naturally present in the parental P. azelaica Aramco J strain. This novel approach allows overcoming one of the major bottlenecks of the biodesulfurization process, i.e., the feedback inhibitory effect of 2HBP on the 4S pathway enzymes. Resting cells-based biodesulfurization assays using DBT as a sulfur source showed that the 2HBP generated from the 4S pathway is subsequently metabolized by the cell, yielding an increase of 100% in DBT removal with respect to previously optimized Pseudomonas putida biodesulfurizing strains. Moreover, the recombinant P. azelaica Aramco J strain was able to use DBT as a carbon source, representing the best characterized biocatalyst harboring a DBT mineralization pathway and constituting a suitable candidate to develop future bioremediation/bioconversion strategies for oil-contaminated sites.
Introduction
Over the last decades, biodesulfurization (BDS) has become an attractive approach that aims to complement the traditional hydrodesulfurization treatment of crude oils and refined products to specifically remove sulfur from S-heterocyclic compounds that are recalcitrant to the chemical treatments (Martínez et al., 2017). Dibenzothiophene (DBT) and its alkylated derivatives are the prototypical compounds targeted in BDS (Monticello, 2000; Soleimani et al., 2007). The most studied pathway to remove sulfur from DBT is the 4S pathway (Figure 1A). The 4S route is a non-destructive aerobic pathway that converts DBT into 2-hydroxybiphenyl (2HBP) through two oxidation steps, catalyzed by the DszC and DszA monooxygenase enzymes, followed by a hydrolytic step, mediated by the DszB desulfinase, which are usually plasmid encoded. The FMNH2 required for the oxidation steps is supplied by a NADH-FMN oxidoreductase (DszD; Figure 1A), which is usually chromosomally encoded (Gupta et al., 2005; Mohebali and Ball, 2016; Kilbane, 2017; Parveen et al., 2020; Hirschler et al., 2021; Martín-Cabello et al., 2022).
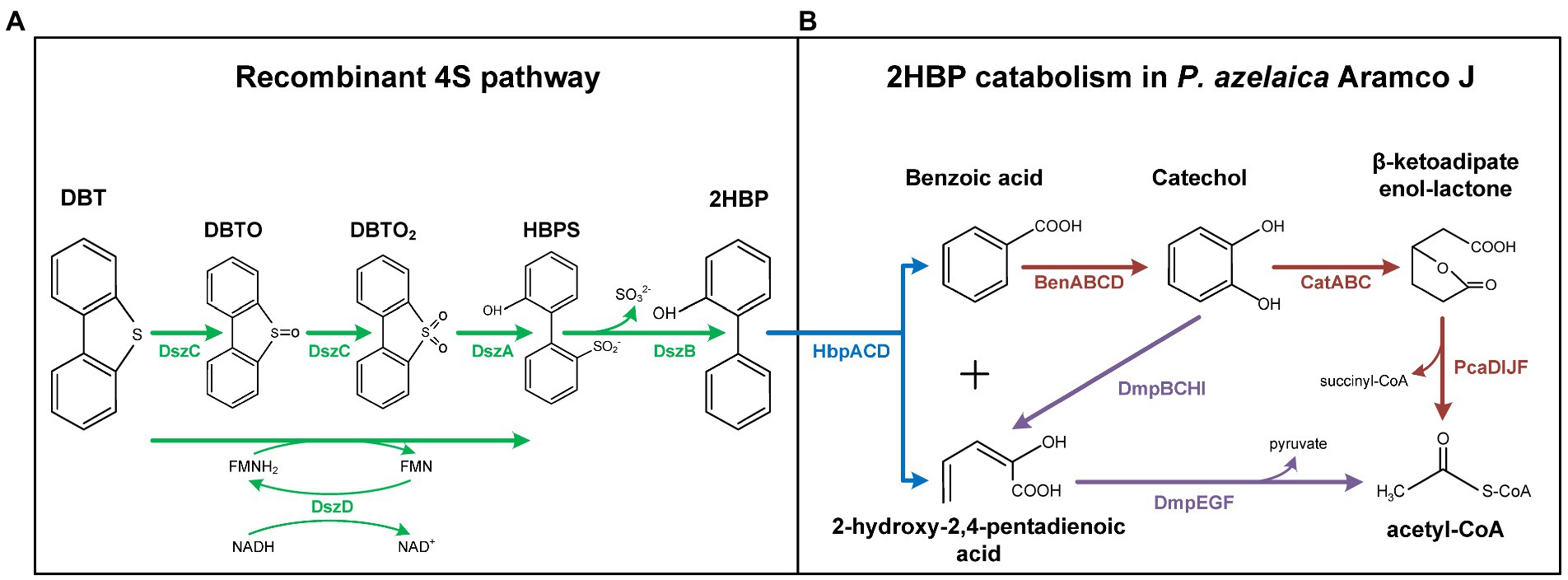
Figure 1. Synthetic DBT degradation pathway in recombinant Pseudomonas azelaica AJdsz strain. The synthetic pathway was constructed by coupling a recombinant 4S pathway (A) with a 2HBP degradation pathway naturally present in P. azelaica Aramco J (B). Green: dsz pathway; blue: hbp pathway; brown: ben-cat-pca pathways; purple: dmp pathway. DBT, dibenzothiophene; DBTO, dibenzothiophene sulfoxide; DBTO2 dibenzothiophene sulfone; HBPS, 2-hydroxybiphenyl-2-sulfinate; 2HBP, 2-hydroxybiphenyl.
One of the major bottlenecks of the biodesulfurization of DBT through the 4S route is the inhibitory effect of the final product, 2HBP, on the initial Dsz enzymes (Xu et al., 2006; Chen et al., 2008; Akhtar et al., 2009; Abin-Fuentes et al., 2013; Kilbane, 2017; Li et al., 2019a). To deal with this limitation, different approaches have been applied over the years. Optimization of the oil/aqueous phase ratio is an option to weaken the feedback inhibition effects (Feng et al., 2016). Compartmentalization of the individual reactions of the 4S pathway into different hosts allows for their individual optimization to overcome 2HBP feedback inhibition (Martínez et al., 2016). The use of 2HBP-reasonably resistant bacteria harboring the 4S pathway can handle the inhibitory effects of 2HBP in cell growth (Akhtar et al., 2018). Directed evolution combined with a high-throughput screening method, combinatorial mutagenesis and enzyme overexpression generated a DszC mutant enzyme that was less sensitive to 2HBP feedback inhibition than the parental enzyme (Li et al., 2019a). Other alternative is the use of an extended 4S pathways where the final product, e.g., 2-methoxybiphenyl, has less inhibitory effects than 2HBP (Li et al., 2003; Chen et al., 2009; Bordoloi et al., 2014, 2016).
In this work, we propose a new approach to overcome the 2HBP-mediated inhibition of biodesulfurization through the expression of the 4S pathway in a 2HBP-degrading bacterium, Pseudomonas azelaica strain Aramco J (Mohamed et al., 2015), that is highly similar (99% nucleotide identity) to the well-studied Pseudomonas nitroreducens strain HBP-1 that can naturally export and catabolize 2HBP using the tripartite complex pump MexAB-OprM and HbpCAD enzymes, respectively (Jaspers et al., 2001; Czechowska et al., 2013; Carraro et al., 2020). The engineered P. azelaica strain is the first rationally-designed biocatalyst that couples the 4S pathway to the subsequent degradation of 2HBP, yielding a synthetic pathway that significantly increases DBT biodesulfurization yield, and allows the use of DBT as a carbon source.
Materials and methods
Chemicals and culture media
Suppliers of the different chemicals used were as follows: 2HBP was from Fluka; DBT, HEPES, glutamic acid, glucose, Tween 80, gentamicin, kanamycin and isopropyl β-D-thiogalactopyraniside (IPTG) were from Sigma-Aldrich. All the chemicals used to prepare the different media were from Sigma-Aldrich. Deionized water (resistivity 18.5 MΩ cm) was used to prepare all of the media.
Lysogeny broth (LB) medium containing 1% tryptone, 0.5% yeast extract and 1% NaCl was used for the pre-cultures (Miller, 1972). Sulfur-free basal salts medium (BSM) employed for the growth experiments using DBT (0.1 mM) as a sulfur source had the following composition (Martín et al., 2004): NaH2PO4·H2O 4 g/l; K2HPO4·3H2O 4 g/l; NH4Cl 2 g/l; MgCl2·6H2O 0.0245 g/l; CaCl2·2H2O 0.001 g/l; and FeCl3·6H2O 0.001 g/l. M63 medium was used for the growth experiments using DBT (2.5 mM) as a carbon source and had the following composition (Miller, 1972): KH2PO4 13.6 g/l; (NH4)SO4 2 g/l; FeSO4·7H2O 0.5 mg/l, adjusted to pH 7.0 using KOH, with trace solutions (MnCl2·4H2O 1.98 mg/l; CoSO4·7H2O 2.81 mg/l; CaCl2·2H2O 1.47 mg/l; CuCl2·2H2O 0.17 mg/l and ZnSO4·7H2O 0.29 mg/l). Due to the low solubility of DBT, this medium was sonicated for 30 min in a sonication bath to maximize the particle dispersion and obtain a homogeneous distribution without visually aggregated particles of DBT.
Resting cells assays were conducted in 50 mM HEPES buffer at pH 8.0 supplemented with Tween 80 (0.1%) and sonicated for 30 min in a sonication bath after DBT addition.
Bacterial strains, plasmids and growth conditions
Bacterial strains, plasmids and primers used in this work are listed in Table 1. Escherichia coli strains were grown in LB medium at 37°C and 200 rpm. P. azelaica and Pseudomonas putida strains were grown at 30°C in an orbital shaker at 200 rpm in BSM or M63 media. When appropriate, antibiotics were added at the following concentrations: ampicillin (100 μg mL−1), kanamycin (50 μg mL−1), gentamicin (10 μg mL−1).
Growing cells experiments
All experiments started with overnight pre-cultures in LB medium containing the appropriate antibiotics. For the growth experiments using DBT as a sulfur source, P. azelaica pre-cultures were washed twice with saline solution (0.9% NaCl, w/v) and used to inoculate (initial OD600 of 0.1) 50 ml of sulfur-free BSM medium supplemented with glucose (2 g/l), glycerol (2.45 g/l), 0.1 mM DBT and 1 mM IPTG in 250 ml Erlenmeyer flasks.
For the growth experiments using DBT as a carbon source, P. azelaica pre-cultures were washed twice with saline solution and used to inoculate (initial OD600 of 0.1) 50 ml of pre-sonicated M63 medium supplemented with 6.8 mM glutamic acid, Tween 80 (0.1%) and 2.5 mM DBT or 2.5 mM 2HBP in 250 ml Erlenmeyer flasks.
Optical density at 600 nm (OD600) was measured using a Shimadzu UV–vis spectrophotometer (model UV mini 1,240). Due to the high turbidity of the M63 medium when 2.5 mM DBT was added, growth under these conditions was determined as colony forming units (CFUs) by plating culture samples in LB solid medium.
Resting cells assays
For the resting cells assays, P. azelaica and P. putida strains were grown overnight in LB medium containing the appropriate antibiotics. Then, cultures were washed twice with saline solution and used to inoculate 50 ml of M63 medium supplemented with glutamic acid (20 g/l), 1 mM IPTG and the appropriate antibiotic. After 24 h, cultures were washed twice in saline solution and resuspended in HEPES buffer supplemented with Tween 80 (0.1%) and 0.5 mM DBT at an OD600 of 2.0 (about 1 g/l cell dry weight) to start the resting cells assays.
Samples were collected periodically and mixed with an equal volume of acetonitrile, then centrifuged at 14,000 ×g for 10 min and filtered through 0.22 μm polyethersulfone filters (Minisart high flow, Sartorius) for the subsequent HPLC analysis.
Molecular biology techniques
Standard molecular biology techniques were performed as previously described (Sambrook and Russell, 2001). Plasmid DNA was prepared with a High Pure plasmid isolation kit (Roche Applied Science). DNA fragments were purified with Gene-Clean Turbo (Q-BIOgene). Oligonucleotides were supplied by Sigma. All cloned inserts and DNA fragments were confirmed by DNA sequencing through an ABI Prism 377 automated DNA sequencer (Applied Biosystems Inc.). Transformation of bacterial cells was carried out by electroporation (Gene Pulser; BioRad) (Sambrook and Russell, 2001).
Construction of Pseudomonas azelaica AJdhbpA strain
For insertional disruption of the hbpA gene through single homologous recombination, a 1.1 kb internal region of hbpA gene was PCR-amplified using primers hbpA-Fw and hbpA-Rv and cloned into EcoRI site of vector pGEM-T Easy giving rise to plasmid pGEM-hbpA (Table 1). The pGEM-hbpA plasmid was digested with EcoRI, and the 1.1 kb fragment was then subcloned into the pK18mob suicide vector giving rise to the pK18mobhbpA plasmid. The pK18mobhbpA plasmid was purified and transformed by electroporation into P. azelaica AJ. The recombinant strain, harboring the disrupted hbpA gene by insertion of the pK18mobhbpA suicide plasmid, was isolated on kanamycin-containing LB agar plates. The mutant strain was finally analyzed by PCR to confirm the disruption of hbpA gene.
Analytical methods
HPLC (Agilent 1100 Series) was employed to analyze the concentration of DBT and 2HBP using a C18 column (Teknokroma C18 150×4.6 mm, 5 μm particles) at 1 ml/min flow rate. The mobile phase was a mixture acetonitrile/water (55:45). Peaks were monitored at different wave lengths (234 nm for DBT and 206 nm for 2HBP). Calibrations were performed using highly purified standards of each compound.
Results and discussion
Engineering a heterologous 4S biodesulfurization pathway in recombinant Pseudomonas azelaica Aramco J strain
Pseudomonas azelaica strain Aramco J (P. azelaica AJ) was isolated from an oil-contaminated soil sample from Abu Ali Island (Saudi Arabia) and is naturally adapted to degrade 2HBP as well as other hydroxybiphenyls (Mohamed et al., 2015). Growth experiments revealed that P. azelaica AJ was able to grow in the presence of up to 15 g/l 2HBP. Degradation of 2HBP is carried out by the hbp pathway that generates benzoate and 2-hydroxy-pentadienoate (Figure 1B; Jaspers et al., 2001). The hbpACD genes are located within an integrative-conjugative element (ICEhbp) in the genome of P. azelaica AJ (Mohamed et al., 2015). Next to the hbp genes in the ICEhbp element are located the dmp genes encoding a catechol meta-cleavage pathway also involved in the metabolism of 2-hydroxy-pentadienoate (Figure 1B). The degradation of benzoate involves its initial conversion to catechol by the benABCD gene products (Figure 1B). Catechol can be then catabolized through the dmp meta-cleavage pathway or via the cat ortho-cleavage pathway (Figure 1B). Two different ben-cat gene clusters are located outside the ICEhbp element, and they are mapped at different positions of the Aramco J genome (Mohamed et al., 2015). The final product of the cat pathway, i.e., β-ketoadipate enol-lactone, is finally converted to Krebs-cycle intermediates through the β-ketoadipate pathway (Figure 1B) which is encoded by two pca gene clusters, one located within ICEhbp element and the other associated to one of the ben-cat clusters (Mohamed et al., 2015).
In a previous work, we have constructed a synthetic 4S pathway (dszB1A1C1-D1 cassette) that enhanced the bioconversion of DBT to 2HBP when expressed in P. putida KT2440 (Martínez et al., 2016). Plasmid pIZdszB1A1C1-D1 is a pIZ1016-derived plasmid that harbors the synthetic dszB1, dszA1 and dszC1 genes under control of the IPTG-dependent Ptac/lacIq regulatory system, and dszD1 gene under control of Ptac/lacIq regulatory system (Martínez et al., 2016). When plasmid pIZdszB1A1C1-D1 was transferred to P. azelaica AJ, the resulting strain P. azelaica AJ (pIZdszB1A1C1-D1), named P. azelaica AJdsz, was predicted to acquire the ability to transform DBT into 2HBP by the 4S pathway with the subsequent degradation of 2HBP by the endogenous metabolic network of the host strain (Figure 1). To confirm this assumption, we performed desulfurization experiments by growing cells in the presence of DBT.
In contrast to the wild-type P. azelaica AJ strain, the recombinant P. azelaica AJdsz was able to grow using 0.1 mM DBT as the sole sulfur source when IPTG was added to the culture medium (Figure 2A), hence demonstrating that the heterologous 4S pathway was successfully expressed in the recombinant P. azelaica AJdsz strain. Analyzes of the culture supernatants along the growth curve confirmed that all DBT was removed during the exponential growth phase in about 20 h (Figure 2B). Although some 2HBP accumulated in the supernatants during DBT consumption reaching a maximum of 23 μM at 24 h, it was subsequently degraded until its complete removal (Figure 2B). These results further confirm that P. azelaica AJdsz is able to use sulfur from DBT through the 4S pathway without accumulating 2HBP as final product, thus suggesting that 2HBP is efficiently metabolized by the action of the endogenous hbp genes avoiding the 2HBP-dependent feedback inhibition of Dsz enzymes. The initial accumulation of 2HBP in the culture medium is likely due to the fact that the initial biodegradation steps of DBT are expressed in a multicopy plasmid under the control of strong promoters, whereas the degradation pathway of 2HBP is expressed in a single copy in the chromosome. The fine tuning between both pathways to avoid the accumulation of intermediates, a property that is acquired during evolution, can be achieved in the future by changing gene copy number and the promoters. Moreover, it should be mentioned that a search in the genome of strain Aramco J revealed the presence in contig 7 of a mexABoprM cluster highly similar to that reported in P. nitroreducens HBP-1 strain and that encodes an efficient 2HBP efflux system that is crucial to secrete 2HBP and tolerate high 2HBP concentrations (Czechowska et al., 2013). Secretion of 2HBP via the MexAB-OprM efflux pump could also contribute to the action of the HbpCAD enzymes metabolizing 2HBP to prevent high 2HBP intracellular accumulation and thus, helping to reduce feedback inhibition of the Dsz enzymes. Interestingly, neither in strain HBP-1 nor in Aramco J strain the mexABoprM cluster is linked to the catabolic hbpCAD genes but rather it is located elsewhere on the chromosome (Carraro et al., 2020).
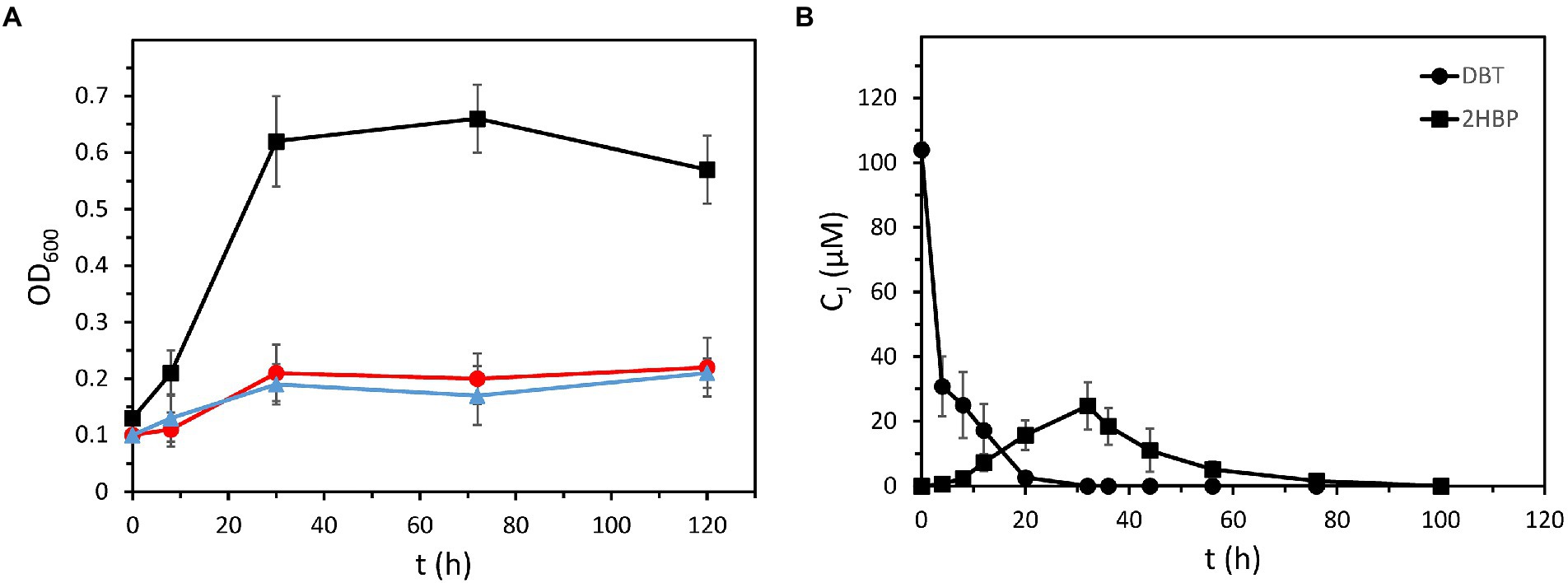
Figure 2. Growth of recombinant P. azelaica AJdsz using DBT as the only sulfur source. (A) Growth of recombinant P. azelaica AJdsz in BSM containing 100 μM of DBT as the only sulfur source, 0.2% (w/v) glucose as carbon source, and 1 mM IPTG to induce the dsz gene expression (black squares). Control experiments of P. azelaica AJdsz without DBT (blue triangles) and wild-type P. azelaica AJ strain with DBT (red circles) are shown. (B) Time course of the evolution of DBT (black circles) and 2HBP (black squares) during growth of recombinant P. azelaica AJdsz in DBT as only sulfur source. Cj indicates the concentration of the monitored compound. Values are the mean of three different experiments. Error bars indicate standard deviations.
Nevertheless, to check whether growth on 2HBP could inhibit the Dsz enzymes in P. azelaica AJdsz, this recombinant strain and the wild-type P. azelaica AJ strain were grown in minimal medium with 2 mM 2HBP as carbon source and 0.1 mM DBT or 0.1 mM sulfate as sole sulfur sources (Table 2). As expected, P. azelaica AJ was able to grow using sulfate but it did not grow in the presence of DBT. In contrast, the strain AJdsz was able to grow under both conditions (Table 2), indicating that the dsz pathway expressed in P. azelaica AJdsz is functional even when growing the cells in the presence of high amounts of 2HBP.
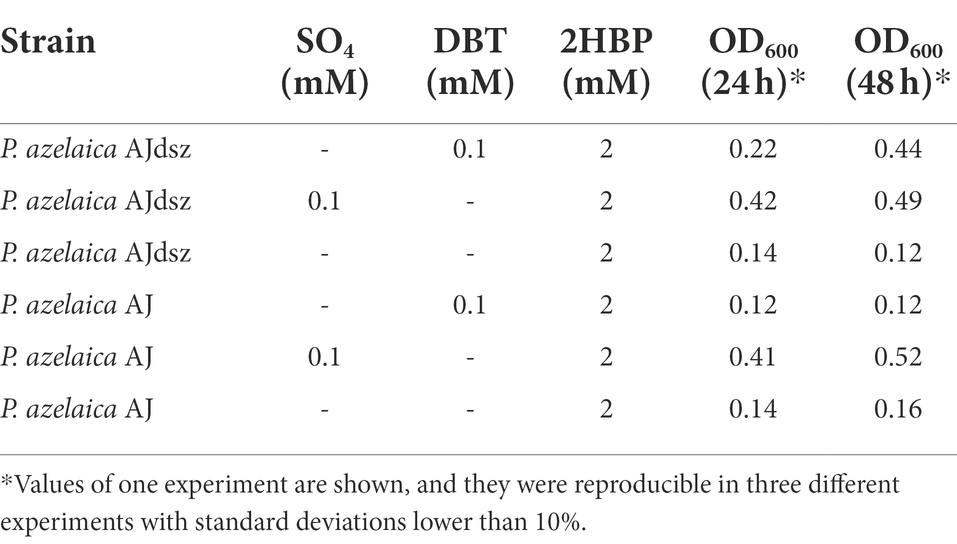
Table 2. Growth of P. azelaica AJ strains in BSM with 2HBP as a carbon source and SO4 or DBT as a sulfur source.
All these results taken together suggest that the efficient metabolism of 2HBP in P. azelaica AJdsz reduces the inhibitory effects of this 4S pathway intermediate, and encourage the use of this recombinant strain as a suitable biocatalyst for DBT desulfurization.
Recombinant Pseudomonas azelaica AJdsz strain as a biocatalyst for DBT desulfurization
As suggested above, the expression of the 4S pathway in a 2HBP degrading bacterium, as P. azelaica AJdsz, reduces the 2HBP-dependent inhibition of Dsz enzymes by efficiently degrading this intermediate. To confirm this hypothesis, the desulfurizing capacity of P. azelaica AJdsz was tested using 500 μM DBT in resting cell assays. P. azelaica AJdsz was able to efficiently remove about 85% of the initial DBT after 120 min (Figure 3A) with almost no net accumulation of 2HBP (Figure 3B). This result contrasts with that obtained under the same conditions with our best desulfurizing strain so far, i.e., P. putida KT2440 (pIZdszB1A1C1-D1; Martínez et al., 2016), that was able to remove only 40% of the initial DBT (Figure 3A) and accumulated an equimolar amount of 2HBP (about 200 μM, Figure 3B). Thus, P. azelaica AJdsz strain behaved as a more efficient DBT biodesulfurizer than the previously engineered P. putida strain, in agreement with the expected lower 2HBP accumulation in the resting assays.
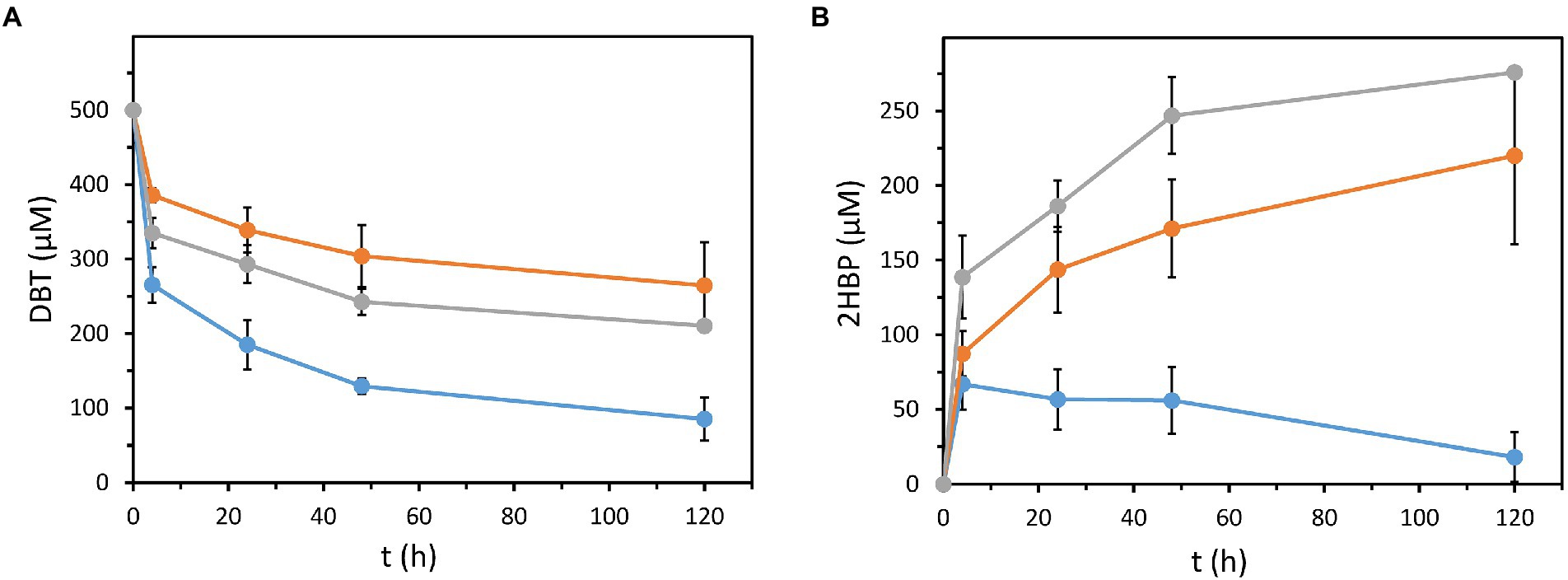
Figure 3. DBT degradation and 2HBP accumulation in resting cells assays. Strains tested were: P. azelaica AJdsz (blue circles), P. azelaica AJdhbpAdsz (gray triangles) and P. putida KT2440 harboring plasmid pIZdszB1A1C1-D1 (orange squares). (A) Evolution of DBT concentration in resting cells assays containing 500 μM of DBT as initial sulfur substrate. (B) Evolution of 2HBP concentration. Values are the mean of three different experiments. Error bars indicate standard deviations.
To confirm that the significant increase of DBT desulfurization in P. azelaica AJdsz strain was due to the metabolism of the released 2HBP, we engineered a mutant P. azelaica AJ strain lacking a functional HbpA enzyme responsible for the first step in the 2HBP catabolism. The mutant strain, P. azelaica AJdhpbA, was transformed with plasmid pIZdszB1A1C1-D1 and, as expected, the resulting strain P. azelaica AJdhpbAdsz was unable to grow using 2HBP as carbon source. The recombinant strain P. azelaica AJdhpbAdsz was able to desulfurize DBT via the 4S pathway, although it was only able to eliminate 50% of the initial DBT (Figure 3A) accumulating equimolar amounts of 2HBP in the culture medium (Figure 3B). This behavior was similar to that of P. putida KT2440 (pIZdszB1A1C1-D1), another strain unable to degrade 2HBP (Martínez et al., 2016), and contrasts with the higher desulfurization efficiency of the P. azelaica AJdsz strain able to metabolize 2HBP (Figure 3). Therefore, all these results taken together revealed that a bacterial host cell able to metabolize 2HBP and expressing the dsz genes, as P. azelaica AJdsz, constitutes a successful DBT biodesulfurizer strain that reaches one of the highest specific desulfurization activities (about 42 μmolDBT/g DCW/h) reported so far when using microbial resting cell cultures in aqueous phase (Martínez et al., 2016; Li et al., 2019a).
Use of DBT as a carbon source by recombinant Pseudomonas azelaica AJdsz strain
Another important property of the recombinant P. azelaica AJdsz strain developed in this work is that this strain has the enzymatic machinery required to use DBT as a carbon source (Figure 1). To test the mineralization of DBT in P. azelaica AJdsz strain, initial tests were carried out in minimal medium with DBT as the sole carbon and energy source, but no apparent growth was observed. Since the 4S pathway requires high amounts of NADH (Galán et al., 2000; Abin-Fuentes et al., 2013), it might be necessary the use of an additional carbon source to provide the energy required for the initial degradation of DBT. Thus, we checked the growth of P. azelaica AJdsz by counting the colony forming units (CFU/ml) in minimal medium with 2.5 mM DBT (30 mM total carbon) in the presence of 6.8 mM glutamic acid (34 mM total carbon) as additional carbon and energy source (Martín et al., 2004). The results obtained revealed that the addition of 2.5 mM DBT increased cell growth with respect to the control experiment without DBT (Figure 4A). Nevertheless, the CFU value was lower than that obtained using 2.5 mM 2HBP (30 mM total carbon) as carbon and energy source (Figure 4A). HPLC analysis of the culture supernatants at 72 h revealed that whereas all 2HBP was consumed, only about 0.7 mM DBT (8.4 mM total carbon) was removed without accumulating 2HBP. This result explains the higher CFU value observed when the cells grew in 2HBP. Interestingly, when we used the P. azelaica AJdhbpAdsz mutant strain unable to catabolize 2HBP we did not observe any increase of cell growth in DBT or 2HBP with respect to the control experiment without such carbon source (Figure 4B). It is possible that DBT degradation may deliver carbon atoms at quite different rate than glutamate and 2HBP due to the relative insolubility of the DBT substrate. Therefore, these results allow us to conclude that 2HBP generated from DBT desulfurization via the 4S pathway is finally mineralized in strain P. azelaica AJdsz, likely by using the catabolic scheme depicted in Figure 1.
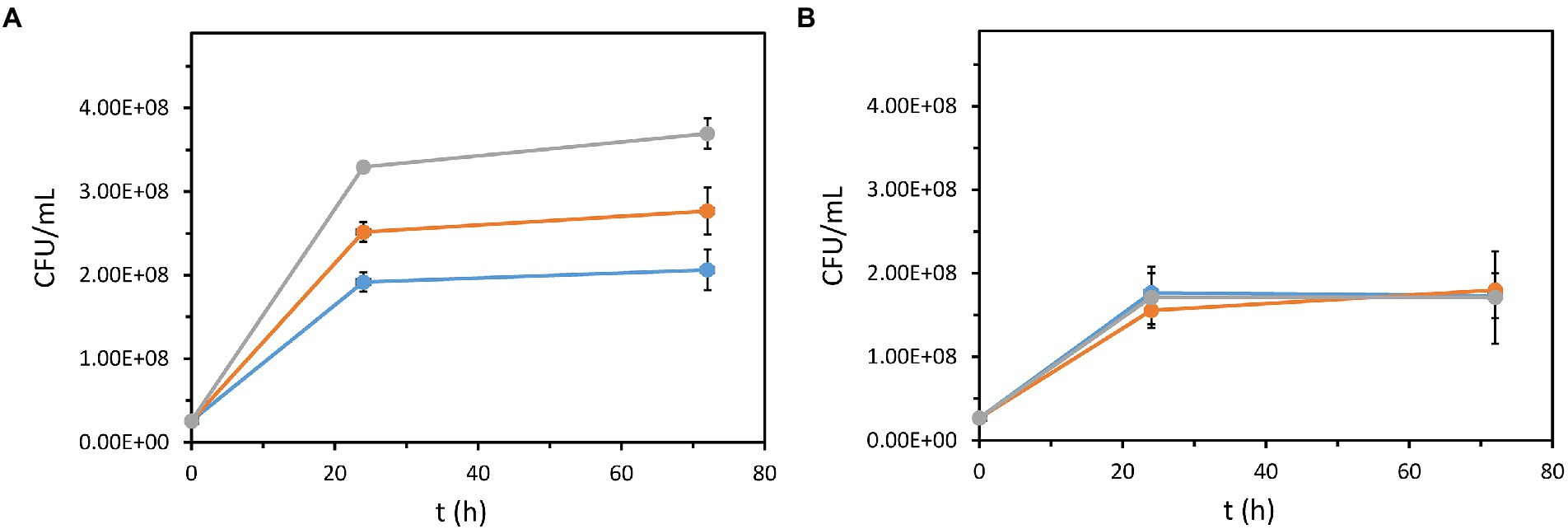
Figure 4. Growth of recombinant P. azelaica strains using DBT or 2HBP as a carbon source. P. azelaica AJdsz (A) and P. azelaica AJdhbpAdsz (B) were grown in M63 medium with 6.8 mM glutamic acid (basal carbon and energy source) in the absence (blue lines) or in the presence of 2.5 mM DBT (orange lines) or 2.5 mM 2HBP (gray lines). Due to the high turbidity of the medium, growth was determined as CFU/mL (1 gDCW/L = 5.2·108 CFU/ml). Values are the mean of three different experiments. Error bars indicate standard deviations.
Polyaromatic sulfur heterocycles can persist over the long term with low bioavailability under field conditions and they are harmful to their surroundings. DBT, in particular, has been confirmed to be carcinogenic, teratogenic, and mutagenic to humans and other species either by ingestion, touch, or inhalation even at trace concentrations (Kirimura et al., 2001; Xu et al., 2006; Li et al., 2012). Surprisingly, despite DBT has been extensively studied as a sulfur source in desulfurization processes, there are only a few works dealing with the use of DBT as a carbon source in bacteria, and there is a lack of knowledge on the metabolic pathways responsible for this catabolism. Attempts to study DBT as C source have been unsuccessful partly because of the very low solubility of DBT relative to C demand, and partly because expression of the main known desulfurization genes is repressed by the excess of sulfate generated in the 4S pathway. The production of benzoate from DBTO sulfoxide/sulfone and its further degradation has been proposed in Brevibacterium (Van Afferden et al., 1990) and Arthrobacter DBTS2 (Sato and Clark, 1996). The introduction of the carABC operon of Pseudomonas CA10 in the DBT desulfurizer Rhodococcus XP strain suggested that the carbazole-degrading enzymes can attack the 2HBP as it was possible to detect benzoate in the medium (Yu et al., 2006). Different isolates of the genus Bacillus (Buzanello et al., 2014) and Cobetia (Ibacache-Quiroga et al., 2013) were able to grow in a medium supplemented with DBT as carbon source. Using benzoate as carbon source, Enterobacter sp. strain NISOC-03 was able to use DBT and produce 2HBP during the exponential growth phase and subsequently degrade it in the stationary growth phase, but the metabolic pathway involved has not been elucidated (Papizadeh et al., 2017). Recently a multiple metabolic pathway, with the production of at least twenty-six different metabolites, was proposed for the degradation of DBT in Pseudomonas sp. LKY-5 (Li et al., 2019b). The synthetic pathway generated in P. azelaica AJdsz (Figure 1) constitutes the best characterized mechanism of DBT degradation reported so far, and it could be easily expanded to and tested in other 2HBP bacterial degraders.
Conclusion
We have assembled the first synthetic pathway to completely metabolize DBT. The expression of the 4S pathway dsz genes in a 2HBP-degrading bacterium (P. azelaica Aramco J strain) was shown to mitigate a major bottleneck, i.e., the 2HBP-mediated inhibition of Dsz enzymes, hence significantly improving the yield of DBT biodesulfurization. Moreover, the P. azelaica AJdsz strain becomes also a suitable biocatalyst not only to completely degrade DBT for the bioremediation of polyaromatic sulfur heterocycles-contaminated sites and sediments, but also to transform it into value-added compounds taking advantage of the complex metabolism present in P. azelaica Aramco J. Future work should be performed to enhance DBT metabolism for real-world applications, e.g., evaluating the biodesulfurization process of crude oil in biphasic systems or the remediation of crude in contaminated sites.
Data availability statement
The original contributions presented in the study are included in the article/supplementary material, further inquiries can be directed to the corresponding author.
Author contributions
IM performed the experiments. IM, MM, JG, and ED designed the experiments, contributed to the discussion, and interpretation of the data. IM, JG, and ED wrote the article. All authors contributed to the article and approved the submitted version.
Funding
This work was supported by Saudi Aramco, and by grants BIO2016-79736-R, RTI2018-095584-B-C44, PID2019-110612RB-I00, and PCI2019-111833-2 from the Ministry of Science and Innovation of Spain; by grant CSIC 2019 20E005, and by European Union H2020 Grant 101000733.
Acknowledgments
We thank A. Valencia for technical assistance and Secugen S. L. for DNA sequencing. We acknowledge support of the publication fee by the CSIC Open Access Publication Support Initiative through its Unit of Information Resources for Research (URICI).
Conflict of interest
Authors declare that the research was conducted in the absence of any commercial or financial relationships that could be construed as a potential conflict of interest.
Publisher’s note
All claims expressed in this article are solely those of the authors and do not necessarily represent those of their affiliated organizations, or those of the publisher, the editors and the reviewers. Any product that may be evaluated in this article, or claim that may be made by its manufacturer, is not guaranteed or endorsed by the publisher.
References
Abin-Fuentes, A., El-Said Mohamed, M., Wang, D. I. C., and Prather, K. L. J. (2013). Exploring the mechanism of biocatalyst inhibition in microbial desulfurization. Appl. Environ. Microbiol. 79, 7807–7817. doi: 10.1128/AEM.02696-13
Akhtar, N., Akhtar, K., and Ghauri, M. A. (2018). Biodesulfurization of thiophenic compounds by a 2-hydroxybiphenyl-resistant Gordonia sp. HS126-4N carrying dszABC genes. Curr. Microbiol. 75, 597–603. doi: 10.1007/s00284-017-1422-8
Akhtar, N., Ghauri, M. A., Anwar, M. A., and Akhtar, K. (2009). Analysis of the dibenzothiophene metabolic pathway in a newly isolated Rhodococcus spp. FEMS Microbiol. Lett. 301, 95–102. doi: 10.1111/j.1574-6968.2009.01797.x
Bordoloi, N. K., Bhagowati, P., Chaudhuri, M. K., and Mukherjee, A. K. (2016). Proteomics and metabolomics analyses to elucidate the desulfurization pathway of Chelatococcus sp. PLoS One 11:e0153547. doi: 10.1371/journal.pone.0153547
Bordoloi, N. K., Rai, S. K., Chaudhuri, M. K., and Mukherjee, A. K. (2014). Deep-desulfurization of dibenzothiophene and its derivatives present in diesel oil by a newly isolated bacterium Achromobacter sp. to reduce the environmental pollution from fossil fuel combustion. Fuel Process. Technol. 119, 236–244. doi: 10.1016/j.fuproc.2013.10.014
Buzanello, E. B., Rezende, R. P., Sousa, F. M. O., Marques, E. L. S., and Loguercio, L. L. (2014). A novel Bacillus pumilus-related strain from tropical landfarm soil is capable of rapid dibenzothiophene degradation and biodesulfurization. BMC Microbiol. 14:257. doi: 10.1186/s12866-014-0257-8
Carraro, N., Sentchilo, V., Polák, L., Bertelli, C., and van der Meer, J. R. (2020). Insights into mobile genetic elements of the biocide-degrading bacterium Pseudomonas nitroreducens HBP-1. Genes 11:930. doi: 10.3390/genes11080930
Chen, H., Cai, Y. B., Zhang, W. J., and Li, W. (2009). Methoxylation pathway in biodesulfurization of model organic compounds with Mycobacterium sp. Bioresour. Technol. 100, 2085–2087. doi: 10.1016/j.biortech.2008.10.010
Chen, H., Zhang, W. J., Cai, Y. B., Zhang, Y. M., and Li, W. (2008). Elucidation of 2-hydroxybiphenyl effect on dibenzothiophene desulfurizaiton by Microbacterium sp. strain ZD-M2. Bioresour. Technol. 99, 6928–6933. doi: 10.1016/j.biortech.2008.01.033
Czechowska, K., Reimmann, C., and van der Meer, J. R. (2013). Characterization of a MexAB-OprM efflux system necessary for productive metabolism of Pseudomonas azelaica HBP1 on 2-hydroxybiphenyl. Front. Microbiol. 4:203. doi: 10.3389/fmicb.2013.00203
Feng, S., Yan, G. H., Zhan, X., and Wang, W. (2016). Enhancement of dibenzothiophene biodesulfurization by weakening the feedback inhibition effects based on a systematic understanding of the biodesulfurization mechanism by Gordonia sp. through the potential "4S" pathway. RSC Adv. 6, 82872–82881. doi: 10.1039/C6RA14459D
Galán, B., Díaz, E., and García, J. L. (2000). Enhancing desulphurization by engineering a flavin reductase-encoding gene cassette in recombinant biocatalysts. Environ. Microbiol. 2, 687–694. doi: 10.1046/j.1462-2920.2000.00151.x
Gupta, N., Roychoudhury, P. K., and Deb, J. K. (2005). Biotechnology of desulfurization of diesel: prospects and perspectives. Appl. Microbiol. Biotechnol. 66, 356–366. doi: 10.1007/s00253-004-1755-7
Hirschler, A., Carapito, C., Maurer, L., Zumsteg, J., Villette, C., Heintz, D., et al. (2021). Biodesulfurization induces reprogramming of sulfur metabolism in Rhodococcus qingshengii IGTS8: proteomics and untargeted metabolomics. Microbiol. Spectr. 9:e0069221. doi: 10.1128/Spectrum.00692-21
Ibacache-Quiroga, C., Ojeda, J., Espinoza-Vergara, G., Olivero, P., Cuellar, M., and Dinamarca, M. A. (2013). The hydrocarbon-degrading marine bacterium Cobetia sp. strain MM1IDA2H-1 produces a biosurfactant that interferes with quorum sensing of fish pathogens by signal hijacking. Microb. Biotechnol. 6, 394–405. doi: 10.1111/1751-7915.12016
Jaspers, M. C. M., Schmid, A., Sturme, M. H. J., Goslings, D. A. M., Kohler, H. P. E., and van der Meer, J. R. (2001). Transcriptional organization and dynamic expression of the hbpCAD genes, which encode the first three enzymes for 2-hydroxybiphenyl degradation in Pseudomonas azelaica HBP1. J. Bacteriol. 183, 270–279. doi: 10.1128/JB.183-1.270-279.2001
Kilbane, J. J. (2017). Biodesulfurization: how to make it work? Arab. J. Sci. Eng. 42, 1–9. doi: 10.1007/s13369-016-2269-1
Kirimura, K., Furuya, T., Nishii, Y., Ishii, Y., Kino, K., and Usami, S. (2001). Biodesulfurization of dibenzothiophene and its derivatives through the selective cleavage of carbon sulfur bonds by a moderately thermophilic bacterium Bacillus subtilis Wu-S2B. J. Biosci. Bioeng. 91, 262–266. doi: 10.1016/S1389-1723(01)80131-6
Li, L., Liao, Y., Luo, Y., Zhang, G., Liao, X., Zhang, W., et al. (2019a). Improved efficiency of the desulfurization of oil sulfur compounds in Escherichia coli using a combination of desensitization engineering and DszC overexpression. ACS Synth. Biol. 8, 1441–1451. doi: 10.1021/acssynbio.9b00126
Li, L., Shen, X., Zhao, C., Liu, Q., Liu, X., and Wu, Y. (2019b). Biodegradation of dibenzothiophene by efficient Pseudomonas sp. LKY-5 with the production of a biosurfactant. Ecotoxicol. Environ. Saf. 176, 50–57. doi: 10.1016/j.ecoenv.2019.03.070
Li, M. J., Wang, T.-G., Simoneit, B. R. T., Shi, S. B., Zhang, L. W., and Yang, F. L. (2012). Qualitative and quantitative analysis of dibenzothiophene, its methylated homologues, and benzonaphthothiophenes in crude oils, coal, and sediment extracts. J. Chromatogr. A 1233, 126–136. doi: 10.1016/j.chroma.2012.01.086
Li, F. L., Xu, P., Ma, C. Q., Luo, L. L., and Wang, X. S. (2003). Deep desulfurization of a hydrodesulfurization-treated diesel oil by a facultative thermophilic Mycobacterium sp. X7B. FEMS Microbiol. Lett. 223, 301–307. doi: 10.1016/S0378-1097(03)00397-5
Martín, A. B., Alcón, A., Santos, V. E., and García-Ochoa, F. (2004). Production of a biocatalyst of Pseudomonas putida CECT5279 for dibenzothiophene biodesulfurization for different media compositions. Energ. Fuel 18, 851–857. doi: 10.1021/ef030174c
Martín-Cabello, G., Terrón-González, L., and Santero, E. (2022). Characterization of a dszEABC operon providing fast growth on dibenzothiophene and construction of broad-host-range biodesulfurization catalysts. Environ. Microbiol. 24, 1946–1963. doi: 10.1111/1462-2920.15951
Martínez, I., Mohamed, M. E. S., Rozas, D., García, J. L., and Díaz, E. (2016). Engineering synthetic bacterial consortia for enhanced desulfurization and revalorization of oil sulfur compounds. Metab. Eng. 35, 46–54. doi: 10.1016/j.ymben.2016.01.005
Martínez, I., Mohamed, M. E. S., Santos, V. E., García, J. L., García-Ochoa, F., and Díaz, E. (2017). Metabolic and process engineering for biodesulfurization in gram-negative bacteria. J. Biotechnol. 262, 47–55. doi: 10.1016/j.jbiotec.2017.09.004
Miller, J. H. (1972). Experiments in Molecular Genetics. Cold Spring Harbor, NY: Cold Spring Harbor Laboratory Press.
Mohamed, M. E. S., García, J. L., Martínez, I., del Cerro, C., Nogales, J., and Díaz, E. (2015). Genome sequence of Pseudomonas azelaica strain Aramco J. Genome. Announc. 3:e00037-15. doi: 10.1128/genomeA.00037-15
Mohebali, G., and Ball, A. G. (2016). Biodesulfurization of diesel fuels - past, present and future perspectives. Int. Biodeter. Biodegrad. 110, 163–180. doi: 10.1016/j.ibiod.2016.03.011
Monticello, D. J. (2000). Biodesulfurization and the upgrading of petroleum distillates. Curr. Opin. Biotechnol. 11, 540–546. doi: 10.1016/S0958-1669(00)00154-3
Papizadeh, M., Ardakani, M., and Motamedi, H. (2017). Growth-phase dependent biodesulfurization of dibenzothiophene by Enterobacter sp. strain NISOC-03. Pollution 3, 101–111. doi: 10.22059/POLL.2017.59576
Parveen, S., Akhtar, N., Ghauri, M. A., and Akhtar, K. (2020). Conventional genetic manipulation of desulfurizing bacteria and prospects of using CRISPR-Cas systems for enhanced desulfurization activity. Crit. Rev. Microbiol. 46, 300–320. doi: 10.1080/1040841X.2020.1772195
Sambrook, J., and Russell, D. W. (2001). Molecular Cloning: A laboratory Manual. Cold Spring Harbor Laboratory Press. Cold Spring Harbor, New York
Sato, H., and Clark, D. P. (1996). Degradation of dibenzothiophene sulphoxide and sulphone by Arthrobacter strain DBTS2. Microbios 83, 145–159.
Schäfer, A., Tauch, A., Jäger, W., Kalinowski, J., Thierbach, G., and Pühler, A. (1994). Small mobilizable multi-purpose cloning vectors derived from Escherichia coli plasmids pK18 and pK19: selection of defined deletions in the chromosome of Corynebacterium glutamicum. Gene 145, 69–73. doi: 10.1016/0378-1119(94)90324-7
Soleimani, M., Bassi, A., and Margaritis, A. (2007). Biodesulfurization of refractory organic sulfur compounds in fossil fuels. Biotechnol. Adv. 25, 570–596. doi: 10.1016/j.biotechadv.2007.07.003
Van Afferden, M., Schacht, S., Klein, J., and Trüper, H. G. (1990). Degradation of dibenzothiophene by Brevibacterium sp. DO. Arch. Microbiol 153, 324–328. doi: 10.1007/BF00249000
Xu, P., Yu, B., Li, F., Cai, X. F., and Ma, C. Q. (2006). Microbial degradation of sulfur, nitrogen and oxygen heterocycles. Trends Microbiol. 14, 398–405. doi: 10.1016/j.tim.2006.07.002
Keywords: Pseudomonas azelaica, dibenzothiophene, metabolic engineering, biodesulfurization, 4S pathway
Citation: Martínez I, Mohamed ME-S, García JL and Díaz E (2022) Enhancing biodesulfurization by engineering a synthetic dibenzothiophene mineralization pathway. Front. Microbiol. 13:987084. doi: 10.3389/fmicb.2022.987084
Edited by:
Wael Ahmed Ismail, Arabian Gulf University, BahrainReviewed by:
Michael Kertesz, The University of Sydney, AustraliaHongzhi Tang, Shanghai Jiao Tong University, China
Dimitris G. Hatzinikolaou, National and Kapodistrian University of Athens, Greece
Copyright © 2022 Martínez, Mohamed, García and Díaz. This is an open-access article distributed under the terms of the Creative Commons Attribution License (CC BY). The use, distribution or reproduction in other forums is permitted, provided the original author(s) and the copyright owner(s) are credited and that the original publication in this journal is cited, in accordance with accepted academic practice. No use, distribution or reproduction is permitted which does not comply with these terms.
*Correspondence: Eduardo Díaz, ediaz@cib.csic.es
†Present Address: Igor Martínez,Department of Research and Development, PharmaMar S.A., Madrid, Spain