- Beijing Laboratory for Food Quality and Safety, College of Food Science and Nutritional Engineering, China Agricultural University, Beijing, China
Polymyxin is the “last resort” of antibiotics. The self-induced resistance to polymyxin in Gram-negative bacteria could be mediated by lipopolysaccharide (LPS) modification, which is regulated by the two-component system, PhoP/PhoQ. Yersinia enterocolitica is a common foodborne pathogen. However, PhoP/PhoQ has not been thoroughly studied in Y. enterocolitica. In this study, the functions of PhoP/PhoQ in Y. enterocolitica intrinsic resistance were investigated. The resistance of Y. enterocolitica was found to decrease with the deletion of PhoP/PhoQ. Further, PhoP/PhoQ was found to play an important role in maintaining membrane permeability, intercellular metabolism, and reducing membrane depolarization. Based on subsequent studies, the binding ability of polymyxin to Y. enterocolitica was decreased by the modification of LPS with structures, such as L-Ara4N and palmitate. Analysis of the gene transcription levels revealed that the LPS modification genes, pagP and arn operon, were downregulated with the deletion of PhoP/PhoQ in Y. enterocolitica during exposure to polymyxin. In addition, pmrA, pmrB, and eptA were downregulated in the mutants compared with the wild-type strain. Such findings demonstrate that PhoP/PhoQ contributes to the intrinsic resistance of Y. enterocolitica toward polymyxins. LPS modification with L-Ara4N or palmitate is mainly responsible for the resistance of Y. enterocolitica to polymyxins. The transcription of genes related to LPS modification and PmrA/PmrB can be both affected by PhoP/PhoQ in Y. enterocolitica. This study adds to current knowledge regarding the role of PhoP/PhoQ in intrinsic resistance of Y. enterocolitica to polymyxin.
Introduction
Yersinia enterocolitica is a common foodborne pathogen that causes a broad range of gastrointestinal syndromes in humans (yersiniosis; Shoaib et al., 2019). Yersinia enterocolitica is widely distributed in various environments and food production (Shoaib et al., 2019). To infect humans, Y. enterocolitica must survive in the host environment by escaping cationic antibiotic peptides (AMPs), which mediate parts of the innate immune system against infections (Rahnamaeian, 2011). In bacteria, two-component systems (TCSs) are employed to sense and respond to various environmental stresses (West and Stock, 2001). Typical TCSs consist of a membrane-embedded sensor kinase and a cytosolic response regulator. These systems can sense extracellular signals and perform cascade phosphorylation in response. The activated response regulator then regulates the expression of specific genes directly or indirectly, thereby initiating cellular responses to environmental changes (Hoch, 2000; West and Stock, 2001).
PhoP/PhoQ is a prototypical TCS present in many bacteria. PhoQ, the dimeric sensor kinase of this system, contains cytoplasmic, transmembrane, and periplasmic domains (Véscovi et al., 1997; Bader et al., 2005; Prost et al., 2007). The patch of acidic residues close to the membrane surface in the periplasmic sensing domain of PhoQ is crucial for sensing cationic AMPs (Bader et al., 2005). Divalent cation concentrations, low pH conditions, and high osmolarity have been reported to activate PhoQ (Véscovi et al., 1997; Bader et al., 2005; Prost et al., 2007). And PhoP is a phosphorylated response regulator which is controlled by activated PhoQ (Simpson and Trent, 2019). PhoP directly regulates a set of genes that vary among different bacteria (Winfield et al., 2005; Hong et al., 2018; Chen et al., 2021). However, some Gram-negative bacteria have conserved ancestral genes, such as mgtA in Escherichia coli acting as magnesium transporter and mgrB as the direct negative regulator of PhoP/PhoQ (Minagawa et al., 2003; Zwir et al., 2012). The protein, PmrD, which is also directly regulated by PhoP, could combine PhoP/PhoQ and PmrA/PmrB. Thus, PhoP/PhoQ is indirectly involved in PmrA/PmrB regulation of genes (Figures 1A,B; Rubin et al., 2015; Hong et al., 2018).
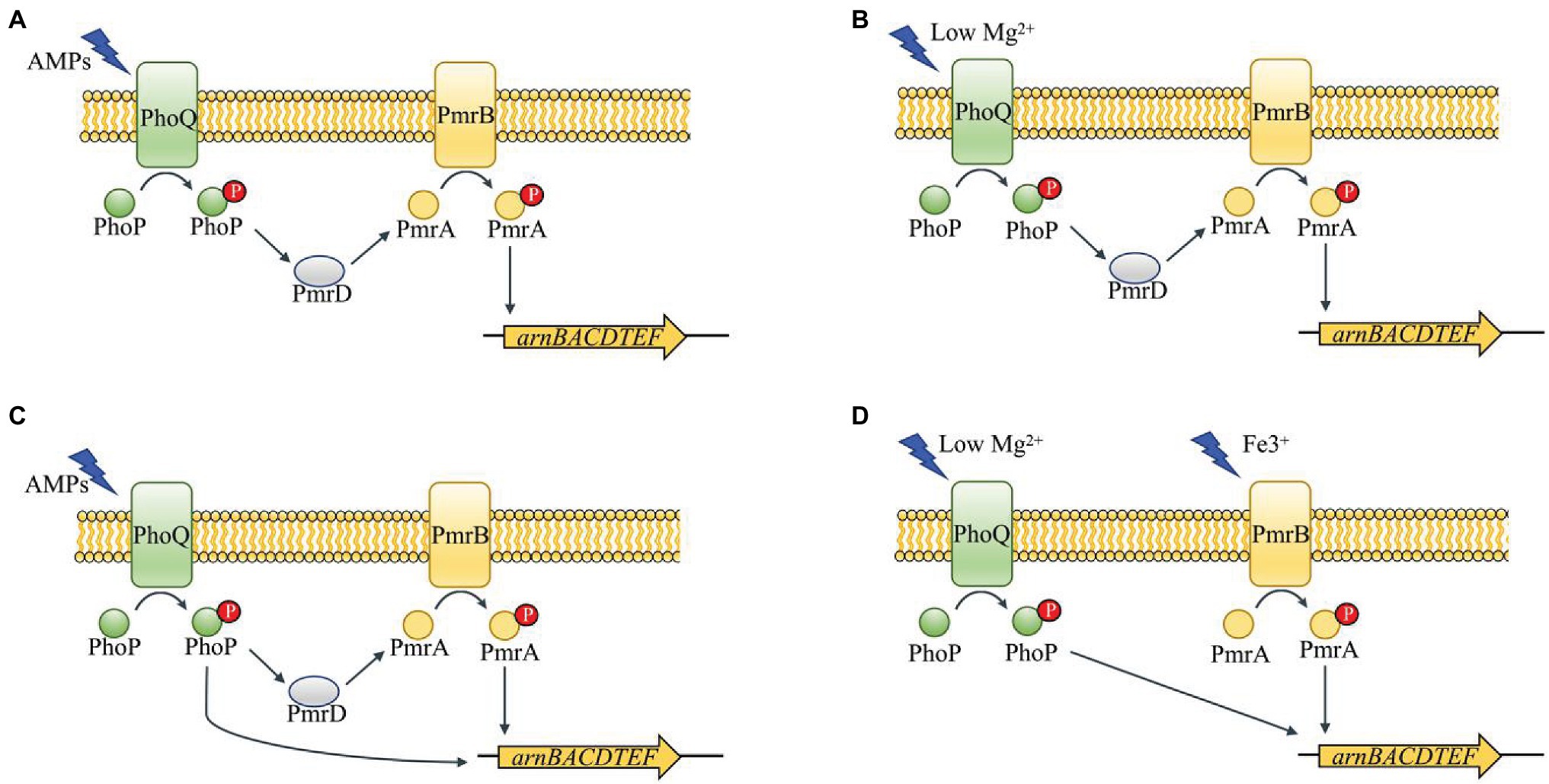
Figure 1. Schematic of PhoP/PhoQ and PmrA/PmrB regulation network in different genera of Enterobacteriaceae (Winfield et al., 2005; Rubin et al., 2015; Hong et al., 2018; Chen et al., 2021). PmrD functions as a connector between PhoP/PhoQ and PmrA/PmrB in Salmonella enterica (A). The connector function of PmrD is activated under low Mg2+ in Escherichia coli (B). PhoP can regulate arn operon directly and indirectly in Klebsiella pneumoniae (C). PhoP/PhoQ and PmrA/PmrB perform a cross-regulation of arn operon under different environments in Yersinia pestis (D).
Polymyxins are AMPs that target Gram-negative outer membranes (Mitchell and Silhavy, 2019). Lipid A of Gram-negative bacterial outer membrane is the target binding site for AMPs, which mediates the interaction with negatively charged LPS. A hydrophobic domain is known to insert itself into membranes, forming pores, which results in cell lysis and death (Brogden, 2005). The resistance of Gram-negative bacteria to polymyxins primarily relies on chemical modifications of the lipopolysaccharide (LPS) structure and leads to major changes in the physicochemical properties of the outer membrane (Trimble et al., 2016). In most resistant strains, LPS modified with 4-amino-4-deoxy-L-arabinose (L-Ara4N), phosphoethanolamine (pEtN), as well as other modification substituents, showed polymyxin resistance (Santos et al., 2017). Expression of most of the genes in the LPS modification pathway is controlled by several TCSs, including PhoP/PhoQ and PmrA/PmrB (Rubin et al., 2015; Trimble et al., 2016; Duperthuy, 2020).
Although PhoP/PhoQ has been best studied in Salmonella typhimurium, it has not been thoroughly studied in Y. enterocolitica. Further, some proteins such as PmrD in PhoP/PhoQ regulatory pathway in Salmonella have not been found in Y. enterocolitica. Therefore, whether the resistance of Y. enterocolitica to polymyxins could be affected by the deletion of PhoP/PhoQ needs to be determined. Further, the role of PhoP/PhoQ in the intrinsic resistance mechanism of Y. enterocolitica to AMP is unclear. In this study, the role of PhoP/PhoQ in the polymyxin resistance of Y. enterocolitica as well as the growth, membrane damage, and intracellular metabolism of Y. enterocolitica was identified. The difference in the ability of polymyxins to bind to resistant or sensitive membranes regulated by PhoP/PhoQ was assessed. In addition, Y. enterocolitica lipid A modifications implicated in polymyxin resistance were characterized. By analyzing gene expression, PhoP/PhoQ was found to affect polymyxin resistance by regulating various genes related to LPS modification. These findings reveal some of the roles of PhoP/PhoQ in Y. enterocolitica polymyxin resistance.
Materials and Methods
Bacterial Strains, Plasmids, and Culture Conditions
The bacterial strains and plasmids used in this study are listed in Table 1. Escherichia coli S17-λpir was used as the host bacteria in plasmid construction and was cultured at 37°C in lysogeny broth (LB) containing 5 g/L yeast extract, 10 g/L tryptone, and 5 g/L NaCl. Yersinia enterocolitica ATCC23715 (biotype 1 B and serotype O:8) was used as the parent strain for the construction of Y. enterocolitica mutants, which were cultured at 26°C in LB broth and LBNS (LB without salts). Ampicillin (100 μg/ml), chloramphenicol (16 μg/ml), cefsulodin (15 μg/ml), irgasan (4 μg/ml), and novobiocin (2.5 μg/ml) were added as required.
Construction of Plasmids and Strains
To construct pDS132-ΔphoP, fragments upstream and downstream of the phoP gene were amplified from the Y. enterocolitica genome using the primers, phoP-up-F/phoP-up-R and phoP-down-F/phoP-down-R. The upstream and downstream fragments were fused and amplified by fusion PCR with primers phoP-up-F/phoP-down-R. The resultant long fragment was then digested with SacI and SalI and ligated into pDS132 digested with the same enzymes to yield pDS132-ΔphoP. The same approach was used to construct pDS132-ΔphoQ with the corresponding primers. To construct the phoP knockout strain, the suicide plasmid, pDS132-ΔphoP, was introduced into E. coli S17-1 λpir by electroporation and then mobilized into Y. enterocolitica by conjugation. The strategy used for gene deletion in the Y. enterocolitica chromosome was based on the two-step homologous recombination with plasmid pDS132 containing the sacB counter-selectable marker and a chloramphenicol resistant marker, as described previously (Schäfer et al., 1994). Similarly, the plasmid pDS132-ΔphoQ was used to construct the ΔphoQ mutants.
To construct pBAD24-phoP, the phoP fragment was amplified from the Y. enterocolitica genome using primers p-phoP-F/p-phoP-R, digested with XbalI and HindIII, and inserted into pBAD24 digested with the same enzymes. The resultant pBAD24-phoP plasmid contained the phoP gene, which was controlled by the araBAD promoter (PBADphoP). The plasmid, pBAD24-phoP, was used to transform the ΔphoP mutant by electroporation to construct the ΔphoP-phoP complemented strain. The same method was also used to construct ΔphoQ-phoQ complemented strains. All primers used in this study are listed in Supplementary Table 1. Ampicillin (100 μg/ml) and L-arabinose (0.6 g/L) were added to maintain plasmid and induce the expression of phoP or phoQ, respectively.
Growth Curves Measurement
Growth curves of the strains were determined by measuring the OD600 at 60 min intervals over a period of 24 h. Overnight cultures of bacterial strains were inoculated into LB medium supplemented 0.5 μg/ml PMB or PME (at 1:50 dilution) and incubated at 26°C with shaking at 180 rpm. Growth curves under no PMB or PME were also measured for the control group. L-arabinose (0.6 g/L) was added to induce the expression of phoP or phoQ of complemented strains. The experiments were performed with three biological replicates.
Minimal Inhibitory Concentration and Minimal Bactericidal Concentration
The minimal inhibitory concentration (MIC) of PMB and PME were assessed according to the CLSI guidelines with some modifications (CLSI, 2017). Briefly, overnight cultures of WT, phoP, and phoQ mutants were diluted in cation-adjusted Mueller–Hinton broth (CaMHB) with approximately106 colony forming units (CFU)/ml in tubes. Then, the suspended bacteria were exposed to PMB or PME at 0.5, 1.0, 2.0, 4.0, 8.0, and 16.0 μg/ml. All tubes were incubated for 18–24 h at 26°C with shaking. The MIC was determined as the minimal concentration at which turbidity was not visible in comparison with the blank and minimal bactericidal concentration (MBC) was the minimal concentration which had no growth. And it was performed with three biological replicates.
Laser Scanning Confocal Microscope
The overnight cultures were inoculated to LBNS broth and grown at 26°C and 180 rpm to mid-log phase. The bacterial cells were collected and washed with PBS buffer for three times and then resuspended at a final optical density of OD600 = 1.0. The equal volume of bacterial suspension was mixed with PMB or PME solution (10 μg/ml) and incubated at 26°C for 2 h. Then, the solutions were centrifuged at 5,000 × g for 5 min and resuspended at the same volume with PBS. And cell viability after PMB or PME treatment was assessed using fluorescently labeled propidium iodide (PI) and 4′,6-diamidino-2-phenylindole (DAPI) and analyzed with a laser scanning confocal microscope (LSCM). DAPI is a blue, fluorescent stain that labels both live and dead cells while PI is a red fluorescent nucleic acid stain that only penetrates cells with damaged cell membranes (dead cells). All images were captured by LSCM (Leica TCS SP8, Mannheim, Germany) and quantified using ImageJ.
Cell Membrane Permeabilization and Potential
N-phenyl-1-naphthylamine (NPN) uptake assays were performed to evaluate the outer membrane permeability of Y. enterocolitica strains, as previously described (Lee and Je, 2013). Briefly, cells were harvested in the log phase of growth and centrifuged at 5,000 rpm for 10 min at 4°C. Thereafter, the cells were washed three times with PBS and resuspended to an OD600 of 0.5. An aliquot of bacterial suspension and polymyxin solutions were fully mixed with NPN at a final concentration of 10 μM. The fluorescence value was then measured immediately using a spectrofluorometer FS5 (Edinburgh Instruments, United Kingdom). Excitation and emission wavelengths for NPN were set at 350 and 420 nm, respectively, with a slit width of 0.5 nm. The inner membrane permeability was tested with O-nitrophenyl-β-D-galactoside (ONPG) according to the method previously mentioned (Lee and Je, 2013). Logarithmic-phase bacteria were washed with PBS and resuspended to OD600 at 0.5. Then, 100 μl of bacterial suspension was added to 100 μl polymyxin solutions and 10 μl ONPG (30 mM) in each well. And the absorbance at 410 nm was used to evaluate o-nitrophenol over time using a spectrophotometer.
Overnight cultures were inoculated to LBNS and grown at 180 rpm, 26°C to mid-log phase. And bacteria were washed with 20 mM HEPES buffer for three times at 4°C and adjust to OD600 = 0.5. Then, bacteria were exposed under 0.5 μg/ml PMB or PME for 2 h at 26°C. Changes in membrane polarity were detected using the bis-oxonol dye, DiBAC4(3) (AAT Bioquest, Inc., CA, United States). The assay was performed according to a previously described method with some modifications (Clementi et al., 2014).
Measurement of Intracellular Adenosine Triphosphate Concentration
Overnight cultures were inoculated to LBNS and grown at 180 rpm, 26°C to mid-log phase. Then, bacteria were washed with 20 mM HEPES buffer for three times at 4°C and adjust to OD600 = 0.5. Then, equal volume of bacteria suspension and 1 μg/ml PMB or PME solutions were incubate for 2 h at 26°C. The adenosine triphosphate (ATP) concentration of Y. enterocolitica after PMB or PME treatment was assayed using an ATP assay kit (Beyotime Biotechnology, Jiangsu, China). ATP remain rate was used which was calculated by dividing the ATP content of Y. enterocolitica treated with PMB or PME by the corresponding ATP content without PMB or PME treated.
Dansyl-Polymyxin Binding Experiments
Dansyl-PMB and dansyl-PME were prepared according to the modified method of Schindler and Teuber (Schindler and Teuber, 1975). The synthesized Dansyl-PMB and Dansyl-PME were dissolved in 3 ml of 1× PBS buffer (pH 7.0), purified using a Sephadex G-25 column, and quantitated using the dinitrophenylation assay (Bader and Teuber, 1973). The concentrations of dansyl-PMB and dansyl-PME were 0.519 and 0.409 mg/ml, respectively, relative to a triplicate standard curve derived from a 1 mg/ml stock solution of PMB and PME.
The fluorescence of dansyl-polymyxin bound to bacteria was measured using a spectrofluorometer FS5 (Edinburgh Instruments, United Kingdom), set at an excitation wavelength of 330 nm and an emission wavelength of 565 nm. To quantify Dansyl-PMB and Dansyl-PME binding, previous methods were used as reference, with some modifications (Cullen et al., 2011; Akhoundsadegh et al., 2019). All strains were grown to an OD600 of 0.8 to 1.0, and the cells were washed three times with PBS. Thereafter, the same volume of Dansyl-PMB or Dansyl-PME was applied to washed cells and incubated for 2 min. Following incubation, the cells were washed with PBS and resuspended in PBS. The fluorescence was determined by spectrofluorometer FS5 and A600 was determined using a microplate reader (Thermo Scientific, Waltham, MA, United States). Each experiment was repeated in triplicate, and data are reported as the ratio of fluorescence intensity to A600.
Lipid A Extraction and Analysis by MALDI-TOF
Yersinia enterocolitica cultures (200 ml) were grown to an A600 of 0.5 and then exposed to 1.0 μg/ml PMB or PME for 3 h. The cells were then harvested by centrifugation. Lipid A was purified from bacteria as described previously (Hankins et al., 2013; Henderson et al., 2013) and stored frozen at −20°C. The lipid A species were analyzed using a Bruker Atuoflex III MALDI-TOF mass spectrometer (Bruker Daltonics, Germany) according to a previous method (Hankins et al., 2013; Henderson et al., 2013; Han et al., 2018b).
RNA Extraction and Real-Time Quantitative PCR
Overnight cultures were inoculated to LBNS and grown at 180 rpm, 26°C for 3 h, and then exposed to 1.0 μg/ml PMB or PME for 3 h until OD600 was approximately at 0.5. Total RNA was extracted from Y. enterocolitica strains using the TransZol Up Plus RNA Kit (TransGen, Beijing, China). The extracted RNA was then tested for concentration and quality using a Nanodrop 2000c (Thermo Scientific, Waltham, United States). Real-time quantitative PCR (RT-qPCR) was conducted using SYBR Green and specific primers (Supplementary Table 2) in a Light Cycler 480 II (Roche, Basel, Switzerland). Reactions were performed in triplicate. Relative transcription of the target genes was analyzed by the 2–ΔΔCt method described previously, and the 16S rRNA gene was used as a reference for normalization (Meng et al., 2020).
Statistical Analysis
Statistical analysis was performed using GraphPad Prism 8 (GraphPad Software, San Diego, CA, United States). One-way ANOVA analysis was performed, and the levels of significance are indicated in the figure legends.
Results
Deletion of PhoP/PhoQ Decreases the Resistance of Yersinia enterocolitica to Polymyxins
Studies have shown that the PhoP/PhoQ system can respond to the presence of antimicrobial peptides and increase resistance (Shprung et al., 2012; Lin et al., 2018). To explore the role of the PhoP/PhoQ system in Y. enterocolitica exposed to polymyxins, MIC and MBC of wild-type and mutants were evaluated (Table 2). MIC to PMB and PME of wild type were both 2 μg/ml, while phoP or phoQ mutants was 1 μg/ml. And MBC were also decreased when PhoP/PhoQ was deleted in Y. enterocolitica. Then, the growth curves of wild-type, mutants, and complemented strains were also measured. The growth of Y. enterocolitica was not affected by the deletion of PhoP/PhoQ (Supplementary Figure 1). However, the lag phase of the growth curves was prolonged in phoP and phoQ mutants compared to the wild-type and complemented strains under 0.5 μg/ml PMB or PME (Figure 2). Both wild-type and mutant strains were stained with PI and DAPI after treatment with 5 μg/ml of PMB or PME and observed using LCSM (Supplementary Figure 2). The live-to-dead bacterial ratio was quantitatively analyzed, and the ratios of the mutants were lower than those of the wild type. Combined with the above evidence, the deletion of the PhoP/PhoQ system was found to decrease the resistance of Y. enterocolitica to PMB and PME and affected the growth of Y. enterocolitica under PMB or PME at sub-inhibitory concentration.
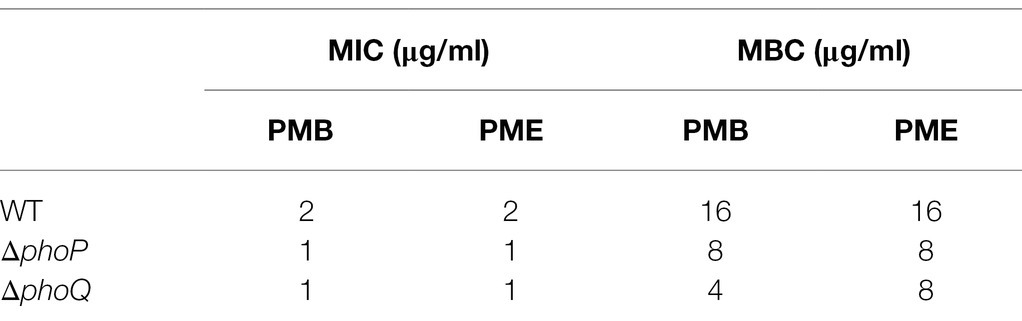
Table 2. Minimal inhibitory concentration (MIC) and minimal bactericidal concentration (MBC) of wild type, phoP and phoQ mutants to PMB and PME.
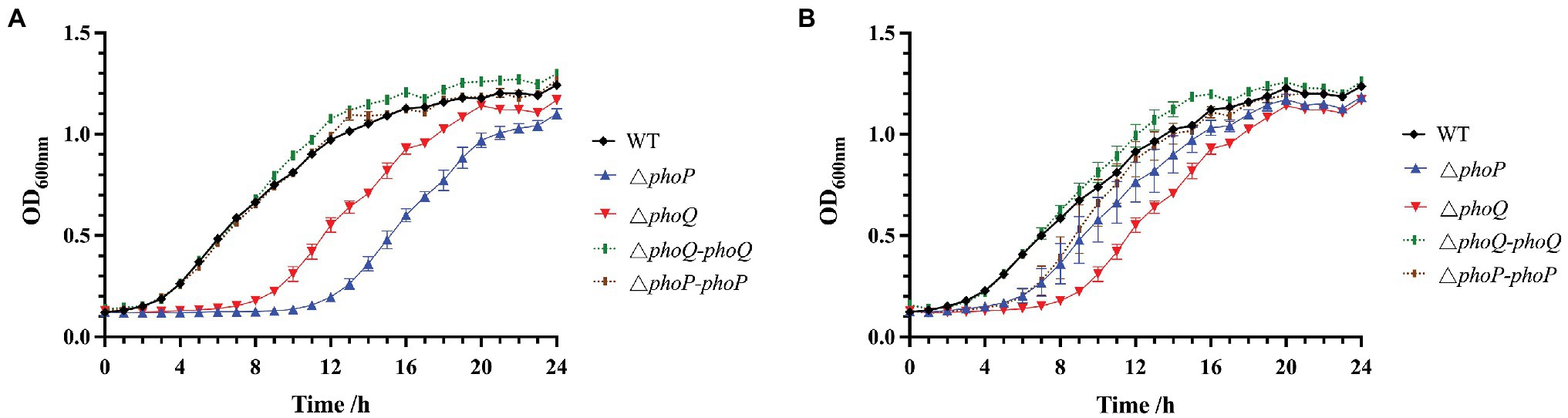
Figure 2. Effect of PhoP/PhoQ on the growth of Y. enterocolitica under PMB or PME. Growth curves of wild type, mutants and complemented strains under 0.5 μg/ml PMB (A). Growth curves of wild type, mutants and complemented strains under 0.5 μg/ml PME (B).
Deletion of PhoP/PhoQ Increases Membrane Sensitivity and Decreases Intracellular Metabolism When Exposed to Polymyxin
According to several reports, polymyxin can cause membrane damage, thereby interfering with the normal physiological functions of bacteria (Santos et al., 2017). Therefore, the changes in cell membrane potential and cell membrane permeability of the wild-type and mutant strains under sub-inhibitory concentrations of PMB or PME were explored. When PME and PMB were stimulated with 0.5 μg/ml, the permeability of the outer membrane and the changes in the membrane potential were measured, the results of which are displayed in Figures 3A,B. The NPN uptake method was used to evaluate the permeability of the outer membrane. NPN is a hydrophobic fluorescent probe that can be embedded in the cell membrane (Lee and Je, 2013). As its fluorescence intensity increases in a hydrophobic environment, it is often used to reflect the permeability of the cell membrane (Lee and Je, 2013; Akhoundsadegh et al., 2019). The fluorescence of the phoP and phoQ mutants was significantly higher than that of the wild type. In addition, the absorbance at 410 nm of the mutants was higher than that of the wild type under PMB or PME (Supplementary Figure 3). Thus, PMB or PME can be concluded to cause severe damage to the membrane permeability of mutants compared to the wild type. The membrane potential dye, DiBAC4(3), was used to measure the cellular membrane potential of Y. enterocolitica. At 0.5 μg/ml of PMB or PME, the membrane depolarization of the mutants was significantly enhanced compared with that of the wild type (Figures 3C,D).
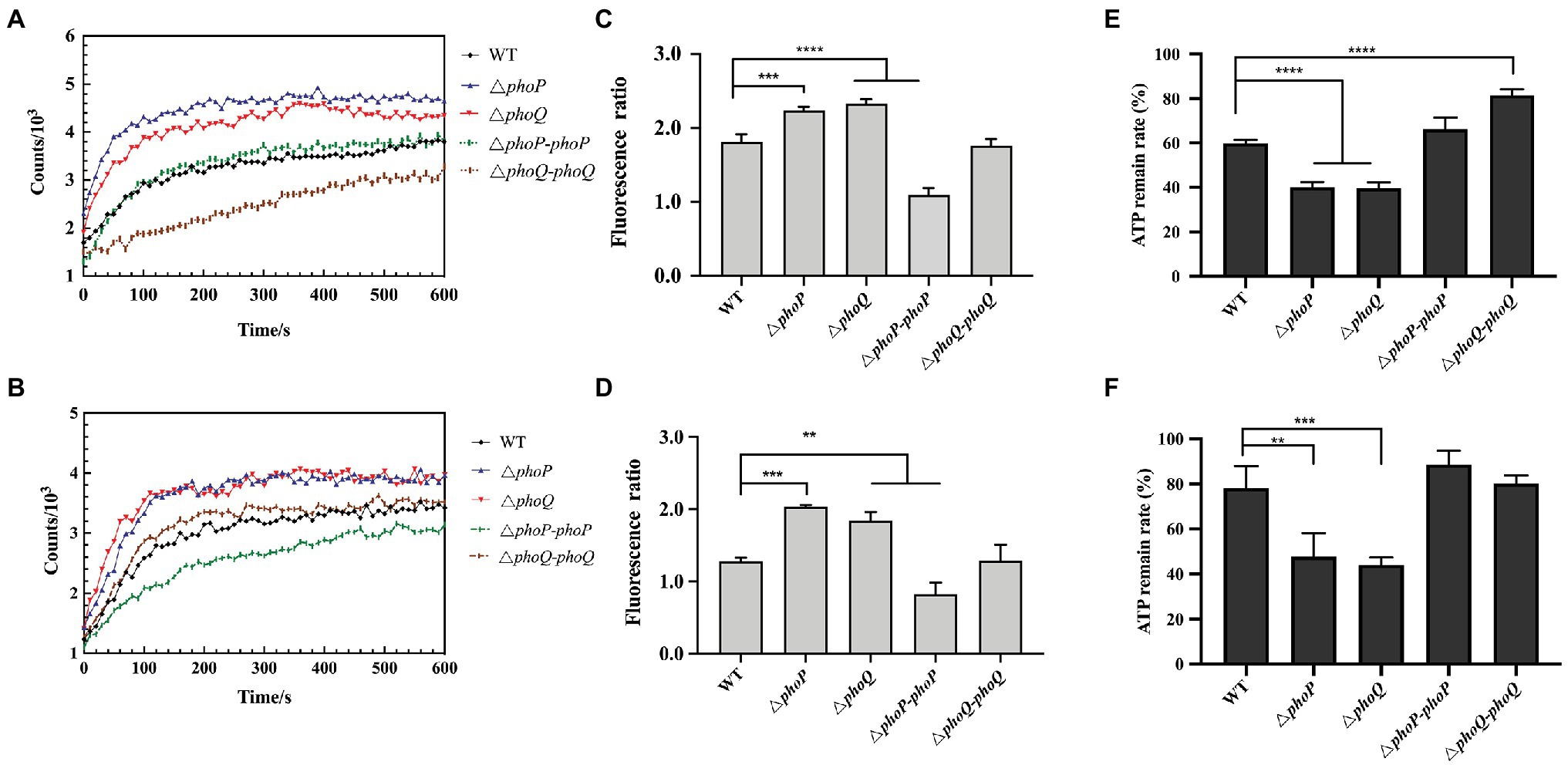
Figure 3. PhoP/PhoQ is essential for Y. enterocolitica to maintain membrane stability and intercellular metabolism when treated with 0.5 μg/ml PMB or PME. Outer membrane permeability evaluated by the N-phenyl-1-naphthylamine (NPN) uptake of wild type, mutants and complemented strains treated with 0.5 μg/ml PMB (A) and PME (B). Membrane depolarization of wild type, mutants and complemented strains treated with 0.5 μg/ml PMB (C) and PME (D) and measured using DiBAC4(3). The ordinate is the fluorescence ratio between the treatment group and the blank (untreated with PMB or PME). The intercellular ATP remain rate following treatment with 0.5 μg/ml PMB (E) and PME (F). Asterisks indicate a significant difference. ****, p < 0.0001; ***, p < 0.001; and **, p < 0.01.
Treatment with AMP can reduce cytoplasmic ATP concentration and inhibit respiration (Brogden, 2005). Bacterial resistance to PMB has also been reported to be strongly related to energy metabolism (Yu et al., 2019). To further explore whether the PhoP/PhoQ system influences the intracellular metabolism of Y. enterocolitica under stimulation with the same sub-inhibitory concentration, the intracellular ATP contents of mutants and wild type were determined at 0.5 μg/ml PMB or PME, and the results are shown in Figures 3E,F. The intracellular ATP content of wild type and mutants were reduced after PMB or PME treatment, but the mutants showed a significantly higher degree of reduction when compared with the wild type. Therefore, the resistance of Y. enterocolitica to PMB or PME after the deletion of the PhoP/PhoQ system was observed, which was demonstrated by more severe changes in cell membrane permeability, membrane depolarization, and intracellular metabolism.
PhoP/PhoQ Is Responsible for the Increased Resistance of Cell Membrane to Polymyxin Binding
Polymyxin binding to membrane promote cation displacement and structural changes in membrane curvature. And this binding is selectively which determines the sensitivity or resistance of bacteria to AMPs (Santos et al., 2017). The interactions between polymyxins and the Gram-negative outer membrane play an important role in polymyxin resistance (Brogden, 2005). Thus, the following hypothesis was proposed: the existence of the PhoP/PhoQ system can increase the resistance to polymyxins by reducing the binding and/or entry ability of polymyxin to Y. enterocolitica. To prove this hypothesis, PMB or PME binding assays were performed using the fluorescently labeled polymyxin compounds, Dansyl-PMB and Dansyl-PME. The quantification results of the binding or entry ability of Dansyl-PMB and Dansyl-PME with Y. enterocolitica are presented in Figure 4. First, the fluorescence intensity increased with increasing concentrations of Dansyl-PMB or Dansyl-PME, indicating increased surface-bound or entry of Dansyl-PMB or Dansyl-PME. No significant difference in fluorescence was found between the wild type and mutants when dansyl-PMB and dansyl-PME concentrations of 5 and 4 μg/ml were, respectively, employed. However, phoP and phoQ mutants showed significantly increased fluorescence compared to the wild type when the concentrations of dansyl-PMB and dansyl-PME were 50, 500, 40, and 400 μg/ml, respectively, confirming the protective effect of PhoP/PhoQ against polymyxin attack on the bacterial surface of Y. enterocolitica. Therefore, these results proved our hypothesis that the existence of PhoP/PhoQ provides more resistance to polymyxin binding in the cell membrane of Y. enterocolitica.
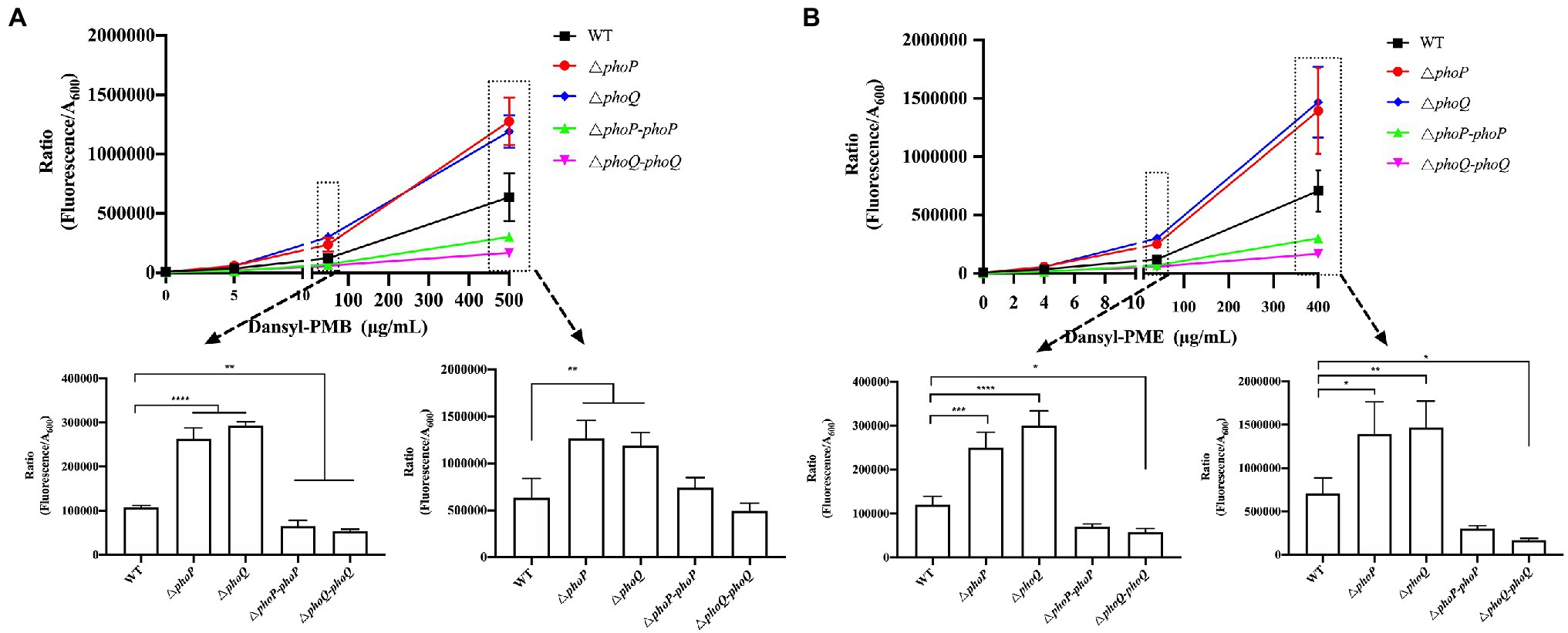
Figure 4. PhoP/PhoQ created a more resistant Y. enterocolitica membrane for the binding of polymyxin. The binding ability of different concentrations of Dansyl-PMB (A) and Dansyl-PME (B) to wild type, mutants and complemented strains. Asterisks indicate a significant difference. ****, p < 0.0001; ***, p < 0.001; **, p < 0.01; and *, p < 0.05.
LPS Structure, Which Is Associated With AMP Resistance, Depends on the Regulation of PhoP/PhoQ
The interaction of AMPs with the anionic bacterial surface is necessary for the exertion of their microbicidal action (Brogden, 2005). LPS modifications that reduce the negative charge or alter the acyl chains of lipid A provide resistance against AMP binding through charge repulsion or decrease the fluidity of the outer membrane (Simpson and Trent, 2019). To further explore the relationship between the binding ability of AMPs to the outer membrane of Y. enterocolitica and LPS modification, MALDI-TOF mass spectrometry was used to characterize the lipid A structure. Figure 5Aa shows that lipid A from Y. enterocolitica exposed to PMB contained a predominant peak at m/z 1,224. And three peaks were also found at m/z 1,864, m/z 1,708, and m/z 1,724 which contained two glucosamines, one or two phosphates, 2-OH-C12, and aminoarabinose (Figure 5B; Reinés et al., 2012a,b; Han et al., 2018a). The wild type grown under the PME showed a predominant peak at m/z 1,823 for a lipid A species that predominantly contained hexa-acylated species that correspond to two glucosamines, two phosphates, four 3-OH-C14, one C12, and one C16:1 (Figure 5B; Reinés et al., 2012b). The presence of polymyxins caused Y. enterocolitica to induce palmitate and aminoarabinose lipid A modification, which increased the resistance to polymyxins. Lipid A from wild type and mutants also did not undergo pEtN modification under PMB and PME stimulation, which may be related to the absence of PmrD in Y. enterocolitica (Reinés et al., 2012b). In the presence of PMB or PME, modifications to lipid A by aminoarabinose or palmitate could be detected in Y. enterocolitica under the regulation of PhoP/PhoQ, which led to resistance in polymyxin binding to the membrane and higher resistance. It was showed that lipid A from phoP and phoQ mutants under PME lacked multiple modifications, which could explain the higher fluorescence of the polymyxin binding mutants. Similarly, the phoQ mutants treated with PMB also lacked multiple modifications. However, for phoP mutants under PMB, result was also shown that peak at m/z 1,686 which was closely to m/z 1,684 has also been detected. This reveals that other pathways may involve in the regulation of lipid A modifications. Although PmrD is absent in Y. enterocolitica, but whether the cross-regulation between PhoP/PhoQ and PmrA/PmrB is existence has not been proved, so the quantification of the transcription level of PmrA/PmrB was performed which may give some explanation.
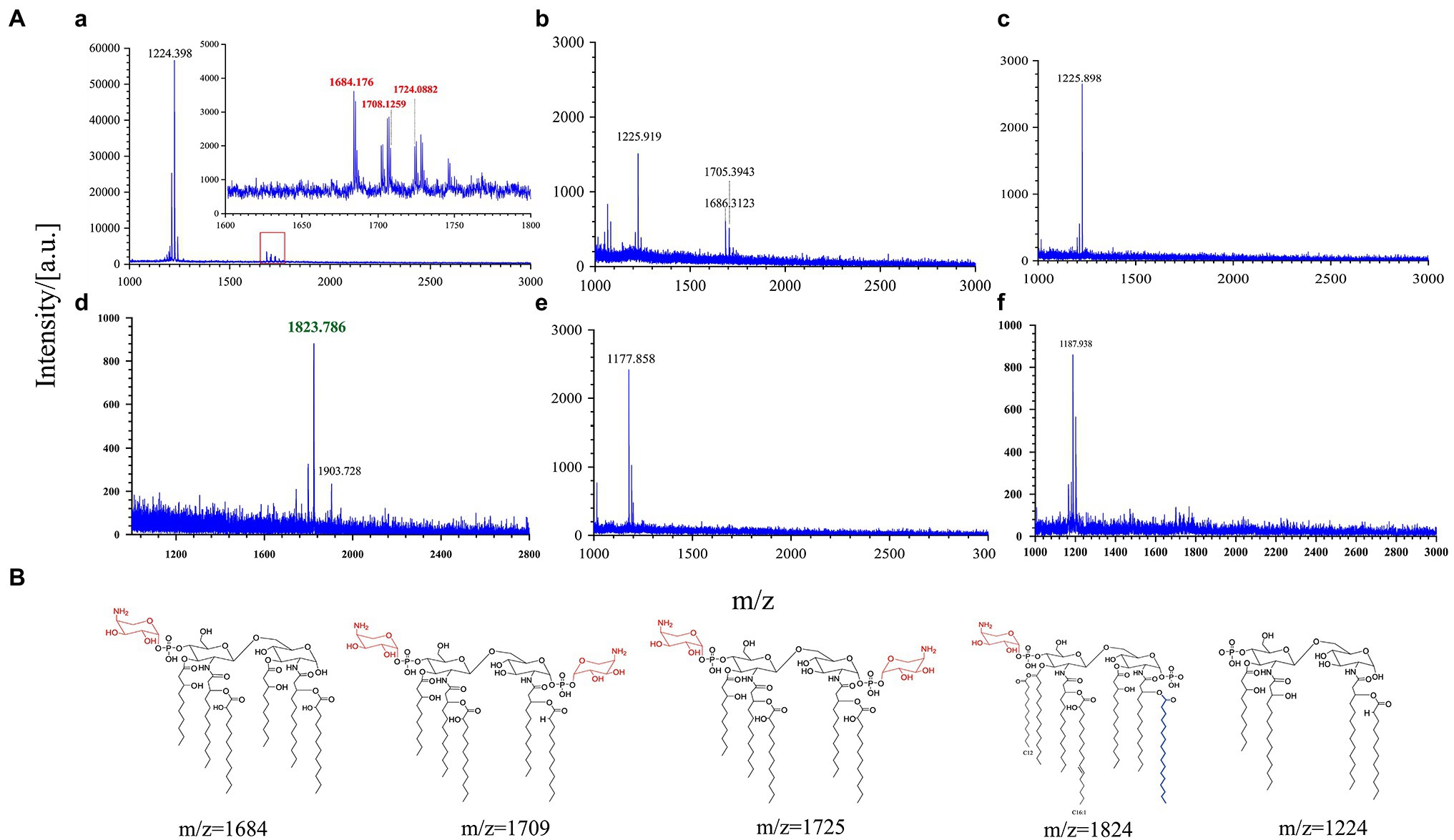
Figure 5. PhoP/PhoQ regulates Y. enterocolitica to form different lipopolysaccharide (LPS) modifications. MALDI-TOF analysis of wild type and mutants treated with PMB or PME (A). (a–c) Display the results of wild type, phoP mutants, and phoQ mutants treated with PMB and (d–f) display the results of wild type, phoP mutants, and phoQ mutants treated with PME. Possible structures of lipid A at different m/z (B).
Activated PhoP/PhoQ Upregulates Genes Related to LPS Modification in Yersinia enterocolitica
The above data confirmed that the presence of the PhoP/PhoQ system can increase the viability of Y. enterocolitica when exposed to PMB or PME. The increased resistance was achieved by reducing the binding ability of PMB or PME to Y. enterocolitica. The chemical characteristics of lipid A were characterized by MALDI-TOF, which revealed that wild-type Y. enterocolitica had a variety of structures related to AMP resistance compared to the mutants. Studies have shown that PhoP/PhoQ regulates pagP directly to maintain the asymmetry of the outer membrane (Simpson and Trent, 2019). Moreover, arnBCADTEF is required for the synthesis and addition of aminoarabinose to lipid A (Reinés et al., 2012a). Salmonella enterica subspp. and E. coli encode the protein, PmrD, which mediates coupling between PhoP/PhoQ and PmrA/PmrB (Rubin et al., 2015; Hong et al., 2018). Although PmrD was not found in Y. enterocolitica (Reinés et al., 2012b), the connections between PhoP/PhoQ and PmrA/PmrB can also be revealed using small RNAs, which remain unclear in Y. enterocolitica. In this study, the transcription levels of lipid A modification genes were analyzed to determine the regulatory effects of PhoP/PhoQ on lipid A modification (Figure 6). When exposed to PMB or PME at the MIC, the transcription levels of the genes, pagP and arnC, in the mutant strains were both reduced compared to those in the wild type. When exposed to PMB, pagP was downregulated by an average of 71 and 83% in the phoP and phoQ mutants, respectively, whereas arnC was downregulated by an average of 67 and 78%, respectively. The transcription levels of these genes in the ΔphoP-phoP and ΔphoQ-phoQ strains were restored. Exposed to PME, arnC was downregulated by an average of 32.4 and 30.8% in the phoP and phoQ mutants, respectively. And pagP was downregulated by an average of 42.5 and 36%, respectively, in phoP and phoQ mutants. There are differences of genes transcription between PMB and PME treatment. It may be caused by the differences of these two AMPs, as LPS modifications formed by Y. enterocolitica were also various under PMB and PME.
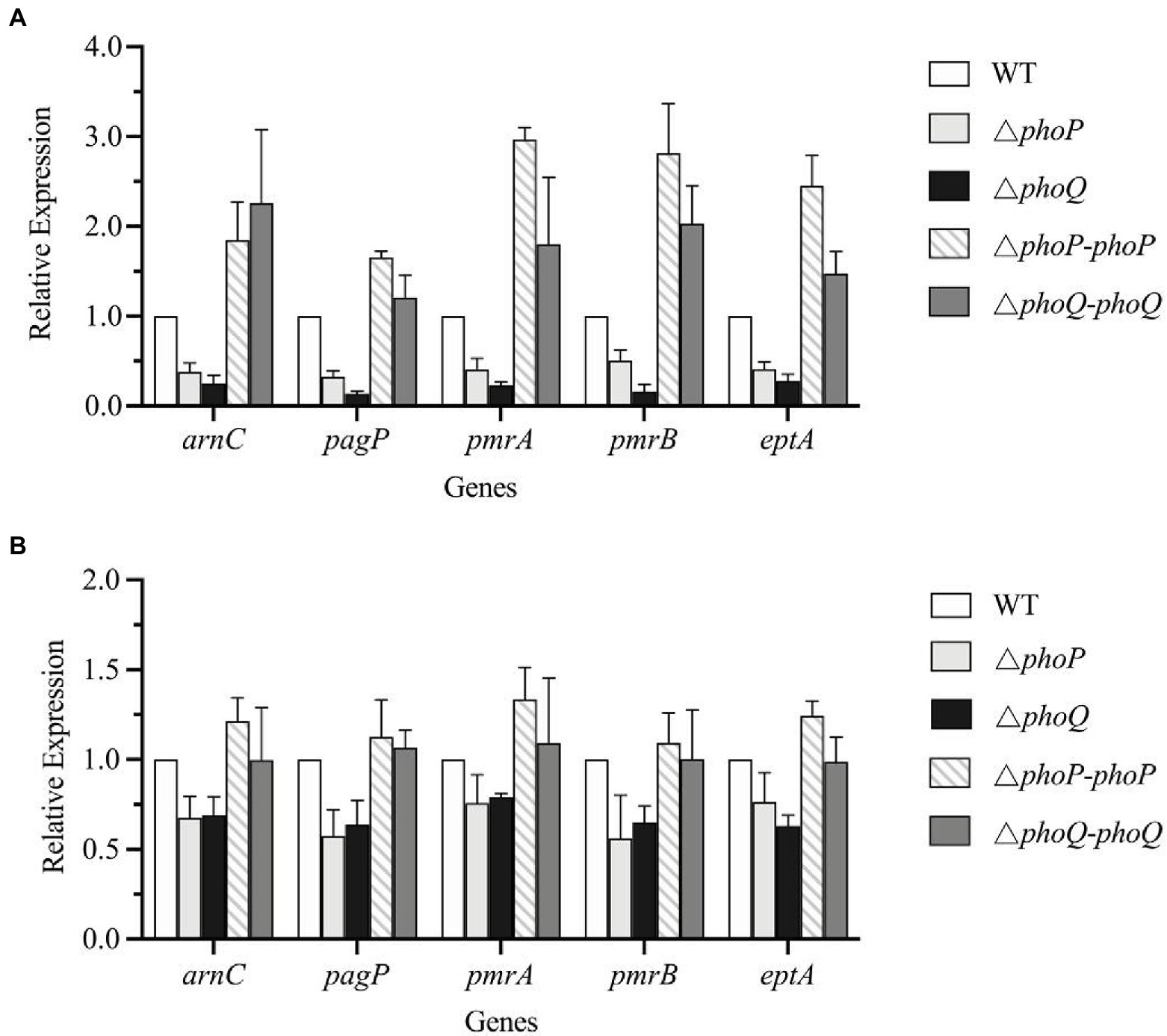
Figure 6. PhoP/PhoQ is involved in the regulation of genes related to LPS modification. Transcriptional changes of wild type, mutants and complemented strains treated with PMB (A) and PME (B).
Since the absent of PmrD, we speculated that PmrA/PmrB and PhoP/PhoQ independently regulated LPS modification pathways and without interaction. But the transcription levels of pmrA, pmrB, and eptA in the mutants were reduced to varying degrees compared with the wild type, indicating that the deletion of PhoP/PhoQ in Y. enterocolitica can also affect the transcription of the PmrA/PmrB system.
Discussion
Polymyxins have been employed as “last resort” antimicrobials (Han et al., 2018a). Gaining a better understanding of the mechanism of resistance to polymyxin is of critical importance. Currently, there are two main mechanisms to explain bacterial resistance to polymyxin: intrinsic resistance (adaptive resistance), which relies on LPS modifications, such as L-Ara4N and pEtN; and mcr genes induced resistance, which are encoded on plasmids and phages (Trimble et al., 2016; MacNair et al., 2018; Simpson and Trent, 2019). In this study, PhoP/PhoQ was demonstrated to be essential for the resistance of Y. enterocolitica to polymyxins (Figure 2). This was mainly because PhoP/PhoQ conferred a more resistant membrane for the binding of polymyxin by regulating LPS modification related to AMP resistance (Figures 4, 5). However, the LPS modification of Y. enterocolitica under PMB and PME was found to be different (Figure 5). In the presence of PMB, a variety of lipid-modified structures containing L-Ara4N were detected. However, when exposed to PME, in addition to containing L-Ara4N, the structures of palmitate and C16:1 were also observed (m/z at 1,823). Thus, when Y. enterocolitica was exposed to different AMPs, the LPS modification was not totally the same. And L-Ara4N was the main structure for Y. enterocolitica to set the adaptive resistance to polymyxin. Deletion of phoP and phoQ in Y. enterocolitica resulted the downregulation of the arnBCADTEF and papP which encode enzymes that responsible for L-Ara4N and palmitate (Figure 6). These results emphasized the role of PhoP/PhoQ in response to polymyxin in Y. enterocolitica. LPS modification also reduced the binding ability of polymyxin to Y. enterocolitica (Figure 4). This decrease may be due to a reduction in the negative charge and resistance to polymyxin induced by charge repulsion (Simpson and Trent, 2019). In addition, steric hindrance and lipid A tail packing produced by LPS modification could decrease the fluidity of the outer membrane, which may also prevent polymyxin from binding to Y. enterocolitica (Khondker et al., 2019; Simpson and Trent, 2019). Studies have shown that polymyxin exerts antimicrobial activity by targeting the bacterial cell membrane and gradually destroys the outer and inner membranes (Brogden, 2005). The destruction of the inner and outer membranes also increased when PhoP/PhoQ was absent (Figure 3; Supplementary Figure 2). Besides, study has reported that polymyxin B causes malfunction of respiration and severe consumption of ATP, leading to cell death and carbon starvation. And maintaining ATP level benefits the polymyxin resistance (Yu et al., 2019). Similarly, in Figures 3E,F, it is showed that the mutants of phoP and phoQ were sensitive to polymyxins and maintained lower intercellular ATP content compared to wild type.
In most Gram-negative bacteria, the TCSs, PhoP/PhoQ and PmrA/PmrB, mediate polymyxin resistance by governing the LPS modification pathway (Trimble et al., 2016; Simpson and Trent, 2019). However, there are some differences in the LPS modification mechanisms among different Gram-negative bacteria, as shown in Figure 1. For example, PhoP phosphorylated in Salmonella can activate PmrA/PmrB through the protein, PmrD (Hong et al., 2018). Although PmrD in E. coli cannot function in the same manner as Salmonella, it is required for the modification of lipid A in E. coli under low Mg2+ growth conditions (Rubin et al., 2015). In Klebsiella pneumoniae, the arn operon can not only be regulated directly by PhoP/PhoQ, but also indirectly through PmrD (Chen et al., 2021). In Yersinia pestis, despite the lack of PmrD, protein lipid A with L-Ara4N can be promoted by PhoP dependently and independently through different inducing signals (Winfield et al., 2005). Based on our results, the role of PhoP/PhoQ in intrinsic resistance mechanism of Y. enterocolitica to polymyxins summarized as shown in Figure 7. The modification of LPS with L-Ara4N and palmitate regulated by PhoP/PhoQ increase the resistance of Y. enterocolitica. It reduces the binding ability of polymyxin to cell membrane. For the cross-regulation between PhoP/PhoQ and PmrA/PmrB, although PmrD was absent in Y. enterocolitica, and such pEtN structure was not observed under PMB and PME (Figure 5), the connection between PhoP/PhoQ and PmrA/PmrB was also observed. By comparing the results of gene expression analysis, the transcription of pmrA, pmrB, and eptA in the phoP and phoQ mutants was downregulated (Figure 6). Besides, for Y. enterocolitica, the intrinsic resistance mechanism induced by PhoP/PhoQ showed difference compared with Salmonella, not only the absent of PmrD, but also other genes such as lpxT lacking a PhoP binding site (Hong et al., 2018). And lipid A containing L-Ara4N has still been detected in phoP mutants (Figure 5), these all indicates that the response to polymyxin is not independently regulated by PhoP/PhoQ. Some non-coding small RNAs may play an important role in regulation pathways in Y. enterocolitica (Simpson and Trent, 2019). But, detail mechanism of the intrinsic resistance needs further study.
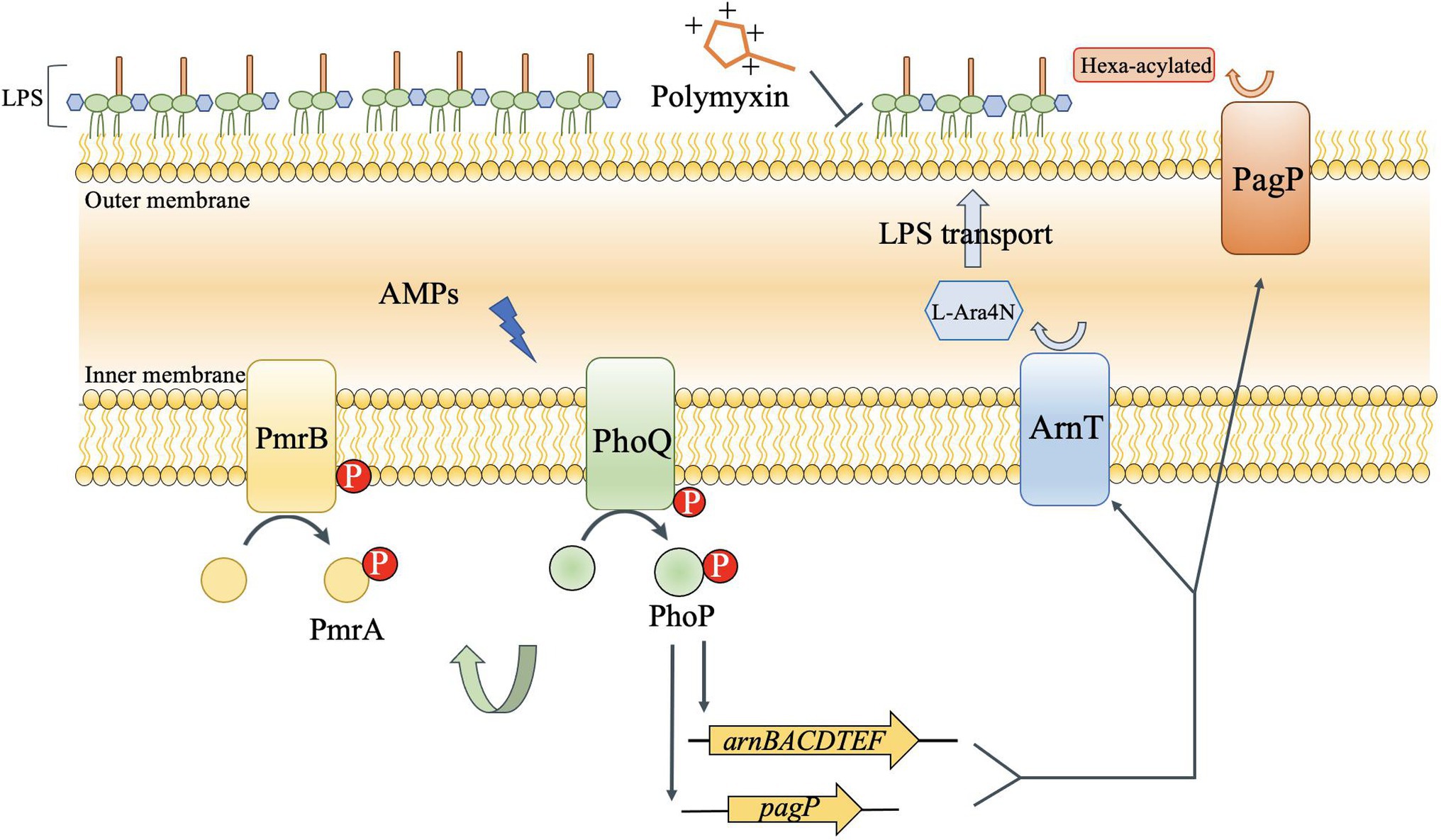
Figure 7. Regulation of LPS modification by the PhoP/PhoQ pathway of Y. enterocolitica to increase self-induced resistance to polymyxin. PhoP/PhoQ regulates genes that encode enzymes that alter acylation (PagP) and modify phosphates with aminoarabinose (arnT). The activity of PhoP can also affect the transcription of pmrA and pmrB. The increased modification of lipid A by ArnT and PagP alters the charge of the outer membrane and accumulates lipid tail packing to decrease the fluidity, which caused the resistance of Y. enterocolitica to AMP.
Although the regulation pathway of PhoP/PhoQ in the presence of polymyxin in Y. enterocolitica is not completely understood, we demonstrated the role of PhoP/PhoQ to increase the resistance of Y. enterocolitica to polymyxins. And we also raised some new tasks to find out the mechanism of the intrinsic resistance in Y. enterocolitica. Firstly, finding out the other potential regulatory factors involved in the connection between PhoP/PhoQ and PmrA/PmrB. Secondly, screening other regulatory factors involved in lipid A modifications which enhance the intrinsic resistance to polymyxins.
Conclusion
All in all, it is important to gain a better understanding of the intrinsic resistance toward polymyxin in Gram-negative bacteria. In this study, the role of PhoP/PhoQ in Y. enterocolitica intrinsic resistance to polymyxins was demonstrated. The existence of PhoP/PhoQ in Y. enterocolitica showed a higher resistance to polymyxins, which was mainly caused by the modification of LPS with palmitate and L-Ara4N, and regulated by PhoP/PhoQ, conferring a more resistant membrane for the binding of polymyxin. According to the transcription analysis, the PhoP/PhoQ of Y. enterocolitica not only regulated LPS modification genes, but also affected the transcription of PmrA/PmrB. These findings expand our understanding of the intrinsic resistance to polymyxin in Y. enterocolitica and expand current knowledge regarding the role of PhoP/PhoQ in Enterobacteriaceae.
Data Availability Statement
All datasets generated for this study are included in the article/Supplementary Material.
Author Contributions
HG performed the experiments under the guidance of JC, analyzed the experimental data, and drafted the manuscript. JC and HG developed the idea for the study and designed the research. TZ and CH made substantial contributions to conception, interpretation of data and revised the manuscript. All authors have read and approved the final manuscript.
Funding
This work was supported by the Beijing Natural Science Foundation (6202016) and the National Natural Science Foundation of China (31671830).
Conflict of Interest
The authors declare that the research was conducted in the absence of any commercial or financial relationships that could be construed as a potential conflict of interest.
Publisher’s Note
All claims expressed in this article are solely those of the authors and do not necessarily represent those of their affiliated organizations, or those of the publisher, the editors and the reviewers. Any product that may be evaluated in this article, or claim that may be made by its manufacturer, is not guaranteed or endorsed by the publisher.
Supplementary Material
The Supplementary Material for this article can be found online at: https://www.frontiersin.org/articles/10.3389/fmicb.2022.758571/full#supplementary-material
References
Akhoundsadegh, N., Belanger, C. R., and Hancock, R. E. W. (2019). Outer membrane interaction kinetics of new polymyxin B analogs in Gram-negative bacilli. Antimicrob. Agents Chemother. 63, 1–13. doi: 10.1128/AAC.00935-19
Bader, M. W., Sanowar, S., Daley, M. E., Schneider, A. R., Cho, U., Xu, W., et al. (2005). Recognition of antimicrobial peptides by a bacterial sensor kinase. Cell 122, 461–472. doi: 10.1016/j.cell.2005.05.030
Bader, J., and Teuber, M. (1973). Binding to the O-antigenic lipopolysaccharide of Salmonella typhimurium. Z. Naturforsch. 28, 422–430.
Brogden, K. A. (2005). Antimicrobial peptides: pore formers or metabolic inhibitors in bacteria? Nat. Rev. Microbiol. 3, 238–250. doi: 10.1038/nrmicro1098
Chen, A. I., Albicoro, F. J., Zhu, J., and Goulian, M. (2021). Effects of regulatory network organization and environment on PmrD connector activity and polymyxin resistance in Klebsiella pneumoniae and Escherichia coli. Antimicrob. Agents Chemother. 65, e00889–20. doi: 10.1128/AAC.00889-20
Clementi, E. A., Marks, L. R., Roche-Håkansson, H., and Håkansson, A. P. (2014). Monitoring changes in membrane polarity, membrane integrity, and intracellular ion concentrations in Streptococcus pneumoniae using fluorescent dyes. J. Vis. Exp. 84, 1–8. doi: 10.3791/51008
CLSI (2017). M100 Performance Standards for Antimicrobial Susceptibility Testing. 27th Edn. Clinical and Laboratory Standards Institute.
Cullen, T. W., Giles, D. K., Wolf, L. N., Ecobichon, C., Boneca, I. G., and Trent, M. S. (2011). Helicobacter pylori versus the host: remodeling of the bacterial outer membrane is required for survival in the gastric mucosa. PLoS Pathog. 7:e1002454. doi: 10.1371/journal.ppat.1002454
Duperthuy, M. (2020). Antimicrobial peptides: virulence and resistance modulation in Gram-negative bacteria. Microorganisms 8:280. doi: 10.3390/microorganisms8020280
Han, M. L., Velkov, T., Zhu, Y., Roberts, K. D., Le Brun, A. P., Chow, S. H., et al. (2018a). Polymyxin-induced lipid A deacylation in Pseudomonas aeruginosa perturbs polymyxin penetration and confers high-level resistance. ACS Chem. Biol. 13, 121–130. doi: 10.1021/acschembio.7b00836
Han, M. L., Zhu, Y., Creek, D. J., Lin, Y. W., Anderson, D., Shen, H. H., et al. (2018b). Alterations of metabolic and lipid profiles in polymyxin-resistant Pseudomonas aeruginosa. Antimicrob. Agents Chemother. 62, 1–14. doi: 10.1128/AAC.02656-17
Hankins, J. V., Madsen, J. A., Needham, B. D., Brodbelt, J. S., and Trent, M. S. (2013). The outer membrane of Gram-negative bacteria: lipid A isolation and characterization. Methods Mol. Biol. 966, 239–258. doi: 10.1007/978-1-62703-245-2_15
Henderson, J. C., O’Brien, J. P., Brodbelt, J. S., and Trent, M. S. (2013). Isolation and chemical characterization of lipid A from Gram-negative bacteria. J. Vis. Exp. 79, 1–11. doi: 10.3791/50623
Hoch, J. A. (2000). Two-component and phosphorelay signal transduction. Curr. Opin. Microbiol. 3, 165–170. doi: 10.1016/S1369-5274(00)00070-9
Hong, X., Chen, H. D., and Groisman, E. A. (2018). Gene expression kinetics governs stimulus-specific decoration of the Salmonella outer membrane. Sci. Signal. 11, 1–12. doi: 10.1126/scisignal.aar7921
Khondker, A., Dhaliwal, A. K., Saem, S., Mahmood, A., Fradin, C., Moran-Mirabal, J., et al. (2019). Membrane charge and lipid packing determine polymyxin-induced membrane damage. Commun. Biol. 2, 1–11. doi: 10.1038/s42003-019-0297-6
Lee, D. S., and Je, J. Y. (2013). Gallic acid- grafted -chitosan inhibits foodborne pathogens by a membrane damage mechanism. J. Agric. Food Chem. 61, 6574–6579. doi: 10.1021/jf401254g
Lin, Z., Cai, X., Chen, M., Ye, L., Wu, Y., Wang, X., et al. (2018). Virulence and stress responses of Shigella flexneri regulated by PhoP/PhoQ. Front. Microbiol. 8:2689. doi: 10.3389/fmicb.2017.02689
MacNair, C. R., Stokes, J. M., Carfrae, L. A., Fiebig-Comyn, A. A., Coombes, B. K., Mulvey, M. R., et al. (2018). Overcoming mcr-1 mediated colistin resistance with colistin in combination with other antibiotics. Nat. Commun. 9:458. doi: 10.1038/s41467-018-02875-z
Meng, J., Huang, C., Huang, X., Liu, D., Han, B., and Chen, J. (2020). Osmoregulated periplasmic glucans transmit external signals through Rcs phosphorelay pathway in Yersinia enterocolitica. Front. Microbiol. 11:122. doi: 10.3389/fmicb.2020.00122
Minagawa, S., Ogasawara, H., Kato, A., Yamamoto, K., Eguchi, Y., Oshima, T., et al. (2003). Identification and molecular characterization of the Mg2+ stimulon of Escherichia coli. J. Bacteriol. 185, 3696–3702. doi: 10.1128/JB.185.13.3696-3702.2003
Mitchell, A. M., and Silhavy, T. J. (2019). Envelope stress responses: balancing damage repair and toxicity. Nat. Rev. Microbiol. 17, 417–428. doi: 10.1038/s41579-019-0199-0
Prost, L. R., Daley, M. E., Le Sage, V., Bader, M. W., Le Moual, H., Klevit, R. E., et al. (2007). Activation of the bacterial sensor kinase PhoQ by acidic pH. Mol. Cell 26, 165–174. doi: 10.1016/j.molcel.2007.03.008
Rahnamaeian, M. (2011). Antimicrobial peptides: modes of mechanism, modulation of defense responses. Plant Signal. Behav. 6, 1325–1332. doi: 10.4161/psb.6.9.16319
Reinés, M., Llobet, E., Dahlström, K. M., Pérez-Gutiérrez, C., Llompart, C. M., Torrecabota, N., et al. (2012a). Deciphering the acylation pattern of Yersinia enterocolitica lipid A. PLoS Pathog. 8:e1002978. doi: 10.1371/journal.ppat.1002978
Reinés, M., Llobet, E., Llompart, C. M., Moranta, D., Pérez-Gutiérrez, C., and Bengoechea, J. A. (2012b). Molecular basis of Yersinia enterocolitica temperature-dependent resistance to antimicrobial peptides. J. Bacteriol. 194, 3173–3188. doi: 10.1128/JB.00308-12
Rubin, E. J., Herrera, C. M., Crofts, A. A., and Trent, M. S. (2015). PmrD is required for modifications to Escherichia coli endotoxin that promote antimicrobial resistance. Antimicrob. Agents Chemother. 59, 2051–2061. doi: 10.1128/AAC.05052-14
Santos, D. E. S., Pol-Fachin, L., Lins, R. D., and Soares, T. A. (2017). Polymyxin binding to the bacterial outer membrane reveals cation displacement and increasing membrane curvature in susceptible but not in resistant lipopolysaccharide chemotypes. J. Chem. Inf. Model. 57, 2181–2193. doi: 10.1021/acs.jcim.7b00271
Schäfer, A., Tauch, A., Jäger, W., Kalinowski, J., Thierbach, G., and Pühler, A. (1994). Small mobilizable multi-purpose cloning vectors derived from the Escherichia coli plasmids pK18 and pK19: selection of defined deletions in the chromosome of Corynebacterium glutamicum. Gene 145, 69–73. doi: 10.1016/0378-1119(94)90324-7
Schindler, P. R. G., and Teuber, M. (1975). Action of polymyxin B on bacterial membranes: morphological changes in the cytoplasm and in the outer membrane of Salmonella typhimurium and Escherichia coli B. Antimicrob. Agents Chemother. 8, 95–104. doi: 10.1128/AAC.8.1.95
Shoaib, M., Shehzad, A., Raza, H., Niazi, S., Khan, I. M., Akhtar, W., et al. (2019). A comprehensive review on the prevalence, pathogenesis and detection of Yersinia enterocolitica. RSC Adv. 9, 41010–41021. doi: 10.1039/c9ra06988g
Shprung, T., Peleg, A., Rosenfeld, Y., Trieu-Cuot, P., and Shai, Y. (2012). Effect of PhoP-PhoQ activation by broad repertoire of antimicrobial peptides on bacterial resistance. J. Biol. Chem. 287, 4544–4551. doi: 10.1074/jbc.M111.278523
Simpson, B. W., and Trent, M. S. (2019). Pushing the envelope: LPS modifications and their consequences. Nat. Rev. Microbiol. 17, 403–416. doi: 10.1038/s41579-019-0201-x
Trimble, M. J., Mlynárčik, P., Kolář, M., and Hancock, R. E. W. (2016). Polymyxin: alternative mechanisms of action and resistance. Cold Spring Harb. Perspect. Med. 6:a025288. doi: 10.1101/cshperspect.a025288
Véscovi, E. G., Ayala, Y. M., Di Cera, E., and Groisman, E. A. (1997). Characterization of the bacterial sensor protein PhoQ: evidence for distinct binding sites for Mg2+ and Ca2+. J. Biol. Chem. 272, 1440–1443. doi: 10.1074/jbc.272.3.1440
West, A. H., and Stock, A. M. (2001). Histidine kinases and response regulator proteins in two-component signaling systems. Trends Biochem. Sci. 26, 369–376. doi: 10.1016/S0968-0004(01)01852-7
Winfield, M. D., Latifi, T., and Groisman, E. A. (2005). Transcriptional regulation of the 4-amino-4-deoxy-L-arabinose biosynthetic genes in Yersinia pestis. J. Biol. Chem. 280, 14765–14772. doi: 10.1074/jbc.M413900200
Yu, W., Yu, W., Pan, Q., and Ye, B. (2019). Glucose-induced cyclic lipopeptides resistance in bacteria via ATP maintenance through enhanced glycolysis glucose-induced cyclic lipopeptides resistance in bacteria via ATP maintenance through enhanced glycolysis. iScience 21, 135–144. doi: 10.1016/j.isci.2019.10.009
Keywords: Yersinia enterocolitica, PhoP/PhoQ, LPS modification, polymyxin, intrinsic resistance
Citation: Guo H, Zhao T, Huang C and Chen J (2022) The Role of the Two-Component System PhoP/PhoQ in Intrinsic Resistance of Yersinia enterocolitica to Polymyxin. Front. Microbiol. 13:758571. doi: 10.3389/fmicb.2022.758571
Edited by:
Dongsheng Zhou, Beijing Institute of Microbiology and Epidemiology, ChinaReviewed by:
Paola Sperandeo, University of Milan, ItalyAlberto Sola, Instituto de Biotecnología de León, Spain
Copyright © 2022 Guo, Zhao, Huang and Chen. This is an open-access article distributed under the terms of the Creative Commons Attribution License (CC BY). The use, distribution or reproduction in other forums is permitted, provided the original author(s) and the copyright owner(s) are credited and that the original publication in this journal is cited, in accordance with accepted academic practice. No use, distribution or reproduction is permitted which does not comply with these terms.
*Correspondence: Jingyu Chen, chenjy@cau.edu.cn