- 1Centre de Recherche et de Développement de Saint-Jean-sur-Richelieu, Agriculture et Agroalimentaire Canada, Saint-Jean-sur-Richelieu, QC, Canada
- 2Bioinformatics and Systems Biology, Justus Liebig University Giessen, Giessen, Germany
Lettuce is a major vegetable crop worldwide that is affected by numerous bacterial pathogens, including Xanthomonas hortorum pv. vitians, Pseudomonas cichorii, and Pectobacterium carotovorum. Control methods are scarce and not always effective. To develop new and sustainable approaches to contain these pathogens, we screened more than 1,200 plant-associated Pseudomonas strains retrieved from agricultural soils for their in vitro antagonistic capabilities against the three bacterial pathogens under study. Thirty-five Pseudomonas strains significantly inhibited some or all three pathogens. Their genomes were fully sequenced and annotated. These strains belong to the P. fluorescens and P. putida phylogenomic groups and are distributed in at least 27 species, including 15 validly described species. They harbor numerous genes and clusters of genes known to be involved in plant-bacteria interactions, microbial competition, and biocontrol. Strains in the P. putida group displayed on average better inhibition abilities than strains in the P. fluorescens group. They carry genes and biosynthetic clusters mostly absent in the latter strains that are involved in the production of secondary metabolites such as 7-hydroxytropolone, putisolvins, pyochelin, and xantholysin-like and pseudomonine-like compounds. The presence of genes involved in the biosynthesis of type VI secretion systems, tailocins, and hydrogen cyanide also positively correlated with the strains’ overall inhibition abilities observed against the three pathogens. These results show promise for the development of biocontrol products against lettuce bacterial pathogens, provide insights on some of the potential biocontrol mechanisms involved, and contribute to public Pseudomonas genome databases, including quality genome sequences on some poorly represented species.
1 Introduction
Lettuce (Lactuca sativa, Asteraceae) is a widely consumed vegetable worldwide that generated in 2018 a world gross production value of 14.3 billion USD, when combined with chicory (Food and Agriculture Organization of the United Nations, 2021). However, production is affected by several bacterial and fungal pathogens in most growing regions of the world, often resulting in important yield and economic losses (Subbarao et al., 2017). Some of the main bacterial pathogens of lettuce include Xanthomonas hortorum pv. vitians (Hébert et al., 2021), formerly known as X. campestris pv. vitians (Vauterin et al., 1995; Morinière et al., 2020), Pseudomonas cichorii (Pauwelyn et al., 2011), and Pectobacterium carotovorum (Cariddi and Sanzani, 2013). These three bacteria directly affect lettuce aboveground tissues, especially leaves.
X. hortorum pv vitians is a hemibiotrophic organism mainly infecting plant species belonging to the Lactuca genus, especially lettuce, on which it induces bacterial leaf spot (Toussaint et al., 2012; Morinière et al., 2022). This disease is characterized by the development of water-soaked and geometric lesions on leaves (Subbarao et al., 2017; Hébert et al., 2021). These lesions can expand and coalesce, leading to the necrosis of entire leaves. Worldwide, this pathogen has been responsible for several outbreaks on lettuce over the past decade and is considered re-emergent (Subbarao et al., 2017; Morinière et al., 2022). Infection is T3SS-dependent and relies on the secretion of cell wall degrading-enzymes, the use of type VI secretion systems (T6SS) and their effectors, as well as other determinants (Büttner and Bonas, 2010; Timilsina et al., 2020). X. hortorum pv vitians penetrates the leaves through natural openings, mainly the stomata and the hydrathodes, and through wounds. The pathogen then colonizes the mesophyll, before reaching the vascular system (Dia et al., 2022).
P. cichorii, another hemibiotrophic pathogen, infects various hosts and causes varnish spot on lettuce (also called midrib rot) (Hikichi et al., 2013). This disease consists in the development of firm dark-brown necrotic lesions on inner leaves of head-forming lettuce cultivars, sometimes along veins and midribs (Subbarao et al., 2017). P. cichorii produces numerous phytotoxic compounds to infect the plant, such as lipopeptides, and sometimes uses a type III secretion system (T3SS) as well, leading to plant cell death (Hikichi et al., 2013; Huang et al., 2015). It enters the leaves through the stomata, develops into intercellular spaces of the leaf epidermis and the mesophyll, until reaching the vascular system, in a similar way to X. hortorum pv vitians (Hikichi et al., 2013).
P. carotovorum is a necrotrophic pathogen able to infect a broad range of plant species, including lettuce, on which it causes bacterial soft rot (Cariddi and Sanzani, 2013; Davidsson et al., 2013). This disease is characterized by the wilting of the outer leaves and a macerated gelatinous greenish pith, eventually leading to the rotting of the entire lettuce plant (Subbarao et al., 2017). Pathogenicity determinants of P. carotovorum include a wide array of plant cell wall-degrading enzymes, along with some toxins (Davidsson et al., 2013). The pathogen infects its hosts primarily through wounds (Agrios, 2005).
The current strategies to control these pathogens are often ineffective and rely primarily on preventive methods, as few curative options are available, if any (Pauwelyn et al., 2011; Cariddi and Sanzani, 2013; Subbarao et al., 2017; Hébert et al., 2021). Prophylactic methods include the use of crop rotation, less susceptible cultivars, seed and equipment cleaning, well-drained soils, adequate spacing between the plants to improve air movement and reduce leaf wetness, and drip irrigation instead of sprinkler irrigation (Agrios, 2005; Subbarao et al., 2017). Copper-based compounds have been used to try to control lettuce bacterial diseases such as bacterial leaf spot (Dia et al., 2022). However, these compounds sometimes cause phytotoxicity and their widespread use favors resistance emergence, leading to restricted usage (Carisse et al., 2000; Fatmi and Bolkan, 2017; Dia et al., 2022). A fungicide containing potassium salts of phosphorous acid is also used to control bacterial pathogens on lettuce (Hébert et al., 2021). It favors resistance development in bacterial populations as well. To decrease the impacts of bacterial pathogens on lettuce, more efficient, sustainable, and environmentally friendly methods need to be developed and implemented.
Biocontrol could become the cornerstone of these new strategies. It consists in using living agents to control detrimental organisms in order to provide human benefits (Stenberg, 2021). In plant pathology, it especially pertains to the direct or indirect inhibition of a pathogen, or the symptoms it causes, by another organism or a consortia of organisms (Cook and Baker, 1983). Such inhibition can be mediated by antibiosis, hyperparasitism, the induction of plant defenses, and competition for resources (Collinge et al., 2022). Biocontrol offers several advantages compared to compound-based pesticides, such as reduced risks of soil contamination by harmful residues, various modes of action limiting the emergence of resistance in pathogens, and a potentially better social acceptability (Collinge et al., 2022). Some bacteria living in the rhizosphere of plants have been described as effective biocontrol agents. They are often called plant growth-promoting rhizobacteria (PGPR), alongside rhizobacteria that directly improve plant growth by enhancing plant nutrition or interfering with plant hormone signaling (Lugtenberg and Kamilova, 2009).
Pseudomonas is amongst the most studied PGPR-containing genera (Weller, 2007; Höfte, 2021). It belongs to the recently renamed Pseudomonadota phylum, previously called Proteobacteria (Oren and Garrity, 2021). The Pseudomonas genus includes bacteria that are rod-shaped, motile, Gram-negative, mostly aerobic, and distributed across many habitats and hosts (Palleroni, 1984). Many Pseudomonas strains displaying biocontrol abilities against a myriad of bacterial, fungal, and oomycete plant pathogens have been identified over the past decades, especially from agricultural soils (Weller, 2007; Biessy et al., 2019, 2021; Balthazar et al., 2021). A multitude of them belong to the P. fluorescens phylogenomic group, and to a lesser extent, to the P. putida group (Weller, 2007; Berendsen et al., 2015; Planchamp et al., 2015; Biessy et al., 2019; Höfte, 2021). They are able to produce diverse biocontrol-related secondary metabolites, such as antibiotics, lipopeptides, and siderophores, as well as molecules involved in plant-bacteria interactions, including hormones and effectors (Gross and Loper, 2009; Ghequire and De Mot, 2014; Götze and Stallforth, 2020; Zboralski and Filion, 2020; Zboralski et al., 2022). Only few Pseudomonas strains have been identified as effective biocontrol agents against lettuce pathogens. To our knowledge, these have mostly been assessed against fungal pathogens, not bacterial ones (Grosch et al., 2005; Sottero et al., 2006; Adesina et al., 2007; Scherwinski et al., 2008; Schreiter et al., 2018; Aggeli et al., 2020).
To address this issue and to contribute to the development of sustainable biocontrol solutions against lettuce bacterial pathogens, we collected more than 1,200 Pseudomonas strains from agricultural soils in the province of Québec (Canada). We identified 35 strains displaying promising biocontrol abilities against X. hortorum pv. vitians, P. cichorii, and/or P. carotovorum. The genomes of these strains were sequenced and analyzed to identify biosynthetic genes known to be involved in the production of secondary metabolites of interest in biocontrol.
2 Materials and methods
2.1 Bacterial strains isolation
All Pseudomonas strains used in this study were isolated in 2019 from agricultural soils collected from 29 polyculture organic farms located in the Montérégie region in Québec, Canada. Soil samples were collected close to the roots of plants belonging to various vegetable species and stored at 4°C. One gram of each soil sample was diluted into 100 ml of a 0.9% NaCl solution and the resulting suspension was shaken for 10 min at 250 rpm. Serial dilutions of this suspension were spread on King’s B agar medium (King et al., 1954) amended with 100 μg.ml–1 cycloheximide, 40 μg.ml–1 ampicillin, and 13 μg.ml–1 chloramphenicol to select for Pseudomonas strains (McSpadden Gardener et al., 2001). Plates were incubated for 48 h at 25°C. Single colonies were retrieved and successively streaked several times on regular King’s B agar medium to ensure purity. The strains were cryopreserved at –80°C in tryptic soy broth with 10% glycerol until further use.
2.2 In vitro confrontational assays against bacterial lettuce pathogens
The inhibition capabilities of all isolates were individually assessed in vitro against each lettuce bacterial pathogen, namely X. hortorum pv. vitians B07-007, P. cichorii B12-019, and P. carotovorum B12-025. These strains were isolated from infected lettuce leaves in Southern Quebec, Canada (Affia, 2016; Hébert et al., 2021). Each pathogenic bacterium was initially grown for 48 h at 22.5°C on yeast dextrose carbonate agar medium for X. hortorum pv. vitians and on King’s B agar medium for the other two pathogens. The bacteria were then resuspended from the plate into sterile distilled water, to a final concentration of 108 CFU.ml–1. These suspensions of bacterial pathogens were used in the following assays.
A screening of all Pseudomonas isolates was performed using a modified perpendicular streaking method (Gislin et al., 2018). Pseudomonas strains were grown in King’s B broth for 24 h at 125 rpm and 22.5°C before being directly streaked using an inoculation loop at the bottom of Petri plates containing tryptic soy agar (TSA) medium. After 48 h, a suspension volume of 10 μl of each of the three bacterial pathogens adjusted to 108 CFU.ml–1, prepared as described above, was applied on the top of each Petri plate. The Petri dish was immediately inclined to allow the three suspensions of pathogens to reach the streaked Pseudomonas strain. Plates were incubated for 5 days at 25°C and were subsequently evaluated for any antagonistic effect.
Pseudomonas isolates that were found to have an antagonistic activity against at least one pathogen were further used. Their antagonistic effect was quantitatively assessed using an overlay method. This method consisted in applying the antagonistic Pseudomonas strains directly onto the pathogenic bacteria already spread all over the plate. Briefly, a volume of 100 μl of each bacterial pathogen suspension was individually spread onto TSA medium to fully cover the Petri’s surface and left to dry for 2 h. Each antagonistic Pseudomonas strain was grown for 24 h on King’s B agar medium and resuspended in distilled water to a final concentration of 108 CFU.ml–1. Twenty microliters were then added to the center of the plate. After 6 days at 25°C, the diameter of the entire inhibition zone was measured, namely the circular area where the pathogen was not able to grow. Three plates were prepared for each pathogen-Pseudomonas isolate combination.
2.3 Genome sequencing, assembly, and annotation
Isolates displaying any inhibitory activity against at least one of the three bacterial pathogens were selected for whole-genome sequencing. Their DNA was extracted from bacterial cells grown in King’s B agar medium for 48 h at 25°C using the DNeasy UltraClean microbial kit (Qiagen, Toronto, ON, Canada) following a modified version of the manufacturer’s instructions. An additional step was performed just before mechanical lysis of the samples to improve the yield: 10 μl of proteinase K (Bioshop Canada, Burlington, ON, Canada) were added to each sample and the samples were then heated 10 min at 70°C before proceeding with the manufacturer’s instructions. The preparation of the genomic DNA libraries as well as the sequencing were carried out at the Integrated Microbiome Resource (Halifax, NS, Canada). Genomic DNA was mechanically sheared to obtain 9–10 kb fragments using Covaris g-TUBE (Covaris, CA). Libraries were prepared using the PacBio SMRTbell Express Template Prep kit (Pacific Biosciences, Menlo Park, CA). The sequencing was accomplished with a PacBio Sequel sequencer (v3 chemistry). The genomes were assembled using the long-read assembler Flye v2.8.1 (Kolmogorov et al., 2019). Circular contigs were rotated using Geneious Prime 2022.1.1 (Biomatters, Auckland, New Zealand). Annotation was carried out by the NCBI Prokaryotic Genome Annotation Pipeline v5.3 (Tatusova et al., 2016).
2.4 Species-level identification
Species-level identification of the strains under study was performed using the Type (Strain) Genome Server (Meier-Kolthoff and Göker, 2019; Meier-Kolthoff et al., 2022). This web server provides digital DNA-DNA hybridization (dDDH) values between a query genome and a set of closely related type strain genomes. dDDH values were calculated using the Genome BLAST Distance Phylogeny (GBDP) formula d4 (Meier-Kolthoff et al., 2013). When the dDDH value between a strain and a type strain was slightly below the 70% threshold, the JSpeciesWS online tool (Richter et al., 2016) was used to calculate additional overall genome relatedness indexes (OGRIs), such as ANIb and TETRA.
2.5 Phylogenomic analyses
The web-server EDGAR 3.0 was used for ortholog calculation and phylogenomic analyses (Blom et al., 2009; Dieckmann et al., 2021). Orthologs were identified using BLAST bidirectional best hits with an orthology criterion corresponding to a score ratio value of 0.3. A phylogenomic tree was built out of 2,215 core genes per genome (79,740 in total) for a total of 2,163,293 bp per genome. The coding nucleotide sequences of the orthologous gene sets found in all genomes were individually aligned using MUSCLE (Edgar, 2004). The resulting alignments were concatenated into one that was used to generate the phylogenomic tree with the neighbor-joining method implemented in PHYLIP (Felsenstein, 2005). To verify the resulting tree topology, a bootstrapping was performed with 500 iterations. All branches within the tree showed 100% bootstrap support.
2.6 Identification of genes and BGCs involved in the production of phytobeneficial secondary metabolites
Genes and biosynthetic gene clusters involved in the production of secondary metabolites related to biocontrol, plant-growth promotion and rhizocompetence were searched for in all 35 genomes. Sequences of genes and biosynthetic gene clusters (BGCs) of interest were retrieved from GenBank and from the Pseudomonas genome database (Winsor et al., 2016). These sequences (Supplementary Table 1) were used as baits in BLAST searches to identify numerous genes and/or BGCs in the genomes of the strains under study. Putative homologs were identified using a cutoff of 70% sequence identity over 70% of the sequence length. Additional secondary metabolite BGCs were also identified using antiSMASH v6.0 (Blin et al., 2021). Antibacterial proteins (except for type VI effectors) were identified by a proteome-wide analysis of Pfam domains. This analysis was conducted using the built-in Pfam domain search v1.2 in CLC Genomics Workbench v21.0.5 (Qiagen, Aarhus, Denmark) and the Pfam-A v35.0 database (Mistry et al., 2021). Proteins harboring Pfam domains of interest were subsequently retrieved and subjected to further investigation with InterProScan v2.0 (Quevillon et al., 2005) in Geneious Prime 2022.1.1 (Biomatters, Auckland, New Zealand). Putative type VI effectors were identified in a two-step process. Firstly, genes encoding T6SS components, such as VgrG (PF04717/PF05954), Hcp (PF05638), PAAR domain-containing (PF05488) and TssB (PF05591) proteins, as well as type VI adaptor proteins (PF08786, PF09937, and PF13503), were mapped on the genomes of the strains under study. Then, potential type VI effectors encoded in the vicinity of these type VI-related genes were retrieved, analyzed with InterProScan, and compared to a collection of already characterized type VI effectors.
2.7 Statistical analyses
Data from confrontational assays was analyzed using R v3.6.1 with RStudio v1.2.5001 (R Studio Team, 2019; R Core Team, 2021). Multiple comparisons were performed through the “kruskal” function from the “agricolae” R package v1.3.1, involving Fisher’s least significant difference procedure with a Benjamini-Hochberg correction to adjust the p-values (de Mendiburu, 2017). Associations between genomic data and inhibition levels were determined using the Wilcoxon-Mann-Whitney test by comparing two groups, each containing at least 5 strains (Zboralski et al., 2020; Biessy et al., 2021). The level of significance was set at 0.05 for p-values, unless stated otherwise.
3 Results
3.1 Thirty-five Pseudomonas strains displaying biocontrol potential were isolated
A total of 1,210 Pseudomonas strains were retrieved from agricultural soil samples and screened for in vitro antagonistic abilities against X. hortorum pv. vitians, P. cichorii, and P. carotovorum. The streaking technique was found to be an effective screening method that allowed to single out 35 Pseudomonas strains able to inhibit at least one of the lettuce bacterial pathogens under study (data not shown). The overlay method provided quantitative inhibition levels for these strains against the selected lettuce pathogenic strains (Figure 1). All strains were able to inhibit X. hortorum pv. vitians to some extent, while some strains displayed no inhibition against P. cichorii or P. carotovorum. Strains with low- and high-inhibition abilities were divided into two statistically distinct groups, and a third group included strains with intermediate inhibition abilities, which were not necessarily different from the abilities of the other two groups (Figure 1). Some strains belonged to the high-inhibition group for all three pathogens: B21-009, B21-012, B21-023, B21-032, B21-035, B21-036, and B21-042. Similarly, others belonged to the low-inhibition group for all three pathogens: B21-017, B21-021, and B21-050. Some strains were completely unable to generate an inhibition zone against P. carotovorum and P. cichorii, while generating one against X. hortorum pv. vitians: B21-021, B21-039, and B21-063.
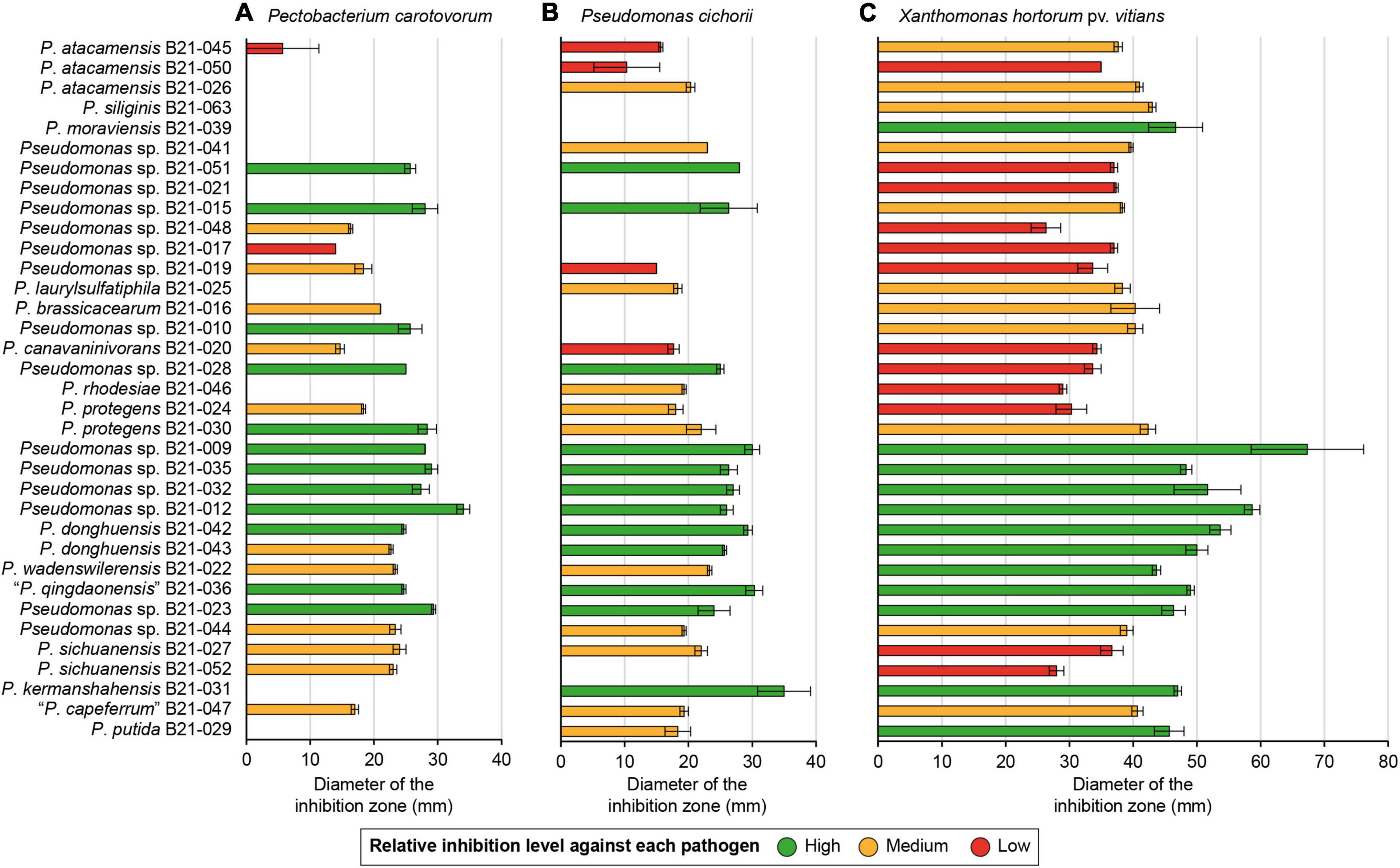
Figure 1. In vitro inhibition abilities of 35 Pseudomonas strains inhibiting P. carotovorum (A), P. cichorii (B), and/or X. hortorum pv. vitians (C). The colored inhibition levels against a given pathogen are significantly distinct from each other regarding the “High” and “Low” group (p < 0.001, Fisher’s least significant difference procedure). The “Medium” group strains do not necessarily display any significant difference in terms of inhibitory activity with strains from the other two groups. Strains are sorted according to their phylogenetic relationships. Error bars: standard error.
3.2 Sequencing reveals diverse Pseudomonas species among the inhibitory strains
The genomes of the 35 strains were sequenced on a PacBio Sequel sequencer, yielding an average of 286,069 reads with an average length of 5,333 bp (Supplementary Table 2). The long-read assembler Flye managed to completely assemble each of the 35 genomes into a single circular chromosome. Genome size ranges from 5.58 to 7.07 Mb (average 6.14 Mb) and the GC content varies from 58.5 to 64.3% (average 61.5%). Details on all sequenced genomes are provided in Supplementary Tables 2, 3. The GenBank accession numbers are displayed in Table 1.
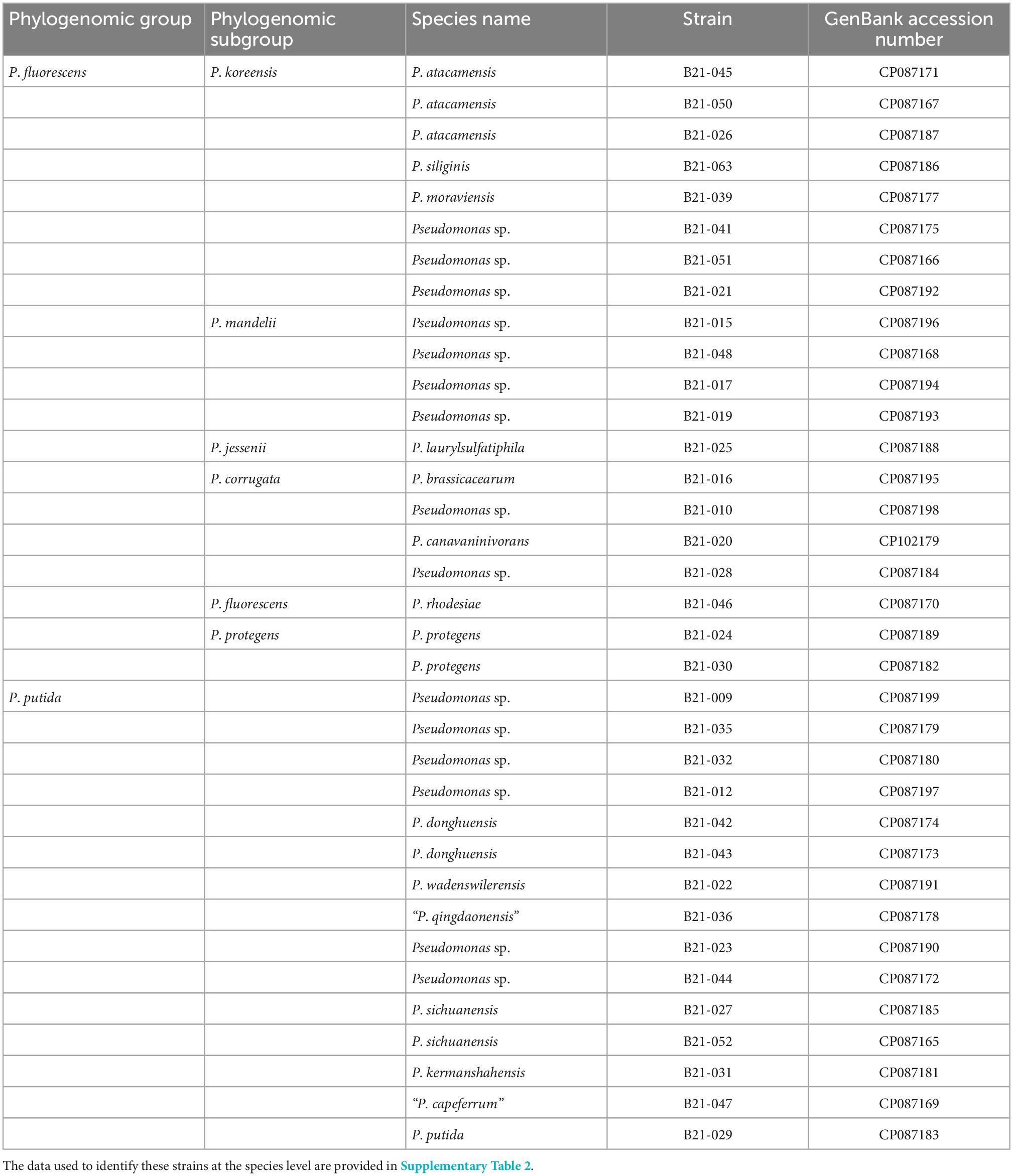
Table 1. List of the 35 sequenced Pseudomonas strains used in this study, according to their phylogenomic groups and subgroups.
The 35 strains fall into two distinct phylogenomic groups within the genus Pseudomonas: the P. fluorescens (20 strains) and P. putida (15 strains) groups (Supplementary Figure 1). Within the P. fluorescens group, the 20 strains are distributed in six distinct subgroups: the P. koreensis, P. mandelii, P. jessenii, P. corrugata, P. fluorescens, and P. protegens subgroups (Figure 2 and Supplementary Figure 1). The Type (Strain) Genome Server enabled us to assign 20 strains to 15 distinct species (Supplementary Figure 1 and Supplementary Table 2). The 15 remaining strains do not belong to any species described to date for which a genome is publicly available. They potentially represent 12 new species (Supplementary Figures 1, 2).
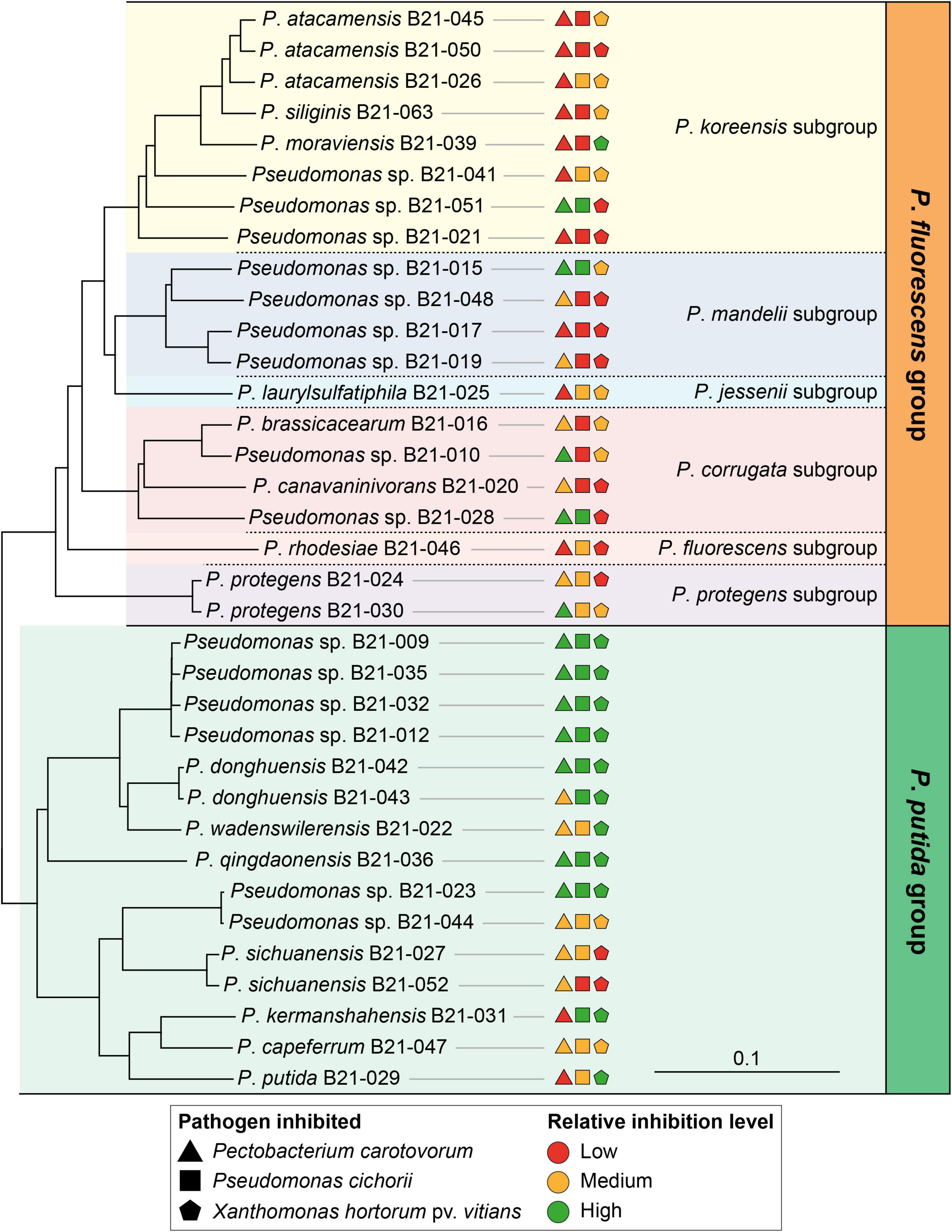
Figure 2. Neighbor-joining phylogeny of the 35 isolated Pseudomonas strains and their relative inhibition levels against various lettuce bacterial pathogens. This phylogenomic tree was generated with EDGAR from a concatenated alignment of 2,215 shared genes per genome. P. aeruginosa DSM 50071T was used as an outgroup (not shown on the tree). The colored inhibition levels refer to the levels presented in Figure 1. All branches in the tree show 100% bootstrap support (500 iterations).
3.3 The genomes contain genes and clusters involved in biocontrol and other plant growth-promoting traits
The 35 strains under study display an important genomic diversity, as evidenced by the size of the pangenome, which reaches 19,863 protein-coding genes. The strains share only 2,364 orthologous coding sequences (CDSs), which account for about 37–47% of the total number of CDSs found in each genome (Supplementary Table 3). In addition, each strain harbors, on average, 124 singletons (Supplementary Table 3). Genes and BGCs involved in the production of secondary metabolites related to biocontrol, plant-growth promotion and rhizocompetence were searched in the 35 genomes under study to determine if this genomic diversity includes a diversity of biocontrol-associated traits. Various genes and/or gene clusters of interest, known to be involved in the biosynthesis of antibiotics, antimicrobial lipopeptides and proteins, siderophores, secretion system apparatuses, and plant hormone-related molecules were uncovered (Figure 3 and Supplementary Tables 4, 5).
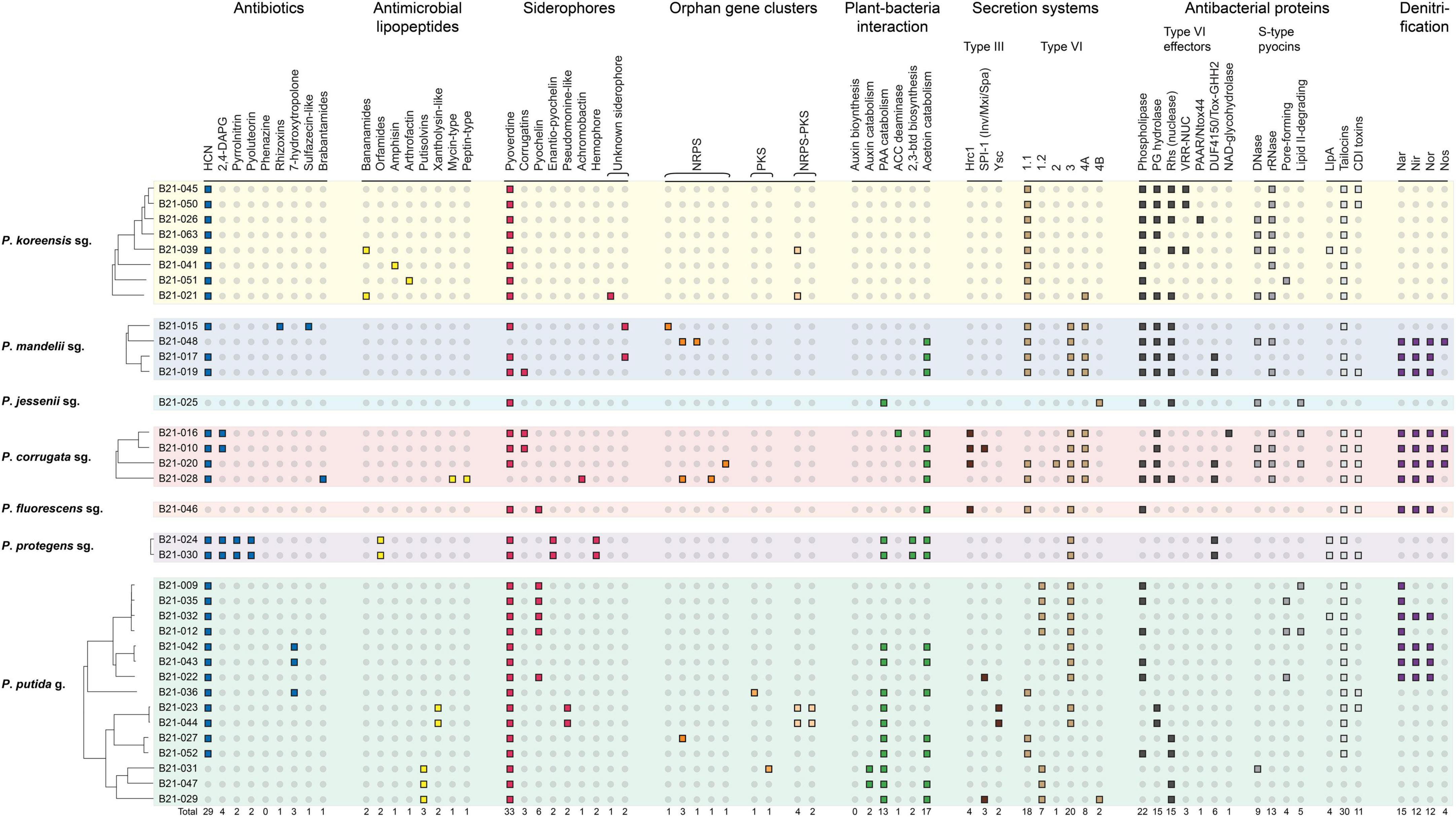
Figure 3. Distribution of diverse genes and clusters involved in plant-bacteria interactions, microbial competition, and biocontrol in the genomes of the 35 Pseudomonas strains. HCN, hydrogen cyanide; 2,4-DAPG, 2,4-diacetylphloroglucinol; NRPS, non-ribosomal peptide synthase; PKS, polyketide synthase; PAA, phenylacetic acid; ACC, 1-aminocyclopropane-1-carboxylate; 2,3-btd, 2,3-butanediol; PG, peptidoglycan; Rhs, VRR-NUC, PAAR, Ntox44, DUF4150, and Tox-GHH2, Pfam domains harbored by some of the Type VI effectors; NAD, Nicotinamide adenine dinucleotide; Llpa, lectin-like putidacin A; CDI, contact-dependent inhibition; sg., subgroup; g., group.
Twenty-nine out of the 35 strains carry BGCs involved in the production of one to four antibiotics. These strains all potentially produce hydrogen cyanide, and some of them carry the gene clusters involved in 2,4-diacetylphloroglucinol, pyrrolnitrin, pyoluteorin, rhizoxins, 7-hydroxytropolone, and/or brabantamides synthesis.
Genes involved in siderophore production were found in all strains except B21-048, which even lacks the biosynthetic genes to produce pyoverdine. Sixteen strains also carry BGCs responsible for the biosynthesis of one or two additional siderophores. Six strains, mainly belonging to the P. putida group, carry the BGC involved in pyochelin production. BGCs putatively responsible for the synthesis of two unknown siderophores were also detected in three strains.
BGCs involved in the production of antimicrobial lipopeptides were found in 12 out of 35 strains. They are predicted to encode lipopeptides with various structures, containing between 8 and 22 amino acids.
As for secretion systems and antibacterial proteins, T3SS clusters were detected in eight strains and belong to three distinct families, i.e., Hrc1, SPI-1 (Inv/Mxi/Spa), and Ysc (Supplementary Figure 3). One strain, B21-010, carries two distinct T3SS clusters. Fifty-six T6SS clusters were found in the genomes of the 35 strains under study. Each strain harbors at least one T6SS cluster and up to four distinct ones. These T6SS clusters belong to six different groups (Supplementary Figure 4). Diverse antibacterial proteins were uncovered in the genomes, including type VI effectors and S-type pyocins, as well as contact-dependent inhibition systems and tailocins (Supplementary Table 5). Some strains harbor up to four S-type pyocins, and up to nine type VI effectors. Three strains carry neither S-type pyocin nor type VI effector genes: B21-032, B21-042, and B21-036. In addition, we found genes potentially involved in the biosynthesis of tailocins in the mutS–cinA intergenic region in 31 strains.
3.4 Phylogenetic relationships and some genomic traits correlate with inhibition abilities
Strains belonging to the P. putida phylogenomic group displayed significantly larger inhibition zones on average than those from the P. fluorescens group, against all bacterial pathogens under study (Figures 2, 4). Strains from the P. putida group generated inhibition zones on average 27% larger than those from the P. fluorescens group when tested against X. hortorum pv. vitians, and 83% larger against P. cichorii and P. carotovorum.
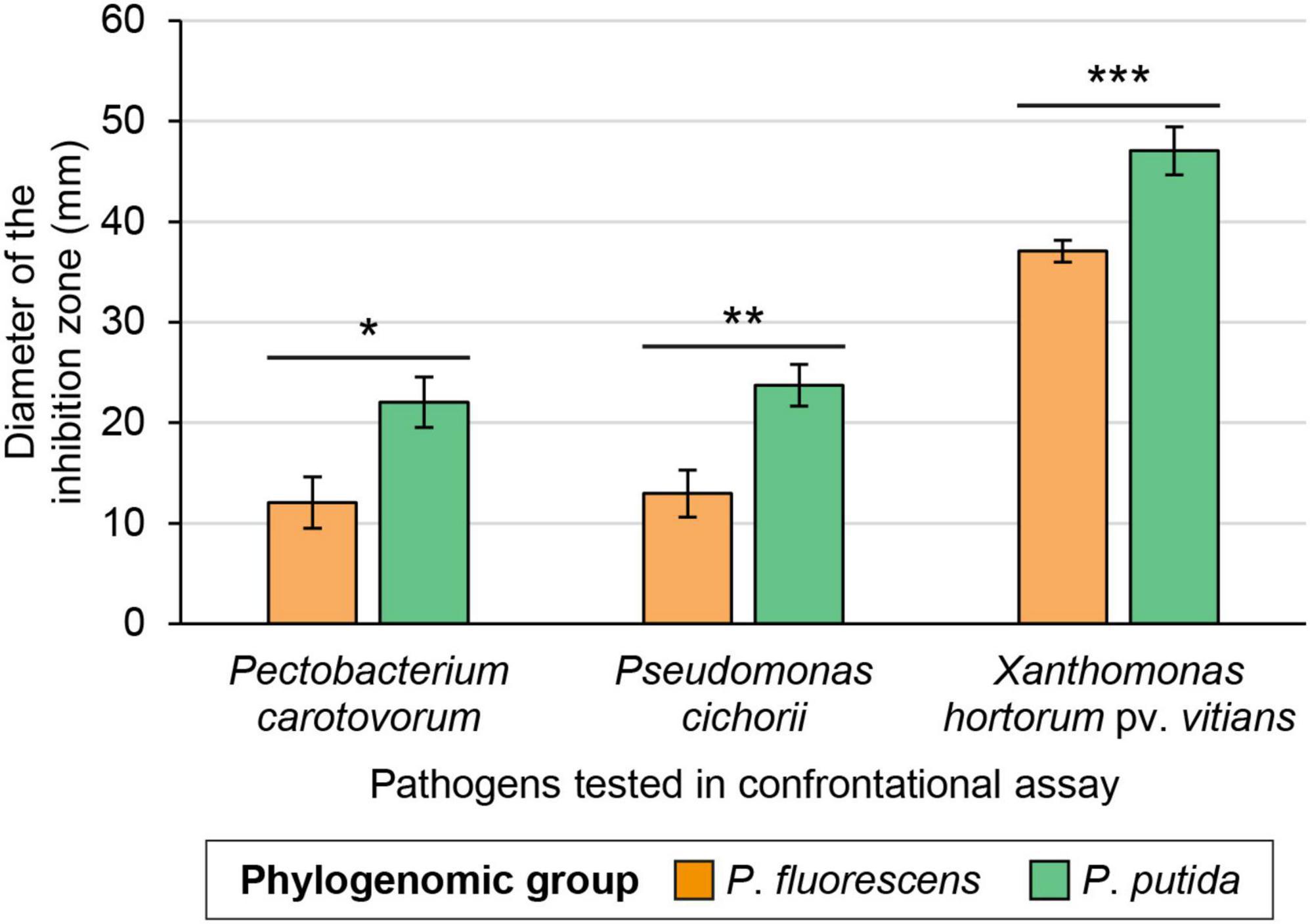
Figure 4. Average in vitro inhibition abilities of the Pseudomonas strains against three lettuce bacterial pathogens according to their phylogenomic group—either P. fluorescens (n = 20) or P. putida (n = 15). One, two, or three asterisks refer to p-values below 0.05, 0.01, and 0.001, respectively (Wilcoxon-Mann-Whitney test). Error bars, standard error.
To better appreciate the potential contribution of some traits in pathogen inhibition abilities, we compared inhibition abilities of strains carrying a given gene or BGC (Figure 3) against strains not carrying it. The results are presented in Table 2. Five BGCs were positively associated with the ability to generate an inhibition zone against one or more of the tested pathogens. The genes involved in the biosynthesis of hydrogen cyanide (HCN), tailocins, and a group-3-type T6SS positively correlated with the ability of inhibiting P. carotovorum, and no other pathogen. The strains carrying the hcn cluster, encoding enzymes required for HCN biosynthesis, produced an inhibition zone 3.3 times larger than the strains not carrying it. The BGC of the group-2 T6SS was linked to higher inhibition levels against both P. cichorii and X. hortorum pv. vitians. The pyochelin BGC was positively associated with inhibition abilities against all three pathogens.
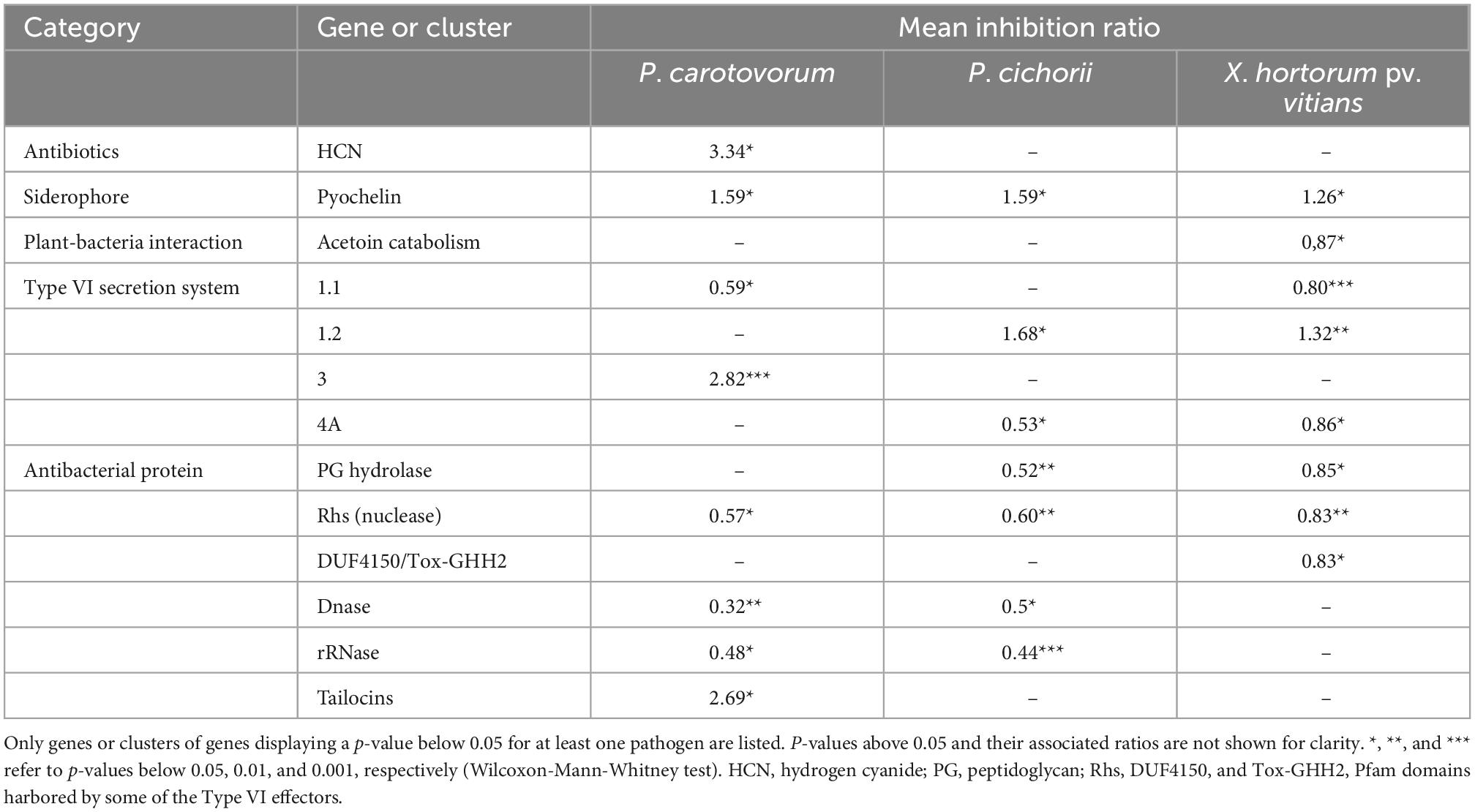
Table 2. Mean inhibition ratios and associated p-values between strains displaying and strains not displaying a given gene or gene cluster in their genomes, considering inhibition against P. carotovorum, P. cichorii, or X. hortorum pv. vitians.
Other genes or BGCs were negatively associated with inhibition abilities against some of or all the bacterial pathogens. They are especially involved in the production of T6SS belonging to two groups and of antibacterial proteins, including some S-type pyocins and T6SS effectors.
4 Discussion
This study aimed at isolating and characterizing Pseudomonas strains displaying inhibitory activity against three lettuce bacterial pathogens, namely X. hortorum pv. vitians, P. cichorii, and P. carotovorum. More than 1,200 Pseudomonas strains were screened for their inhibition potential against these pathogens using a newly developed inhibition assay, resulting in the identification of 35 Pseudomonas strains able to inhibit the growth of some or all three pathogens. Their genomes were entirely sequenced and analyzed in search of a species-level identification and of genes or BGCs potentially responsible for biocontrol, plant-growth promotion, and rhizocompetence traits.
The isolated strains belonging to the P. putida phylogenomic group displayed on average better in vitro inhibitory abilities against the three lettuce bacterial pathogens than those belonging to the P. fluorescens group (Figure 4). The latter group has been extensively studied for its numerous PGPR isolates, contrary to the former (Höfte, 2021; Costa-Gutierrez et al., 2022). Among the 15 isolated strains belonging to the P. putida group, various strains carry biocontrol-related genes or BGCs that are almost absent from the isolated strains belonging to the P. fluorescens group (Figure 3). These genes or BGCs are especially linked to the biosynthesis of 7-hydroxytropolone, putisolvins, xantholysin-like compounds, pyochelin, pseudomonine-like compounds, and unknown molecules produced by non-ribosomal peptide synthases-polyketide synthases (NRPS-PKS) and PKS. These compounds may contribute to some extent to the better inhibitory activity observed in strains belonging to the P. putida group, compared to those in the P. fluorescens group. The compound 7-hydroxytropolone is an iron-chelating and antimicrobial molecule whom BGC has been uncovered in the genome of three Pseudomonas species so far: P. donghuensis, “P. qingdaonensis,” and P. wadenswilerensis (Jiang et al., 2016; Krzyżanowska et al., 2021). In this study, the associated BGC was found in strains belonging to the first two species and not in the only strain of P. wadenswilerensis recovered. This compound is involved in the inhibition of the nematode Caenorhabditis elegans (Gui et al., 2020), phytopathogenic fungi (Muzio et al., 2020; Tao et al., 2020), and various bacterial phytopathogens, including Pectobacterium brasiliense (formerly known as Pectobacterium carotovorum subsp. brasiliense) (Krzyżanowska et al., 2016; Muzio et al., 2020; Matuszewska et al., 2021). Putisolvins and xantholysins are two cyclic lipopeptides mainly, if not solely, described in P. putida strains (Kuiper et al., 2004; Li et al., 2013; Höfte, 2021). Putisolvins are involved in swarming of the producing strains and can inhibit biofilm formation in vitro in other Pseudomonas strains (Kuiper et al., 2004). Their role in the biocontrol of plant pathogens in planta remains to be demonstrated (Kruijt et al., 2009). Xantholysins, mainly represented by xantholysin A, are involved in the inhibition of aphids (Lim et al., 2017), as well as of fungal and bacterial pathogens, including X. hortorum (Li et al., 2013). Five of the six strains carrying the pyochelin BGC belong to the P. putida group and these five strains display medium to high inhibition levels against the three pathogens studied. Pyochelin is a siderophore that has been extensively studied in the opportunistic pathogen P. aeruginosa PAO1 (Gross and Loper, 2009), but rarely in Pseudomonas strains with biocontrol activity. Its diastereoisomers, enantio-pyochelin, has been more often identified in such strains (Youard et al., 2007; Garrido-Sanz et al., 2016). Pyochelin from the strain PAO1 has been shown to inhibit in vitro some plant pathogens belonging to the Xanthomonas genus (Adler et al., 2012). In the isolated Pseudomonas strains, this points to a potential role of this siderophore in the inhibition of the three lettuce bacterial pathogens. Pseudomonine and pseudomonine-like compounds are siderophores identified in various Pseudomonas strains of biocontrol interest (Mercado-Blanco et al., 2001; Loper et al., 2012; Biessy et al., 2019; Oluwabusola et al., 2021). They share structural similarities with pyochelin (Gross and Loper, 2009). Some of these compounds have been shown to inhibit the human bacterial pathogen Mycobacterium tuberculosis in vitro (Oluwabusola et al., 2021). Another family of BGCs found in the genome of the 35 strains under study pertains to NRPS and PKS. NRPS are large multimodular megaenzymes known to produce a great diversity of bioactive secondary metabolites in bacteria and fungi, including cyclic lipopeptides (Hur et al., 2012). PKS produce various bioactive compounds as well, such as 2,4-diacetylphloroglucinol (DAPG), and encompass, but are not limited to, large and modular enzymes (Gross and Loper, 2009). NRPS and PKS act sometimes in combination to synthesize compounds, such as the antibiotic pyoluteorin. These BGCs may be involved in the biosynthesis of undescribed compounds displaying biocontrol activity. Analytical chemistry and mutagenesis approaches are needed to decipher their products and potential role(s) for their producing strains.
In vitro inhibition of the three pathogens X. hortorum pv. vitians, P. cichorii, and P. carotovorum by some of the isolated Pseudomonas strains positively correlates with the presence of genes involved in pyochelin and T6SSs biosynthesis (Table 2). These might be involved in pathogen inhibition. Pyochelin has already been discussed in this paper because of the presence of its BGC in strains almost exclusively belonging to the P. putida group. As for the T6SSs, they are membrane-embedded contractile nanomachines that secrete often toxic effectors acting in the periplasm or cytoplasm of target cells (Bernal et al., 2018; Jurënas and Journet, 2021). A T6SS has especially been shown to mediate leaf protection by P. putida KT2440 against X. campestris in Nicotiana benthamiana (Bernal et al., 2017). However, T6SS-mediated inhibition is often considered as a contact-dependent mechanism (Coulthurst, 2019). In our inhibition assays, we focused on the diameter of the inhibition zone, which would not uncover any potential contact-dependent inhibitory effect usually expected with T6SSs. This would explain why the presence of the T6SS effectors searched for in the genomes of the 35 Pseudomonas strains did not positively correlate with their inhibition abilities against the three pathogens. It would potentially point to another role of the T6SSs whose presence in the genomes positively correlates with inhibition abilities. In some cases, T6SSs have been shown to mediate a remote effect, especially through iron acquisition. Chen et al. (2016) have shown that a Pseudomonas strain isolated from soil, P. taiwanensis CMST, was able to use its T6SS to secrete newly synthesized pyoverdine, a siderophore, from the periplasm to the extracellular medium. This T6SS-mediated secretion of pyoverdine contributed to the antagonistic activity of this strain against X. oryzae, a rice pathogen. Interestingly, almost all 35 strains carry the BGC responsible for pyoverdine production. Besides, Lin et al. (2017) have shown in P. aeruginosa PAO1 that the T6SS could also act with a Fe(III)-pyochelin receptor to retrieve iron. This strain uses a T6SS to secrete a protein, TseF, that is incorporated into outer membrane vesicles, where it interacts with the high iron-affinity compound called the Pseudomonas quinolone signal. The protein also interacts with the Fe(III)-pyochelin receptor FptA and the porin OprF, allowing the strain to transfer iron into its cytosol. The presence of the pyochelin BGC correlates with in vitro inhibition of the three plant pathogens as well, suggesting that this T6SS-mediated mechanism might be used by some strains to inhibit the pathogens. Removing T6SS- and/or pyochelin-related genes in some strains while cultivating the resulting mutants in different iron concentrations would determine whether this iron-dependent inhibition is at play.
The presence of genes involved in HCN and tailocins production is linked to antagonistic activity against P. carotovorum only. HCN is a toxic volatile compound affecting a wide array of organisms by disrupting the functions of the cytochrome c oxidase, involved in the respiratory chain, and of other metalloproteins (Gross and Loper, 2009). The biosynthesis of this antimicrobial molecule has been demonstrated in numerous Pseudomonas strains (Ramette et al., 2003; Flury et al., 2017), and its conserved BGC was found in the genome of numerous strains belonging to this genus (Loper et al., 2012; Garrido-Sanz et al., 2016; Biessy et al., 2019). The compound is actively involved in the inhibitory activity of several Pseudomonas strains against a broad range of plant parasites/pathogens, such as insects, nematodes, fungi, and bacteria (Höfte, 2021). Such activity has been seldom reported against bacterial phytopathogens. In 2012, Lanteigne et al. (2012) demonstrated that the strain P. brassicacearum LBUM300 inhibited the bacterial plant pathogen Clavibacter michiganensis subsp. michiganensis through the production of HCN and other antibiotics. However, another research team found no correlation between HCN production and the inhibition of bacterial plant pathogens by some rhizobacteria, including P. carotovorum and X. campestris pv. campestris (Rijavec and Lapanje, 2016). Tailocins are phage tail-like antibacterial proteins found in various genera belonging to the Pseudomonadota phylum, including the genus Pseudomonas (Ghequire and De Mot, 2014; Príncipe et al., 2018). They directly induce a depolarization of the membrane of their targets, leading to cell death. These proteins are known to affect closely related bacteria, usually belonging to the same genus or species (Scholl, 2017), but have sometimes been demonstrated to inhibit bacteria outside of their genus, such as the plant pathogen Erwinia amylovora (Jabrane et al., 2002; Ghequire and De Mot, 2014). To our knowledge, P. carotovorum has never been shown to be affected by tailocins. However, two bacteriophages, PP1 and PP16, effectively inhibit this pathogen and represent promising biocontrol tools (Lim et al., 2013; Voronina et al., 2019). Given the structural similarity between tailocins and bacteriophages, tailocins produced by some Pseudomonas strains might be affecting P. carotovorum. The potential role of tailocins and HCN in pathogen inhibition should be assessed through a reverse genetics approach.
The 35 inhibitory Pseudomonas strains display a high degree of diversity, encompassing two phylogenomic groups, six subgroups, and at least 27 species. Many of these species are represented by few if any strains of biocontrol interest, such as P. laurylsulfatiphila and P. sichuanensis, and have been recently described (Furmanczyk et al., 2018; Qin et al., 2019; Girard et al., 2021). Some other strains isolated in this study belong to species well known for their numerous PGPR representatives, such as P. brassicacearum, P. protegens, and P. putida (Paulin et al., 2017; Nelkner et al., 2019; Höfte, 2021; Costa-Gutierrez et al., 2022). This diversity arose thanks to the numerous soil sample collection sites and the discriminating strain isolation process, based only on a Pseudomonas-selective medium and a binary inhibition criterion against the three lettuce bacterial pathogens. Such diversity has led to the identification of a wide range of plant growth promotion-related genes and BGCs in the genomes, heterogeneously distributed among the strains. This high heterogeneity made difficult the identification of correlations between the presence of some genes or BGCs and the inhibitory abilities of the Pseudomonas strains, but potentially expanded the number of biocontrol mechanisms at work against the plant pathogens studied. Furthermore, the presence of a BGC in a genome does not necessarily indicate whether and how much of the corresponding compound is produced (Biessy et al., 2021). Better understanding these potentially diverse mechanisms and biosynthesis pathways may be central to develop effective Pseudomonas-based biocontrol strategies against bacterial pathogens in lettuce fields.
5 Conclusion
A novel assay was developed to enable the rapid screening of 1,210 Pseudomonas strains for their antagonistic properties against three lettuce bacterial pathogens. Among these, 35 Pseudomonas strains identified for their in vitro inhibitory abilities against these pathogens are promising. Many biocontrol-related genes and BGCs have been identified and are potentially involved in various mechanisms to inhibit the targeted pathogens. These mechanisms will have to be validated by using reverse genetics and analytical chemistry approaches, while performing greenhouse and field experiments to determine the most effective strains to prevent bacterial disease development in an agricultural context. This work paves the way to sustainable and preventive lettuce protection methods against bacterial pathogens and contributes to the expansion of quality genomic data for Pseudomonas species, especially those for which little information is available.
Data availability statement
The complete genome sequences of the 35 Pseudomonas strains have been deposited at DDBJ/ENA/GenBank under the accession numbers provided in Table 1. PacBio sequencing reads have been deposited into the Sequence Read Archive (BioProject PRJNA779451) under the accession numbers provided in Supplementary Table 2. The genome versions described in this manuscript are the first versions.
Author contributions
AZ, AB, MCi, MCa, DA, and MF: conceptualization. AB, MCi, MCa, and MF: methodology. JB: software. AZ, AB, MCi, and MCa: formal analysis. AZ, AB, MCi, MCa, and DA: investigation. AB: data curation. AZ and AB: writing—original draft and visualization. AZ, AB, MCi, MCa, DA, JB, and MF: writing—review and editing. MF: supervision and funding acquisition. All authors contributed to the article and approved the submitted version.
Funding
This work was supported by the Fondation Laitue (http://fondationlaitue.ca) and Agriculture and Agri-Food Canada.
Acknowledgments
We would like to thank all the growers who allowed us to collect soil samples on their farms and the Fondation Laitue for its financial support.
Conflict of interest
The authors declare that the research was conducted in the absence of any commercial or financial relationships that could be construed as a potential conflict of interest.
Publisher’s note
All claims expressed in this article are solely those of the authors and do not necessarily represent those of their affiliated organizations, or those of the publisher, the editors and the reviewers. Any product that may be evaluated in this article, or claim that may be made by its manufacturer, is not guaranteed or endorsed by the publisher.
Supplementary material
The Supplementary Material for this article can be found online at: https://www.frontiersin.org/articles/10.3389/fmicb.2022.1038888/full#supplementary-material
References
Adesina, M. F., Lembke, A., Costa, R., Speksnijder, A., and Smalla, K. (2007). Screening of bacterial isolates from various European soils for in vitro antagonistic activity towards Rhizoctonia solani and Fusarium oxysporum: Site-dependent composition and diversity revealed. Soil. Biol. Biochem. 39, 2818–2828. doi: 10.1016/j.soilbio.2007.06.004
Adler, C., Corbalán, N. S., Seyedsayamdost, M. R., Pomares, M. F., de Cristóbal, R. E., Clardy, J., et al. (2012). Catecholate siderophores protect bacteria from pyochelin toxicity. PLoS One 7:e46754. doi: 10.1371/journal.pone.0046754
Affia, H. (2016). Évaluation de différents sels et mélanges de sels pour lutter contre Pseudomonas cichorii dans la laitue. Laval, QC: Université Laval.
Aggeli, F., Ziogas, I., Gkizi, D., Fragkogeorgi, G. A., and Tjamos, S. E. (2020). Novel biocontrol agents against Rhizoctonia solani and Sclerotinia sclerotiorum in lettuce. BioControl 65, 763–773. doi: 10.1007/s10526-020-10043-w
Balthazar, C., Novinscak, A., Cantin, G., Joly, D. L., and Filion, M. (2021). Biocontrol activity of Bacillus spp. and Pseudomonas spp. against Botrytis cinerea and other cannabis fungal pathogens. Phytopathology 112, 549–560. doi: 10.1094/PHYTO-03-21-0128-R
Berendsen, R. L., van Verk, M. C., Stringlis, I. A., Zamioudis, C., Tommassen, J., Pieterse, C. M. J., et al. (2015). Unearthing the genomes of plant-beneficial Pseudomonas model strains WCS358, WCS374 and WCS417. BMC Genomics 16:539. doi: 10.1186/s12864-015-1632-z
Bernal, P., Allsopp, L. P., Filloux, A., and Llamas, M. A. (2017). The Pseudomonas putida T6SS is a plant warden against phytopathogens. ISME J. 11, 972–987. doi: 10.1038/ismej.2016.169
Bernal, P., Llamas, M. A., and Filloux, A. (2018). Type VI secretion systems in plant-associated bacteria. Environ. Microbiol. 20, 1–15. doi: 10.1111/1462-2920.13956
Biessy, A., Novinscak, A., Blom, J., Léger, G., Thomashow, L. S., Cazorla, F. M., et al. (2019). Diversity of phytobeneficial traits revealed by whole-genome analysis of worldwide-isolated phenazine-producing Pseudomonas spp. Environ. Microbiol. 21, 437–455. doi: 10.1111/1462-2920.14476
Biessy, A., Novinscak, A., St-Onge, R., Léger, G., Zboralski, A., and Filion, M. (2021). Inhibition of three potato pathogens by phenazine-producing Pseudomonas spp. is associated with multiple biocontrol-related traits. mSphere 6:e0042721. doi: 10.1128/mSphere.00427-21
Blin, K., Shaw, S., Kloosterman, A. M., Charlop-Powers, Z., van Wezel, G. P., Medema, M. H., et al. (2021). antiSMASH 6.0: Improving cluster detection and comparison capabilities. Nucleic. Acids Res. 49, W29–W35. doi: 10.1093/nar/gkab335
Blom, J., Albaum, S. P., Doppmeier, D., Pühler, A., Vorhölter, F.-J., Zakrzewski, M., et al. (2009). EDGAR: A software framework for the comparative analysis of prokaryotic genomes. BMC Bioinformatics 10:154. doi: 10.1186/1471-2105-10-154
Büttner, D., and Bonas, U. (2010). Regulation and secretion of Xanthomonas virulence factors. FEMS Microbiol. Rev. 34, 107–133. doi: 10.1111/j.1574-6976.2009.00192.x
Cariddi, C., and Sanzani, S. M. (2013). A severe outbreak of bacterial lettuce soft rot caused by Pectobacterium carotovorum subsp. carotovorum in Apulia (Italy). J. Plant. Pathol. 95, 441–446.
Carisse, O., Ouimet, A., Toussaint, V., and Philion, V. (2000). Evaluation of the effect of seed treatments, bactericides, and cultivars on bacterial leaf spot of lettuce caused by Xanthomonas campestris pv. vitians. Plant Dis. 84, 295–299. doi: 10.1094/PDIS.2000.84.3.295
Chen, W.-J., Kuo, T.-Y., Hsieh, F.-C., Chen, P.-Y., Wang, C.-S., Shih, Y.-L., et al. (2016). Involvement of type VI secretion system in secretion of iron chelator pyoverdine in Pseudomonas taiwanensis. Sci. Rep. 6:32950. doi: 10.1038/srep32950
Collinge, D. B., Jensen, D. F., Rabiey, M., Sarrocco, S., Shaw, M. W., and Shaw, R. (2022). Biological control of plant diseases – what has been achieved and what is the direction? Plant. Pathol. 71, 1024–1047. doi: 10.1111/ppa.13555
Cook, R. J., and Baker, K. F. (1983). The nature and practice of biological control of plant pathogens. St. Paul, MN: The American Phytopathological Society.
Costa-Gutierrez, S. B., Adler, C., Espinosa-Urgel, M., and de Cristóbal, R. E. (2022). Pseudomonas putida and its close relatives: Mixing and mastering the perfect tune for plants. Appl. Microbiol. Biotechnol. 106, 3351–3367. doi: 10.1007/s00253-022-11881-7
Coulthurst, S. (2019). The type VI secretion system: A versatile bacterial weapon. Microbiology 165, 503–515. doi: 10.1099/mic.0.000789
Davidsson, P. R., Kariola, T., Niemi, O., and Palva, E. T. (2013). Pathogenicity of and plant immunity to soft rot pectobacteria. Front. Plant Sci. 4:191. doi: 10.3389/fpls.2013.00191
de Mendiburu, F. (2017). agricolae: Statistical procedures for agricultural research. R package version 1.2-8. Available online at: https://CRAN.R-project.org/package=agricolae.
Dia, N. C., Morinière, L., Cottyn, B., Bernal, E., Jacobs, J. M., Koebnik, R., et al. (2022). Xanthomonas hortorum – beyond gardens: Current taxonomy, genomics, and virulence repertoires. Mol. Plant Pathol. 23, 597–621. doi: 10.1111/mpp.13185
Dieckmann, M. A., Beyvers, S., Nkouamedjo-Fankep, R. C., Hanel, P. H. G., Jelonek, L., Blom, J., et al. (2021). EDGAR3.0: Comparative genomics and phylogenomics on a scalable infrastructure. Nucleic Acids Res. 49, W185–W192. doi: 10.1093/nar/gkab341
Edgar, R. C. (2004). MUSCLE: Multiple sequence alignment with high accuracy and high throughput. Nucleic Acids Res. 32, 1792–1797. doi: 10.1093/nar/gkh340
Fatmi, M., and Bolkan, H. (2017). “Bacterial diseases of plants: Epidemiology and management strategies,” in Detection of plant-pathogenic bacteria in seed and other planting material, eds M. Fatmi, R. R. Walcott, and N. W. Schaad (St. Paul, MN: The American Phytopathological Society), 3–9.
Felsenstein, J. (2005). PHYLIP (phylogeny inference package) version 3.6. Seattle, DC: University of Washington.
Flury, P., Vesga, P., Péchy-Tarr, M., Aellen, N., Dennert, F., Hofer, N., et al. (2017). Antimicrobial and insecticidal: Cyclic lipopeptides and hydrogen cyanide produced by plant-beneficial Pseudomonas strains CHA0, CMR12a, and PCL1391 contribute to insect killing. Front. Microbiol. 8:100. doi: 10.3389/fmicb.2017.00100
Food and Agriculture Organization of the United Nations (2021). FAOSTAT database. Rome: Food and Agriculture Organization of the United Nations.
Furmanczyk, E. M., Lipinski, L., Dziembowski, A., and Sobczak, A. (2018). Genomic and functional characterization of environmental strains of SDS-degrading Pseudomonas spp., providing a source of new sulfatases. Front. Microbiol. 9:1795. doi: 10.3389/fmicb.2018.01795
Garrido-Sanz, D., Meier-Kolthoff, J. P., Göker, M., Martín, M., Rivilla, R., and Redondo-Nieto, M. (2016). Genomic and genetic diversity within the Pseudomonas fluorescens complex. PLoS One 11:e0150183. doi: 10.1371/journal.pone.0150183
Ghequire, M. G. K., and De Mot, R. (2014). Ribosomally encoded antibacterial proteins and peptides from Pseudomonas. FEMS Microbiol. Rev. 38, 523–568. doi: 10.1111/1574-6976.12079
Girard, L., Lood, C., Höfte, M., Vandamme, P., Rokni-Zadeh, H., van Noort, V., et al. (2021). The ever-expanding Pseudomonas genus: Description of 43 new species and partition of the Pseudomonas putida group. Microorganisms 9:1766. doi: 10.3390/microorganisms9081766
Gislin, D., Sudarsanam, D., Antony Raj, G., and Baskar, K. (2018). Antibacterial activity of soil bacteria isolated from Kochi, India and their molecular identification. J. Genet. Eng. Biotechnol. 16, 287–294. doi: 10.1016/j.jgeb.2018.05.010
Götze, S., and Stallforth, P. (2020). Structure, properties, and biological functions of nonribosomal lipopeptides from pseudomonads. Nat. Prod. Rep. 37, 29–54. doi: 10.1039/C9NP00022D
Grosch, R., Faltin, F., Lottmann, J., Kofoet, A., and Berg, G. (2005). Effectiveness of 3 antagonistic bacterial isolates to control Rhizoctonia solani Kühn on lettuce and potato. Can. J. Microbiol. 51, 345–353. doi: 10.1139/w05-002
Gross, H., and Loper, J. E. (2009). Genomics of secondary metabolite production by Pseudomonas spp. Nat. Prod. Rep. 26, 1408–1446. doi: 10.1039/b817075b
Gui, Z., You, J., Xie, G., Qin, Y., Wu, T., and Xie, Z. (2020). Pseudomonas donghuensis HYS 7-hydroxytropolone contributes to pathogenicity toward Caenorhabditis elegans and is influenced by pantothenic acid. Biochem. Biophys. Res. Commun. 533, 50–56. doi: 10.1016/j.bbrc.2020.08.067
Hébert, P.-O., Laforest, M., Xu, D., Ciotola, M., Cadieux, M., Beaulieu, C., et al. (2021). Genotypic and phenotypic characterization of lettuce bacterial pathogen Xanthomonas hortorum pv. vitians populations collected in Quebec. Canada. Agronomy 11:2386. doi: 10.3390/agronomy11122386
Hikichi, Y., Wali, U. M., Ohnishi, K., and Kiba, A. (2013). Mechanism of disease development caused by a multihost plant bacterium, Pseudomonas cichorii, and its virulence diversity. J. Gen. Plant Pathol. 79, 379–389. doi: 10.1007/s10327-013-0461-7
Höfte, M. (2021). “The use of Pseudomonas spp. as bacterial biocontrol agents to control plant disease,” in Microbial bioprotectants for plant disease management, eds J. Köhl and W. J. Ravensberg (Cambridge: Burleigh Dodds Science Publishing Limited), 1–74. doi: 10.19103/AS.2021.0093.11
Huang, C.-J., Pauwelyn, E., Ongena, M., Debois, D., Leclère, V., Jacques, P., et al. (2015). Characterization of cichopeptins, new phytotoxic cyclic lipodepsipeptides produced by Pseudomonas cichorii SF1-54 and their role in bacterial midrib rot disease of lettuce. Mol. Plant Microbe Interact. 28, 1009–1022. doi: 10.1094/MPMI-03-15-0061-R
Hur, G. H., Vickery, C. R., and Burkart, M. D. (2012). Explorations of catalytic domains in non-ribosomal peptide synthetase enzymology. Nat. Prod. Rep. 29, 1074–1098. doi: 10.1039/c2np20025b
Jabrane, A., Sabri, A., Compère, P., Jacques, P., Vandenberghe, I., Van Beeumen, J., et al. (2002). Characterization of serracin P, a phage-tail-like bacteriocin, and its activity against Erwinia amylovora, the fire blight pathogen. Appl. Environ. Microbiol. 68, 5704–5710. doi: 10.1128/AEM.68.11.5704-5710.2002
Jiang, Z., Chen, M., Yu, X., and Xie, Z. (2016). 7-hydroxytropolone produced and utilized as an iron-scavenger by Pseudomonas donghuensis. Biometals 29, 817–826. doi: 10.1007/s10534-016-9954-0
Jurënas, D., and Journet, L. (2021). Activity, delivery, and diversity of Type VI secretion effectors. Mol. Microbiol. 115, 383–394. doi: 10.1111/mmi.14648
King, E. O., Ward, M. K., and Raney, D. E. (1954). Two simple media for the demonstration of pyocyanin and fluorescin. J. Lab. Clin. Med. 44, 301–307. doi: 10.5555/uri:pii:002221435490222X
Kolmogorov, M., Yuan, J., Lin, Y., and Pevzner, P. A. (2019). Assembly of long, error-prone reads using repeat graphs. Nat. Biotechnol. 37, 540–546. doi: 10.1038/s41587-019-0072-8
Kruijt, M., Tran, H., and Raaijmakers, J. M. (2009). Functional, genetic and chemical characterization of biosurfactants produced by plant growth-promoting Pseudomonas putida 267. J. Appl. Microbiol. 107, 546–556. doi: 10.1111/j.1365-2672.2009.04244.x
Krzyżanowska, D. M., Iwanicki, A., Czajkowski, R., and Jafra, S. (2021). High-quality complete genome resource of tomato rhizosphere strain Pseudomonas donghuensis P482, a representative of a species with biocontrol activity against plant pathogens. Mol. Plant Microbe Interact 34, 1450–1454. doi: 10.1094/MPMI-06-21-0136-A
Krzyżanowska, D. M., Ossowicki, A., Rajewska, M., Maciąg, T., Jabłońska, M., Obuchowski, M., et al. (2016). When genome-based approach meets the “old but good”: Revealing genes involved in the antibacterial activity of Pseudomonas sp. P482 against soft rot pathogens. Front. Microbiol. 7:782. doi: 10.3389/fmicb.2016.00782
Kuiper, I., Lagendijk, E. L., Pickford, R., Derrick, J. P., Lamers, G. E. M., Thomas-Oates, J. E., et al. (2004). Characterization of two Pseudomonas putida lipopeptide biosurfactants, putisolvin I and II, which inhibit biofilm formation and break down existing biofilms. Mol. Microbiol. 51, 97–113. doi: 10.1046/j.1365-2958.2003.03751.x
Lanteigne, C., Gadkar, V. J., Wallon, T., Novinscak, A., and Filion, M. (2012). Production of DAPG and HCN by Pseudomonas sp. LBUM300 contributes to the biological control of bacterial canker of tomato. Phytopathology 102, 967–973. doi: 10.1094/PHYTO-11-11-0312
Li, W., Rokni-Zadeh, H., De Vleeschouwer, M., Ghequire, M. G. K., Sinnaeve, D., Xie, G.-L., et al. (2013). The antimicrobial compound xantholysin defines a new group of Pseudomonas cyclic lipopeptides. PLoS One 8:e62946. doi: 10.1371/journal.pone.0062946
Lim, D. J., Yang, S. Y., Noh, M. Y., Lee, C. W., Kim, J. C., and Kim, I. S. (2017). Identification of lipopeptide xantholysins from Pseudomonas sp. DJ15 and their insecticidal activity against Myzus persicae. Entomol. Res. 47, 337–343. doi: 10.1111/1748-5967.12241
Lim, J.-A., Jee, S., Lee, D. H., Roh, E., Jung, K., Oh, C., et al. (2013). Biocontrol of Pectobacterium carotovorum subsp. carotovorum using bacteriophage PP1. J. Microbiol. Biotechnol. 23:7. doi: 10.4014/jmb.1304.04001
Lin, J., Zhang, W., Cheng, J., Yang, X., Zhu, K., Wang, Y., et al. (2017). A Pseudomonas T6SS effector recruits PQS-containing outer membrane vesicles for iron acquisition. Nat. Commun. 8:14888. doi: 10.1038/ncomms14888
Loper, J. E., Hassan, K. A., Mavrodi, D. V., Davis, E. W., Lim, C. K., Shaffer, B. T., et al. (2012). Comparative genomics of plant-associated Pseudomonas spp.: Insights into diversity and inheritance of traits involved in multitrophic interactions. PLoS Genet. 8:e1002784. doi: 10.1371/journal.pgen.1002784
Lugtenberg, B., and Kamilova, F. (2009). Plant-growth-promoting rhizobacteria. Annu. Rev. Microbiol. 63, 541–556. doi: 10.1146/annurev.micro.62.081307.162918
Matuszewska, M., Maciąg, T., Rajewska, M., Wierzbicka, A., and Jafra, S. (2021). The carbon source-dependent pattern of antimicrobial activity and gene expression in Pseudomonas donghuensis P482. Sci. Rep. 11:10994. doi: 10.1038/s41598-021-90488-w
McSpadden Gardener, B. B., Mavrodi, D. V., Thomashow, L. S., and Weller, D. M. (2001). A rapid polymerase chain reaction-based assay characterizing rhizosphere populations of 2,4-diacetylphloroglucinol-producing bacteria. Phytopathology 91, 44–54. doi: 10.1094/PHYTO.2001.91.1.44
Meier-Kolthoff, J. P., and Göker, M. (2019). TYGS is an automated high-throughput platform for state-of-the-art genome-based taxonomy. Nat. Commun. 10:2182. doi: 10.1038/s41467-019-10210-3
Meier-Kolthoff, J. P., Auch, A. F., Klenk, H.-P., and Göker, M. (2013). Genome sequence-based species delimitation with confidence intervals and improved distance functions. BMC Bioinformatics 14:60. doi: 10.1186/1471-2105-14-60
Meier-Kolthoff, J. P., Carbasse, J. S., Peinado-Olarte, R. L., and Göker, M. (2022). TYGS and LPSN: A database tandem for fast and reliable genome-based classification and nomenclature of prokaryotes. Nucleic Acids Res. 50, D801–D807. doi: 10.1093/nar/gkab902
Mercado-Blanco, J., van der Drift, K. M. G. M., Olsson, P. E., Thomas-Oates, J. E., van Loon, L. C., and Bakker, P. A. H. M. (2001). Analysis of the pmsCEAB gene cluster involved in biosynthesis of salicylic acid and the siderophore pseudomonine in the biocontrol strain Pseudomonas fluorescens WCS374. J. Bacteriol. 183, 1909–1920. doi: 10.1128/JB.183.6.1909-1920.2001
Mistry, J., Chuguransky, S., Williams, L., Qureshi, M., Salazar, G. A., Sonnhammer, E. L. L., et al. (2021). Pfam: The protein families database in 2021. Nucleic Acids Res. 49, D412–D419. doi: 10.1093/nar/gkaa913
Morinière, L., Burlet, A., Rosenthal, E. R., Nesme, X., Portier, P., Bull, C. T., et al. (2020). Clarifying the taxonomy of the causal agent of bacterial leaf spot of lettuce through a polyphasic approach reveals that Xanthomonas cynarae Trébaol et al 2000 emend. Timilsina et al 2019 is a later heterotypic synonym of Xanthomonas hortorum Vauterin et al., 1995.. Syst. Appl. Microbiol. 43:126087. doi: 10.1016/j.syapm.2020.126087
Morinière, L., Mirabel, L., Gueguen, E., and Bertolla, F. (2022). A comprehensive overview of the genes and functions required for lettuce infection by the hemibiotrophic phytopathogen Xanthomonas hortorum pv. vitians. mSystems 7:e0129021. doi: 10.1128/msystems.01290-21
Muzio, F. M., Agaras, B. C., Masi, M., Tuzi, A., Evidente, A., and Valverde, C. (2020). 7-hydroxytropolone is the main metabolite responsible for the fungal antagonism of Pseudomonas donghuensis strain SVBP6. Environ. Microbiol. 22, 2550–2563. doi: 10.1111/1462-2920.14925
Nelkner, J., Tejerizo, G. T., Hassa, J., Lin, T. W., Witte, J., Verwaaijen, B., et al. (2019). Genetic potential of the biocontrol agent Pseudomonas brassicacearum (formerly P. trivialis) 3Re2-7 unraveled by genome sequencing and mining, comparative genomics and transcriptomics. Genes (Basel) 10:601. doi: 10.3390/genes10080601
Oluwabusola, E. T., Adebisi, O. O., Reyes, F., Acquah, K. S., De La Cruz, M., Mweetwa, L. L., et al. (2021). Isolation and characterization of new phenolic siderophores with antimicrobial properties from Pseudomonas sp. UIAU-6B. Beilstein J. Org. Chem. 17, 2390–2398. doi: 10.3762/bjoc.17.156
Oren, A., and Garrity, G. M. (2021). Valid publication of the names of forty-two phyla of prokaryotes. Int. J. Syst. Evol. Microbiol. 71:005056. doi: 10.1099/ijsem.0.005056
Palleroni, N. J. (1984). “Genus I Pseudomonas,” in Bergey’s manual of systematic bacteriology, eds N. R. Krieg and J. C. Holt (Baltimore, MD: The Williams and Wilkins Co), 141–199.
Paulin, M. M., Novinscak, A., Lanteigne, C., Gadkar, V. J., and Filion, M. (2017). Interaction between 2,4-diacetylphloroglucinol- and hydrogen cyanide-producing Pseudomonas brassicacearum LBUM300 and Clavibacter michiganensis subsp. Michiganensis in the tomato rhizosphere. Appl. Environ. Microbiol. 83, e73–e17. doi: 10.1128/AEM.00073-17
Pauwelyn, E., Vanhouteghem, K., Cottyn, B., De Vos, P., Maes, M., Bleyaert, P., et al. (2011). Epidemiology of Pseudomonas cichorii, the cause of lettuce midrib rot. J. Phytopathol. (1986) 159, 298–305. doi: 10.1111/j.1439-0434.2010.01764.x
Planchamp, C., Glauser, G., and Mauch-Mani, B. (2015). Root inoculation with Pseudomonas putida KT2440 induces transcriptional and metabolic changes and systemic resistance in maize plants. Front. Plant Sci. 5:719. doi: 10.3389/fpls.2014.00719
Príncipe, A., Fernandez, M., Torasso, M., Godino, A., and Fischer, S. (2018). Effectiveness of tailocins produced by Pseudomonas fluorescens SF4c in controlling the bacterial-spot disease in tomatoes caused by Xanthomonas vesicatoria. Microbiol. Res. 21, 94–102. doi: 10.1016/j.micres.2018.05.010
Qin, J., Hu, Y., Feng, Y., Xaioju, L., and Zong, Z. (2019). Pseudomonas sichuanensis sp. nov., isolated from hospital sewage. Int. J. Syst. Evol. Microbiol. 69, 517–522. doi: 10.1099/ijsem.0.003188
Quevillon, E., Silventoinen, V., Pillai, S., Harte, N., Mulder, N., Apweiler, R., et al. (2005). InterProScan: Protein domains identifier. Nucleic Acids Res. 33, W116–W120. doi: 10.1093/nar/gki442
Ramette, A., Moënne-Loccoz, Y., and Défago, G. (2003). Prevalence of fluorescent pseudomonads producing antifungal phloroglucinols and/or hydrogen cyanide in soils naturally suppressive or conducive to tobacco black root rot. FEMS Microbiol. Ecol. 44, 35–43. doi: 10.1111/j.1574-6941.2003.tb01088.x
Richter, M., Rosselló-Móra, R., Oliver Glöckner, F., and Peplies, J. (2016). JSpeciesWS: A web server for prokaryotic species circumscription based on pairwise genome comparison. Bioinformatics 32, 929–931. doi: 10.1093/bioinformatics/btv681
Rijavec, T., and Lapanje, A. (2016). Hydrogen cyanide in the rhizosphere: Not suppressing plant pathogens, but rather regulating availability of phosphate. Front. Microbiol. 7:1785. doi: 10.3389/fmicb.2016.01785
Scherwinski, K., Grosch, R., and Berg, G. (2008). Effect of bacterial antagonists on lettuce: Active biocontrol of Rhizoctonia solani and negligible, short-term effects on nontarget microorganisms. FEMS Microbiol. Ecol. 64, 106–116. doi: 10.1111/j.1574-6941.2007.00421.x
Scholl, D. (2017). Phage tail–like bacteriocins. Annu. Rev. Virol. 4, 453–467. doi: 10.1146/annurev-virology-101416-041632
Schreiter, S., Babin, D., Smalla, K., and Grosch, R. (2018). Rhizosphere competence and biocontrol effect of Pseudomonas sp. RU47 independent from plant species and soil type at the field scale. Front. Microbiol. 9:97. doi: 10.3389/fmicb.2018.00097
Sottero, A. N., Freitas, S., Melo, A. M. T., and Trani, P. E. (2006). Rizobactérias e alface: Colonização rizosférica, promoção de crescimento e controle biológico. Rev. Bras. Cienc. Solo 30, 225–234. doi: 10.1590/S0100-06832006000200004
Stenberg, J. A. (2021). When is it biological control? A framework of definitions, mechanisms, and classifications. J. Pest. Sci. (2004) 94, 665–676. doi: 10.1007/s10340-021-01354-7
Subbarao, K. V., Davis, R. M., Gilbertson, R. L., and Raid, R. N. (eds) (2017). Compendium of lettuce diseases and pests, 2nd Edn. St. Paul, MN: American Phytopathological Society.
Tao, X., Zhang, H., Gao, M., Li, M., Zhao, T., and Guan, X. (2020). Pseudomonas species isolated via high-throughput screening significantly protect cotton plants against verticillium wilt. AMB Expr. 10:193. doi: 10.1186/s13568-020-01132-1
Tatusova, T., DiCuccio, M., Badretdin, A., Chetvernin, V., Nawrocki, E. P., Zaslavsky, L., et al. (2016). NCBI prokaryotic genome annotation pipeline. Nucleic Acids Res. 44, 6614–6624. doi: 10.1093/nar/gkw569
Timilsina, S., Potnis, N., Newberry, E. A., Liyanapathiranage, P., Iruegas-Bocardo, F., White, F. F., et al. (2020). Xanthomonas diversity, virulence and plant–pathogen interactions. Nat. Rev. Microbiol. 18, 415–427. doi: 10.1038/s41579-020-0361-8
Toussaint, V., Benoit, D. L., and Carisse, O. (2012). Potential of weed species to serve as a reservoir for Xanthomonas campestris pv. vitians, the causal agent of bacterial leaf spot of lettuce. Crop Prot. 41, 64–70. doi: 10.1016/j.cropro.2012.05.018
Vauterin, L., Hoste, B., Kersters, K., and Swings, J. (1995). Reclassification of Xanthomonas. Int. J. Syst. Bacteriol. 45, 472–489. doi: 10.1099/00207713-45-3-472
Voronina, M. V., Bugaeva, E. N., Vasiliev, D. M., Kabanova, A. P., Barannik, A. P., Shneider, M. M., et al. (2019). Characterization of Pectobacterium carotovorum subsp. carotovorum bacteriophage PP16 prospective for biocontrol of potato soft rot. Microbiology 88, 451–460. doi: 10.1134/S0026261719040118
Weller, D. M. (2007). Pseudomonas biocontrol agents of soilborne pathogens: Looking back over 30 years. Phytopathology 97, 250–256. doi: 10.1094/PHYTO-97-2-0250
Winsor, G. L., Griffiths, E. J., Lo, R., Dhillon, B. K., Shay, J. A., and Brinkman, F. S. L. (2016). Enhanced annotations and features for comparing thousands of Pseudomonas genomes in the Pseudomonas genome database. Nucleic Acids Res 44, D646–D653. doi: 10.1093/nar/gkv1227
Youard, Z. A., Mislin, G. L. A., Majcherczyk, P. A., Schalk, I. J., and Reimmann, C. (2007). Pseudomonas fluorescens CHA0 produces enantio-pyochelin, the optical antipode of the Pseudomonas aeruginosa siderophore pyochelin. J. Biol. Chem. 282, 35546–35553. doi: 10.1074/jbc.M707039200
Zboralski, A., and Filion, M. (2020). Genetic factors involved in rhizosphere colonization by phytobeneficial Pseudomonas spp. Comput. Struct. Biotechnol. J. 18, 3539–3554. doi: 10.1016/j.csbj.2020.11.025
Zboralski, A., Biessy, A., and Filion, M. (2022). Bridging the gap: Type III secretion systems in plant-beneficial bacteria. Microorganisms 10:187. doi: 10.3390/microorganisms10010187
Zboralski, A., Biessy, A., Savoie, M.-C., Novinscak, A., and Filion, M. (2020). Metabolic and genomic traits of phytobeneficial phenazine-producing Pseudomonas spp. are linked to rhizosphere colonization in Arabidopsis thaliana and Solanum tuberosum. Appl. Environ. Microbiol. 86, e2443–e2419. doi: 10.1128/AEM.02443-19
Keywords: Pseudomonas, Xanthomonas, Pectobacterium, lettuce, PGPR, pathogen, genome, biocontrol
Citation: Zboralski A, Biessy A, Ciotola M, Cadieux M, Albert D, Blom J and Filion M (2022) Harnessing the genomic diversity of Pseudomonas strains against lettuce bacterial pathogens. Front. Microbiol. 13:1038888. doi: 10.3389/fmicb.2022.1038888
Received: 07 September 2022; Accepted: 05 December 2022;
Published: 22 December 2022.
Edited by:
Marco Scortichini, Council for Agricultural and Economics Research (CREA), ItalyReviewed by:
Kanika Bansal, Institute of Microbial Technology (CSIR), IndiaSanjeet Kumar, Max Planck Institute for the Science of Human History (MPI-SHH), Germany
Copyright © 2022 Zboralski, Biessy, Ciotola, Cadieux, Albert, Blom and Filion. This is an open-access article distributed under the terms of the Creative Commons Attribution License (CC BY). The use, distribution or reproduction in other forums is permitted, provided the original author(s) and the copyright owner(s) are credited and that the original publication in this journal is cited, in accordance with accepted academic practice. No use, distribution or reproduction is permitted which does not comply with these terms.
*Correspondence: Martin Filion, ✉ martin.filion@agr.gc.ca
†These authors have contributed equally to this work and share first authorship