Facile MoS2 Growth on Reduced Graphene-Oxide via Liquid Phase Method
- 1Department of Chemical Engineering, Petroleum Institute, Khalifa University of Science and Technology, Abu Dhabi, United Arab Emirates
- 2Department of Materials Science and Engineering, University of Ioannina, Ioannina, Greece
- 3Cambridge Graphene Centre, University of Cambridge, Cambridge, United Kingdom
- 4Department of Physics and Astronomy, Vanderbilt University, Nashville, TN, United States
- 5Materials Science and Technology Division, Oak Ridge National Laboratory, Oak Ridge, TN, United States
- 6Department of Mechanical Engineering and Materials Science, Washington University in St. Louis, St. Louis, MO, United States
- 7Institute of Nanoscience and Nanotechnology, National Centre of Scientific Research Demokritos, Athens, Greece
- 8Center for Nanophase Materials Sciences, Oak Ridge National Laboratory, Oak Ridge, TN, United States
- 9Eyring Materials Center, Arizona State University, Tempe, AZ, United States
- 10Center for Electron Microscopic Research, Korea Basic Science Institute, Daejeon, South Korea
- 11TITAN Cement Company S.A, Attica, Greece
- 12Department of Materials Science and Engineering, National University of Singapore, Singapore, Singapore
Single and few-layers MoS2 were uniformly grown on the surface of chemically reduced graphene oxide (r-GO), via a facile liquid phase approach. The method is based on a simple functionalization of r-GO with oleyl amine which seems to affect significantly the MoS2 way of growth. Scanning-transmission-electron microscopy (STEM) analysis revealed the presence of single-layer MoS2 on the surface of a few-layers r-GO. This result was also confirmed by atomic-force microscopy (AFM) images. X-ray photoemission spectroscopy (XPS) and Raman spectroscopy were used for in-depth structural characterization. Furthermore, we have successfully applied the method to synthesize MoS2 nanocomposites with multi wall carbon nanotubes (CN) and carbon nanofibers (CNF). The results demonstrate clearly the selective MoS2 growth on both carbon-based supports.
Introduction
Due to their unique electronic properties, atomically-thin two-dimensional (2D) materials such as graphene (Geim and Novoselov, 2007), hexagonal boron nitride (h-BN) (Dean et al., 2010) and transition-metal dichalcogenide (Radisavljevic et al., 2011; Lembke and Kis, 2012; Wang et al., 2012) have been attracting increasing attention. Molybdenum disulfide (MoS2) is a layered 2D material that is traditionally used as a solid-state lubricant and is the major industrial catalyst for the hydrodesulfurization process (HDS) (Li et al., 2011; Kong et al., 2013). Recently, MoS2 and hybrid MoS2 materials have been extensively studied as catalysts in the hydrogen evolution reaction (HER) (Liao et al., 2013; Wang et al., 2014; Zheng et al., 2014). In recent years, ultrathin graphene and MoS2 crystals have been obtained by either physical or chemical exfoliation methods (Coleman et al., 2011), while large-scale uniform layers have been grown by chemical-vapor-deposition (CVD) techniques (Chen et al., 2016; Samad et al., 2016; Zobel et al., 2016). Both graphene and MoS2 in monolayer form exhibit unique optical and electrical properties compared with the respective multilayer analogs. MoS2, which is referred to in the literature as the inorganic analog of graphene, is a semiconductor with a large direct band gap (Conley et al., 2013). The presence of a band gap in monolayer MoS2 opens new possibilities in electronic, and photonic applications. Furthermore, the edges of MoS2 monolayers are catalytically active so that increasing the number of such sites is critical for the design of advanced catalysts (Jaramillo et al., 2007).
During the last few years, there has been substantial interest in the literature concerning the synthesis of hybrid materials based on graphene and MoS2. Kun Chang and Weixiang Chen reported the synthesis of single-layer MoS2/graphene dispersed in amorphous carbon and tested its electrochemical performance for lithium batteries (Chang and Chen, 2011). The reaction takes place in water under autoclave conditions at 240°C for 24 h. Molybdenum and sulfur precursors Na2MoO4•2H2O and sulfocarbamide (NH2CSNH2) were used and the final material was annealed at 800°C for 2 h in a H2/N2 atmosphere. Ma et al. reported the synthesis of few-layer MoS2-graphene composites via a cationic surfactant-assisted hydrothermal method followed by a post-annealing step and showed that the electrochemical performance for lithium storage is greatly improved compared to bare MoS2 (Ma et al., 2014). Following a similar experimental procedure in a two-step hydrothermal synthesis, Quanjun Xiang et al. reported the decoration of MoS2/graphene heterostuctures with TiO2 nanoparticles and tested them as non-precious metals photocatalysts for H2 production (Xiang et al., 2012). In a one-step hydrothermal method, MoS2/reduced GO was prepared by (NH4)2MoS4 reduction in N,N'-dimethylformamide (DMF) at 200°C by hydrazine. A transmission-electron-microscopy (TEM) study showed that most of the MoS2 lies flat on the reduced GO sheets, with some possessing-induced folded edges exhibiting parallel lines (Li et al., 2011).
Summarizing the literature concerning the synthesis of MoS2/graphene composite nanomaterials via liquid phase reactions, most of the methodologies involve a hydrothermal process for at least 12 h in the 200–240°C temperature range in one- or two-step procedures. Here we report the synthesis of MoS2/r-GO following a liquid-phase approach. The method is based on the thermolytic decomposition of (NH4)2MoS4 by oleyl amine at 350°C in the presence of chemically-exfoliated reduced graphene oxide (r-GO). The presence of exfoliated r-GO nanosheets in the reaction mixture is crucial, providing a confinement environment for the nucleation and growth of the MoS2. It is shown that r-GO is predominantly decorated by single-layer MoS2 that lies flat on the r-GO sheets. The MoS2/RGO composites are surface functionalized efficiently by oleyl amine molecules, making the material dispersible in non-polar solvents such as toluene and hexane.
Experimental Section
Materials
Ammonium tetrathiomolybdate ((NH4)2MoS4, 99.97%), oleyl amine (90% C-18 content), carbon nanotubes, carbon nanofibers, absolute ethanol and n-hexane were purchased from Sigma-Aldrich and used without any further purification. Graphene oxide was synthesized using a modified Staudenmaier's method (Staudenmaier, 1898; Gengler et al., 2010; Stergiou et al., 2010). Briefly powdered graphite (purum, powder ≤0.2 mm, Fluka) were treated by a 2/1 mixture of concentrated H2SO4 (Riedelde Haën) and HNO3 acid, while cooling in an ice–water bath. Subsequently powdered KClO3 (Fluka) were added to the mixture in small portions under vigorous stirring while cooling in an ice-water bath. The reaction was quenched after 18 h by pouring the mixture into ultrapure water. The oxidation product was washed until the pH reached 6.0, and finally dried at room temperature.
Synthesis of MoS2/Reduced Graphene Oxide
The reduction and functionalization of GO was carried out via a chemical approach. The pristine GO was exfoliated in oleyl amine via probe sonication at room temperature. Subsequently the mixture was heated up to 300°C under H2-Ar reducing atmosphere for 1 h. The oleyl amine functionalized r-GO was separated by centrifuging at 12,000 rpm, washed twice in ethanol in order to remove the excess of oleyl amine, and finally dried at room temperature.
MoS2/r-GO nanocomposites were prepared according to the following procedure. Oleyl-amine-functionalized r-GO (40 mg) was dispersed in 10 ml oleyl amine under probe sonication in an inert atmosphere for 15 min. The mixture was then transferred to a heating plate and 20 mg of (NH4)2MoS4 was dissolved into the mixture under vigorous magnetic stirring. In the beginning, the temperature was raised to 120°C and kept there for 15 min under a high-purity N2 blanket. The reaction finally took place at 350°C for 1 h. After cooling the mixture to room temperature, the composite material was precipitated by adding absolute ethanol and was separated by centrifugation (8,000 RPM, 25 min). The washing/separation procedure was repeated until the complete removal of excess oleyl amine molecules and unreacted components. Finally the composite material was dispersed and stored in toluene.
Characterization
The morphology of the composite MoS2/RGO material was studied by a Nion Ultra STEM 100 aberration-corrected scanning transmission electron microscope (STEM), which is equipped with a Gatan Enfinium spectrometer for Electron Energy Loss Spectroscopy (EELS). The STEM was operated at 60 kV. Additionally, the material was characterized by atomic-force microscopy (AFM), Raman, and X-ray photoelectron spectroscopy (XPS).
XPS measurements were performed under ultrahigh vacuum conditions at a base pressure of 5 × 10−10 mbar in a SPECS GmbH instrument equipped with a monochromatic MgKα source (hν = 1253.6 eV) and a Phoibos-100 hemispherical analyzer. Pulverized samples were dispersed in toluene (1 wt%), and after short sonication and stirring, a minute quantity of the suspensions were drop-cast on evaporated gold films supported on mica substrates and left to dry in air before transferring them to ultrahigh vacuum. The energy resolution was set to 0.3 eV and the photoelectron take-off angle was 45° with respect to the surface normal. Recorded spectra were the average of three scans with energy step set to 0.05 eV and dwell time 1 s. All binding energies were referenced to the Si 2p level at 99.15 eV. Spectral analysis included a Shirley background subtraction and peak deconvolution employing mixed Gaussian–Lorentzian functions, in a least squares curve-fitting program (WinSpec) developed at the Laboratoire Interdisciplinaire de Spectroscopie Electronique, University of Namur, Belgium.
Raman spectra were recorded with a micro-Raman (μ-Raman) Renishaw RM1000 system using a laser diode excitation line at 532 nm in the frequency range of 200–2,000 cm−1. Raman scatter was collected by means of an Olympus optical microscope, equipped with 50× and 100× lenses. Using the 50× lens, the probing spot was about 2 μm in diameter, while the laser was operated at 5 mW unless photodecomposition occurred when the power was decreased. The spectrometer was calibrated by recording the spectrum from a Si sample with characteristic Raman peak at 520.7 cm−1. Raman spectra were obtained from samples in the form of drop casted films onto glass substrates. The reported spectra are an average of 3–5 scans.
AFM images were obtained in tapping mode with a 3D Multimode Nanoscope, using Tap-300G silicon cantilevers with a tip radius <10 nm and a force constant of ≈20–75 N m−1. Samples were deposited onto silicon wafers (P/Bor, single side polished) from dilute dispersions of MoS2/r-GO in toluene by drop casting.
Results and Discussion
The synthetic procedure for the decoration of r-GO with MoS2 is illustrated in Scheme 1. Oleyl-amine-functionalized exfoliated r-GO sheets were dispersed in oleyl amine, providing nucleation centers for the growth of MoS2. Then, the thermolytic decomposition of (NH4)2MoS4 at 350°C resulted in the formation of a single layer of MoS2 on the surface of the r-GO. The XRD patterns of MoS2/r-GO nanocomposite are presented in Figure S1, in the Supplementary Material section. Because of the nanosize regime of the material, the diffraction peaks are quite broad, but it is possible to distinguish four diffraction peaks. The intense broad peak around 2θ = 24° corresponds to the (002) diffraction plane of r-GO, while the other peaks can be assigned to the (101), (103), and (110) lattice planes of MoS2.
The morphology of the MoS2/r-GO nanocomposite was examined by atomic- resolution Scanning Transmission Electron Microscopy (STEM) measurements. In Figure 1, we present Z-contrast images of the MoS2 nanosheets on the few-layer r-GO. It is obvious that the MoS2 layers grow discontinuously on the surface of the r-GO sheets, covering most of their surface by a single- to few-layer MoS2 films. Furthermore, the images confirm the few-layer nature of the r-GO as well as the presence of isolated single atoms, most likely Mo atoms, and Mo vacancies. The STEM observations indicate very clearly that the oleyl-amine-functionalized r-GO sheets could be an excellent substrate for the nucleation and growth of MoS2. This effect can be attributed to the presence of oxygen-containing functional groups on the surface of the r-GO or on the weak van der Waals forces (Shi et al., 2012) between the two layered materials, which accommodate the relatively large lattice mismatch between MoS2 and graphene (~28%) (Ma et al., 2011).
In order to clarify the role of the r-GO surface functionalization on the growth of the MoS2 sheets, the synthesis was also done following a similar methodology using GO as staring material, without any previous reduction and surface functionalization. The TEM images, presented in the Supplementary Material section (Figure S2), clearly show that mainly isolated MoS2 nanosheets grow on the GO surface, but they do not lie flat on the GO sheets. Furthermore, the formation of nanoparticles instead of nanosheets can be observed. This morphology is quite similar with a previously reported work in which the MoS2/r-GO composites synthesized by mixing GO with (NH4)2MoS4 in an alcohol/water medium followed by the (NH4)2MoS4 decomposition by HCl acid addition (Koroteev et al., 2011). The above findings prove indirectly that the surface chemistry of the carbon material plays a very important role in the growth of MoS2. The oxygen-containing functional groups, as already reported, affect the nucleation and growth of the MoS2 nanosheets, but on the other hand probably suppress the van der Waals forces that are responsible for the flat MoS2 growth. Finally, STEM-EELS mapping of sulfur and carbon (Figure 2b, which corresponds to the white square area of Figure 2a) shows the distribution of MoS2 on the surface of the r-GO in the nanocomposite material.
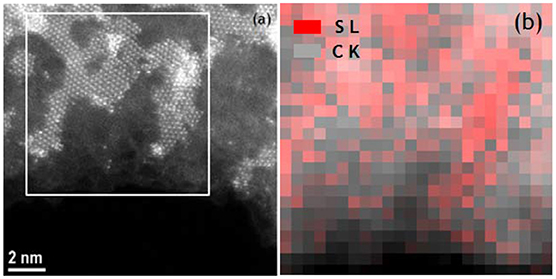
Figure 2. Z-contrast STEM image (a) and EELS sulfur and carbon mapping (b) of MoS2-decorated few layer r-GO. (b) corresponds to the white box area of (a).
The heterostructural nature of the MoS2/r-GO nanocomposite was additionally identified by AFM. In Figure 3, we present the height and phase images (measured in tapping mode AFM) of MoS2 nanosheets grown on few-layer r-GO. The AFM images of the MoS2/r-GO nanocomposite deposited on a Si-wafer show the successful growth of MoS2 layers on the graphene surface (Figure 3). The analysis of the height section profile (Figure 4) showed that the average thickness of the MoS2 flakes on the r-GO sheets is around 4–8 nm, which corresponds to a few MoS2 layers (~5–10). Furthermore, the height profile analysis obtained at the edges of the r-GO sheets, showed a 1.5 nm thickness, a value typically reported for very few r-GO layers as also demonstrated from phase images.
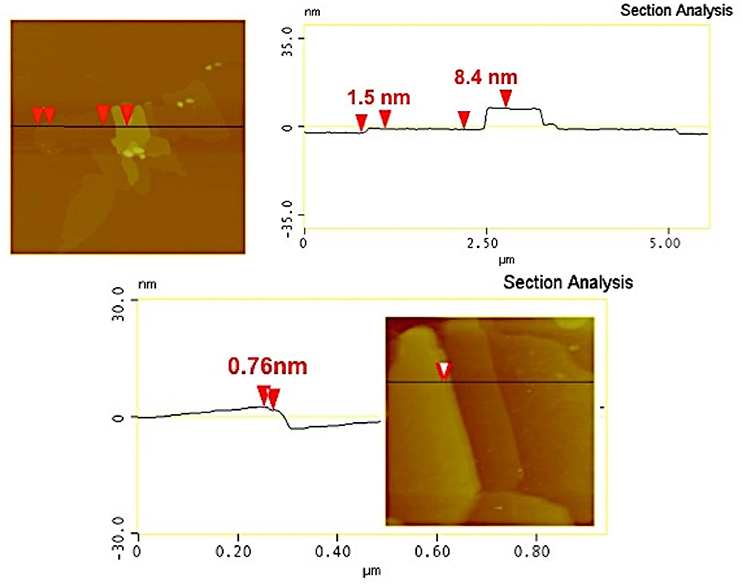
Figure 4. AFM cross-section analysis of MoS2/r-GO nanocomposite (a single MoS2 layer can be distinguished from a package of few-layer MoS2 sheets; picture below).
XPS measurements were used in order to confirm the successful formation of MoS2 on the r-GO surface. C1s (a), Mo3d (b), and S2p (c) XPS spectra of MoS2/r-GO sample are shown in Figure 5. After deconvolution with mixed Gaussian–Lorentzian functions, the C1s spectrum consists of five components. The main peak at a binding energy of 284.6 eV, representing 53.1% of the total C1s area, is attributed to the C-C and C-H bonds. The component at 285.4 eV, contributing a 32.7% to the total C1s signal, is assigned to the hydroxyl C-O bonds as well as the C-N bonds from the oleyl amine. The third peak at 286.2 eV (9.0%) originates from the C-O-C epoxide/ether groups, whereas carbonyl functional groups (C = O) are associated with the peak at 287.3 eV (3.5%). Finally, the higher binding energy contribution recorded at 288.8 eV (1.7%) corresponds to the carboxyl groups (O-C=O) (Dimos et al., 2017; Kouloumpis et al., 2017; Tzitzios et al., 2017).
The domination of the C-C peak along with the high carbon content (80.8%) point out the presence of a r-GO substrate indicating the successful reduction of the starting GO. Due to the low concentration of the functionalizing oleyl amine and accordingly the even lower relative nitrogen atomic concentration, nitrogen and consequently oleyl amine were not detected by XPS. Nevertheless, its presence is verified by FTIR spectroscopy (see Figure S3), which is more effective for distinguishing organic molecules. The presence of oleyl amine in the r-GO and MoS2/r-GO materials was indicated from the characteristic absorptions of the aliphatic and the amine groups in the IR spectra. The vibrations at 2,920 and 2,851 cm−1 are due to the symmetric and asymmetric stretching modes of CH2, the weak band at 2962 arises from the asymmetric C-H stretching mode of the terminal CH3 groups, while the double broad bands around 3,240–3,340 cm−1 arise from the stretching vibrations of the NH2 group. The absence of these characteristic vibrations in the GO spectrum indirectly confirms the successful functionalization by the oleyl amine molecules. Furthermore, the absence of shifting of the N-H stretching band (around 3,300 cm−1 in pure oleyl amine), to lower wavenumbers indicates that the interaction of the NH2 groups on the surface of both r-GO and MoS2/r-GO is weak. On the other hand, molybdenum and sulfur were detected with an atomic concentration of 0.8 and 2.1% respectively proving the development of MoS2 layers on the composite materials as simultaneously the S/Mo ratio is close to 2. In fact, from the distinction of the Mo and S species further conclusions can be deduced.
More specifically, as observed in Figure 5b of the Mo3d energy region, the spectrum consists of two Mo components. From their binding energies (229.4 and 232.4 eV), these peaks are ascribed as MoIV 3d5/2 and MoIV 3d3/2, respectively, and are correlated with MoS2 structures (Spevack and McIntyre, 1993; Merki et al., 2011; Kibsgaard et al., 2012; Zheng et al., 2014). No peaks or shoulders are detected at higher binding energies, indicating the lack of higher oxidation states (+5 or +6) which are possible for Mo and suggesting the growth of further MoxSy species except MoS2 (Spevack and McIntyre, 1993; Merki et al., 2011; Kibsgaard et al., 2012; Zheng et al., 2014). Additionally, the peak at 227.0 eV is defined as S2s whilst more information about sulfur is obtained by the S2p XPS spectrum at Figure 5c. As in the case of Mo, only two peaks are observed; a main peak at 162.3 eV and a shoulder at 163.7 eV, associated with the doublet from S2− 2p3/2 and S2− 2p1/2 respectively (Spevack and McIntyre, 1993; Merki et al., 2011; Kibsgaard et al., 2012; Zheng et al., 2014). Again, no extra peaks are detected affirming that no or other species are present (Spevack and McIntyre, 1993; Merki et al., 2011; Kibsgaard et al., 2012; Zheng et al., 2014). In conclusion, the XPS study confirms the successful growth of MoS2 structures on the composite material with a high content of 10.5 wt% (as deduced from the quantitative XPS analysis), excluding the creation of further MoxSy species.
In addition, Raman spectroscopy is a powerful technique for both r-GO and MoS2 materials, since it provides crucial information about the hybridization of atoms and the vibrational modes. As seen in the spectrum of the composite MoS2/r-GO material (Figure 6), both r-GO-originating G- and D-bands are present as expected. The G-band at 1,594 cm−1 is associated with sp2 hybridized carbon atoms, while the D-band at 1,345 cm−1 originates from the breathing modes of six-atom rings, requiring a defect for its activation, hence is correlated with lattice defects in the case of r-GO. Thus, the ratio of the D- to G-band intensities (ID/IG) states the defects ratio of the r-GO layers, (Tzitzios et al., 2017) and is equal to 0.86. A value slightly lower, as expected, than the one observed for starting GO (see Figure S4). Moreover, peaks at the lower region of Raman shift are attributed to the formed MoS2 layers. This region of the spectrum is multiplied 4 times compared to the rest spectrum in Figure 6, in order to have a clear view of the area. The peak at 407 cm−1 is assigned to the A1g vibrational mode of MoS2, while the one at 375 cm−1 originates from the corresponding mode (Lee et al., 2010; Kibsgaard et al., 2012; Sundaram et al., 2013; Zhang et al., 2013, 2015; David et al., 2014).
The distance between these two peaks has been correlated in the literature with the number of MoS2 layers (Lee et al., 2010; Sundaram et al., 2013; Zhang et al., 2015). Unfortunately in our case, we cannot support the formation of single layers as witnessed in TEM. This is because multilayers are also formed nearby and since the probing spot in Raman measurements was about 2 μm in diameter it was not possible to focus in a region with exclusively single layers of MoS2. Nonetheless, the presence of both MoS2 and r-GO peaks testify to the successful development of the composite material.
In order to study the generality of the methodology carbon nanotubes and carbon fibers were also used as support for the growth of MoS2 layers. Both carbon nanotubes (CN), and carbon nanofibers (CNF), were first functionalized with oleyl amine followed by the growth of the MoS2 using the same procedure. In Figure 7, we present STEM images of the MoS2/CN composites. The images show similar behavior with the r-GO composites, i.e., the MoS2 layers grow flat on the surface of the carbon nanotubes. It is worth mentioning here that, in the CN composites, most of the MoS2 layers are single-layer, suggesting that the sp2 nature of carbon is crucial for the uniform and single-layer growth of the MoS2 on the surface of carbon-based materials. Atomic-resolution Z-contrast STEM images (Figure 7e) reveal the presence of Mo vacancies in the MoS2 monolayers. The images of the corresponding composites with CNF are presented in the Supplementary Material section (Figure S5), and show clearly the uniform growth of mainly monolayer MoS2 on the CNF surfaces.
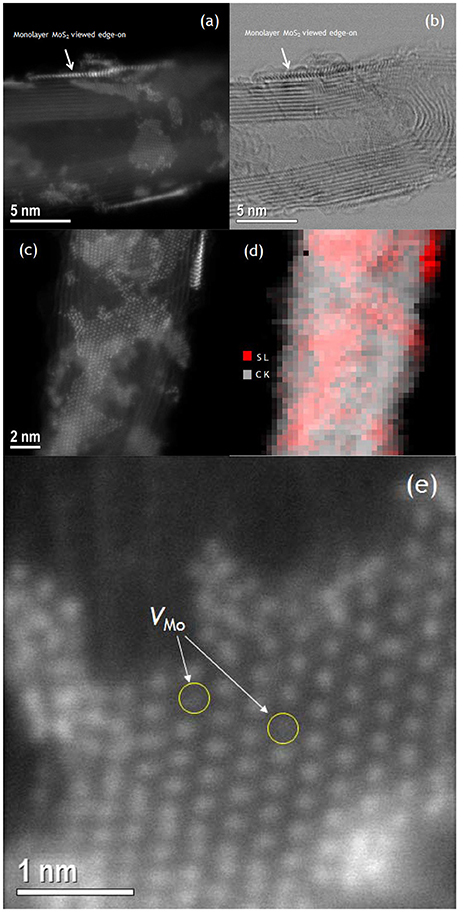
Figure 7. Z-contrast STEM images (a,c), bright field image (b) and EELS sulfur and carbon elemental mapping (d), (with red and gray colors representing sulfur and carbon, respectively), of MoS2/CN composites. Z-contrast image of monolayer MoS2 on CN showing individual Mo vacancies (e).
In conclusion, in this work we report a facile liquid phase chemical approach for the synthesis of hybrid MoS2/r-GO without autoclave conditions. The method is based on the thermolytic decomposition of (NH4)2MoS4 inorganic salt in hot oleyl amine in the presence of chemically exfoliated r-GO. The r-GO sheets work as nucleation centers for the growth of MoS2 and the composite material is composed mainly of single- to few-layer MoS2 on few-layer r-GO. The use of oleyl amine functionalized r-GO as starting material for the growth of the MoS2 seems to be crucial for the flat growth of MoS2. In contrast, when GO is used as starting material, the MoS2 layers do not grow flat. To our knowledge, it is the first time that MoS2/r-GO is synthesized in non-polar media without autoclave conditions and the final hybrid nanocomposites are well dispersible in non-polar solvents such as n-hexane. The methodology is generally applicable and works in a similar way in the case of MoS2 growth on the surface of other carbon-based materials such as multiwall carbon nanotubes (CN) and carbon nanofibers (CNF). This kind of colloidal dispersion can be applicable in the formation of films through inkjet printing techniques (Arapov et al., 2014).
Author Contributions
VT developed the synthesis concept, synthesized the materials and with SA, and GP prepared the manuscript; KD contributed to the manuscript preparation, and with AK, DG, and MAK performed the AFM, XPS and Raman spectroscopy studies; GB, YA, HJ, MK, and MF carried out the structural and the surface functionalization studies; RM, MR, NB, J-CI, SJP, AB, and STP carried out the STEM studies. All authors discussed the results and commented on the manuscript.
Conflict of Interest Statement
The authors declare that the research was conducted in the absence of any commercial or financial relationships that could be construed as a potential conflict of interest.
Acknowledgments
This work is partially funded by the Petroleum Institute (a part of Khalifa University of Science and Technology). Electron microscopy work at Oak Ridge National Laboratory (ORNL) was supported by the U.S. Department of Energy (DOE) Office of Science, Basic Energy Sciences (BES), Materials Science and Engineering Directorate (MR) and through a user project conducted at the Center for Nanophase Materials Sciences, which is a DOE Office of Science User Facility (J-CI, RM).Work at Vanderbilt University was supported by the U. S. Department of Energy grant DE-FG02-09ER-46554 (RM, STP) and the McMinn Endowment.
Supplementary Material
The Supplementary Material for this article can be found online at: https://www.frontiersin.org/articles/10.3389/fmats.2018.00029/full#supplementary-material
References
Arapov, K., Abbel, R., de With, G., and Friedrich, H. (2014). Inkjet printing of graphene. Faraday Discuss. 173, 323–336. doi: 10.1039/C4FD00067F
Chang, K., and Chen, W. (2011). Single-layer MoS2/graphene dispersed in amorphous carbon: towards high electrochemical performances in rechargeable lithium ion batteries. J. Mater. Chem. 21:17175. doi: 10.1039/c1jm12942b
Chen, J., Tang, W., Tian, B., Liu, B., Zhao, X., Liu, Y., et al. (2016). Chemical vapor deposition of high-quality large-sized MoS2 crystals on silicon dioxide substrates. Adv. Sci. 3:1500033. doi: 10.1002/advs.201600033
Coleman, J. N., Lotya, M., O'Neill, A., Bergin, S. D., King, P. J., Khan, U., et al. (2011). Two-dimensional nanosheets produced by liquid exfoliation of layered materials. Science 331, 568–571. doi: 10.1126/science.1194975
Conley, H. J., Wang, B., Ziegler, J. I., Haglund, R. F., Pantelides, S. T., and Bolotin, K. I. (2013). Bandgap engineering of strained monolayer and bilayer MoS2. Nano Lett. 13, 3626–3630. doi: 10.1021/nl4014748
David, L., Bhandavat, R., and Singh, G. (2014). MoS2/graphene composite paper for sodium-ion battery electrodes. ACS Nano 8, 1759–1770. doi: 10.1021/nn406156b
Dean, C. R., Young, A. F., Meric, I., Lee, C., Wang, L., Sorgenfrei, S., et al. (2010). Boron nitride substrates for high-quality graphene electronics. Nat. Nanotechnol. 5, 722–726. doi: 10.1038/nnano.2010.172
Dimos, K., Arcudi, F., Kouloumpis, A., Koutselas, I. B., Rudolf, P., Gournis, D., et al. (2017). Top-down and bottom-up approaches to transparent, flexible and luminescent nitrogen-doped carbon nanodot-clay hybrid films. Nanoscale 9, 10256–10262. doi: 10.1039/C7NR02673K
Geim, A. K., and Novoselov, K. S. (2007). The rise of graphene. Nat. Mater. 6, 183–191. doi: 10.1038/nmat1849
Gengler, R. Y., Veligura, A., Enotiadis, A., Diamanti, E. K., Gournis, D., Józsa, C., et al. (2010). Large-yield preparation of high-electronic-quality graphene by a langmuir–schaefer approach. Small 6, 35–39. doi: 10.1002/smll.200901120
Jaramillo, T. F., Jorgensen, K. P., Bonde, J., Nielsen, J. H., Horch, S., and Chorkendorff, I. (2007). Identification of active edge sites for electrochemical H2 evolution from MoS2 nanocatalysts. Science 317, 100–102. doi: 10.1126/science.1141483
Kibsgaard, J., Chen, Z., Reinecke, B. N., and Jaramillo, T. F. (2012). Engineering the surface structure of MoS2 to preferentially expose active edge sites for electrocatalysis. Nat. Mater. 11, 963–969. doi: 10.1038/nmat3439
Kong, D., Wang, H., Cha, J. J., Pasta, M., Koski, K. J., Yao, J., et al. (2013). Synthesis of MoS2 and MoSe2 films with vertically aligned layers. Nano Lett. 13, 1341–1347. doi: 10.1021/nl400258t
Koroteev, V. O., Bulusheva, L. G., Okotrub, A. V., Yudanov, N. F., and Vyalikh, D. V. (2011). Formation of MoS2 nanoparticles on the surface of reduced graphite oxide. Phys. Status Solidi 248, 2740–2743. doi: 10.1002/pssb.201100123
Kouloumpis, A., Thomou, E., Chalmpes, N., Dimos, K., Spyrou, K., Bourlinos, A. B., et al. (2017). Graphene/carbon dot hybrid thin films prepared by a modified langmuir–schaefer method. ACS Omega 2, 2090–2099. doi: 10.1021/acsomega.7b00107
Lee, C., Yan, H., Brus, L. E., Heinz, T. F., Hone, J., and Ryu, S. (2010). Anomalous lattice vibrations of single- and few-layer MoS2. ACS Nano 4, 2695–2700. doi: 10.1021/nn1003937
Lembke, D., and Kis, A. (2012). Breakdown of high-performance monolayer MoS2 transistors. ACS Nano 6, 10070–10075. doi: 10.1021/nn303772b
Li, Y., Wang, H., Xie, L., Liang, Y., Hong, G., and Dai, H. (2011). MoS2 Nanoparticles grown on graphene: an advanced catalyst for the hydrogen evolution reaction. J. Am. Chem. Soc. 133, 7296–7299. doi: 10.1021/ja201269b
Liao, L., Zhu, J., Bian, X., Zhu, J., Scanlon, M. D., Girault, H. H., et al. (2013). MoS2 formed on mesoporous graphene as a highly active catalyst for hydrogen evolution. Adv. Funct. Mater. 23, 5326–5333. doi: 10.1002/adfm.201300318
Ma, L., Huang, G., Chen, W., Wang, Z., Ye, J., Li, H., et al. (2014). Cationic surfactant-assisted hydrothermal synthesis of few-layer molybdenum disulfide/graphene composites: microstructure and electrochemical lithium storage. J. Power Sources 264, 262–271. doi: 10.1016/j.jpowsour.2014.04.084
Ma, Y., Dai, Y., Guo, M., Niu, C., and Huang, B. (2011). Graphene adhesion on MoS2 monolayer: an ab initio study. Nanoscale 3, 3883–3887. doi: 10.1039/c1nr10577a
Merki, D., Fierro, S., Vrubel, H., and Hu, X. (2011). Amorphous molybdenum sulfide films as catalysts for electrochemical hydrogen production in water. Chem. Sci. 2, 1262–1267. doi: 10.1039/C1SC00117E
Radisavljevic, B., Radenovic, A., Brivio, J., Giacometti, V., and Kis, A. (2011). Single-layer MoS2 transistors. Nat. Nanotechnol. 6, 147–150. doi: 10.1038/nnano.2010.279
Samad, L., Bladow, S. M., Ding, Q., Zhuo, J., Jacobberger, R. M., Arnold, M. S., et al. (2016). Layer-controlled chemical vapor deposition growth of MoS2 vertical heterostructures via van der waals epitaxy. ACS Nano 10, 7039–7046. doi: 10.1021/acsnano.6b03112
Shi, Y., Zhou, W., Lu, A.-Y., Fang, W., Lee, Y.-H., Hsu, A. L., et al. (2012). van der Waals epitaxy of MoS2 layers using graphene as growth templates. Nano Lett. 12, 2784–2791. doi: 10.1021/nl204562j
Spevack, P. A., and McIntyre, N. S. (1993). A Raman and XPS investigation of supported molybdenum oxide thin films. 2. Reactions with hydrogen sulfide. J. Phys. Chem. 97, 11031–11036. doi: 10.1021/j100144a021
Staudenmaier, L. (1898). Verfahren zur Darstellung der Graphitsäure. Berichte der Deutschen Chemischen Gesellschaft 31, 1481–1487. doi: 10.1002/cber.18980310237
Stergiou, D. V., Diamanti, E. K., Gournis, D., and Prodromidis, M. I. (2010). Comparative study of different types of graphenes as electrocatalysts for ascorbic acid. Electrochem. Commun. 12, 1307–1309. doi: 10.1016/j.elecom.2010.07.006
Sundaram, R. S., Engel, M., Lombardo, A., Krupke, R., Ferrari, A. C., Avouris, P., et al. (2013). Electroluminescence in Single Layer MoS2. Nano Lett. 13, 1416–1421. doi: 10.1021/nl400516a
Tzitzios, V., Hu, X., Dimos, K., Gournis, D., Georgakilas, V., Avgouropoulos, G., et al. (2017). Uniform growth of fct FePt nanoparticles on the surface of reduced-GO via a green facile approach. Ferromagnetic r-GO nanocomposites with high coercivity and surface area. Carbon 121, 209–216. doi: 10.1016/j.carbon.2017.05.077
Wang, H., Lu, Z., Kong, D., Sun, J., Hymel, T. M., and Cui, Y. (2014). Electrochemical tuning of MoS2 nanoparticles on three-dimensional substrate for efficient hydrogen evolution. ACS Nano 8, 4940–4947. doi: 10.1021/nn500959v
Wang, H., Yu, L., Lee, Y.-H., Shi, Y., Hsu, A., Chin, M., et al. (2012). Integrated circuits based on bilayer MoS2 transistors. Nano Lett. 12, 4674–4680. doi: 10.1021/nl302015v
Xiang, Q., Yu, J., and Jaroniec, M. (2012). Synergetic effect of MoS2 and graphene as cocatalysts for enhanced photocatalytic H2 production activity of TiO2 nanoparticles. J. Am. Chem. Soc. 134, 6575–6578. doi: 10.1021/ja302846n
Zhang, W., Chuu, C. P., Huang, J. K., Chen, C. H., Tsai, M. L., Chang, Y. H., et al.(2015). Ultrahigh-gain photodetectors based on atomically thin graphene-MoS2 heterostructures. Sci. Rep. 4:3826. doi: 10.1038/srep03826
Zhang, X., Han, W. P., Wu, J. B., Milana, S., Lu, Y., Li, Q. Q., et al. (2013). Raman spectroscopy of shear and layer breathing modes in multilayer MoS2 Phys. Rev. B 87, 1154131–1154138. doi: 10.1103/PhysRevB.87.115413
Zheng, X., Xu, J., Yan, K., Wang, H., Wang, Z., and Yang, S. (2014). Space-confined growth of MoS2 nanosheets within graphite: the layered hybrid of MoS2 and graphene as an active catalyst for hydrogen evolution reaction. Chem. Mater. 26, 2344–2353. doi: 10.1021/cm500347r
Keywords: reduced graphene oxide, MoS2, hybrid, layered materials, colloidal solutions, chemical synthesis
Citation: Tzitzios V, Dimos K, Alhassan SM, Mishra R, Kouloumpis A, Gournis D, Boukos N, Roldan MA, Idrobo J-C, Karakassides MA, Basina G, Alwahedi Y, Jin Kim H, Katsiotis MS, Fardis M, Borisevich A, Pennycook SJ, Pantelides ST and Papavassiliou G (2018) Facile MoS2 Growth on Reduced Graphene-Oxide via Liquid Phase Method. Front. Mater. 5:29. doi: 10.3389/fmats.2018.00029
Received: 09 February 2018; Accepted: 24 April 2018;
Published: 02 July 2018.
Edited by:
Wee-Jun Ong, Institute of Materials Research and Engineering (A*STAR), SingaporeReviewed by:
Jialiang Wang, University of Wisconsin-Madison, United StatesKazuma Gotoh, Okayama University, Japan
Copyright © 2018 Tzitzios, Dimos, Alhassan, Mishra, Kouloumpis, Gournis, Boukos, Roldan, Idrobo, Karakassides, Basina, Alwahedi, Jin Kim, Katsiotis, Fardis, Borisevich, Pennycook, Pantelides and Papavassiliou. This is an open-access article distributed under the terms of the Creative Commons Attribution License (CC BY). The use, distribution or reproduction in other forums is permitted, provided the original author(s) and the copyright owner(s) are credited and that the original publication in this journal is cited, in accordance with accepted academic practice. No use, distribution or reproduction is permitted which does not comply with these terms.
*Correspondence: Vasileios Tzitzios, vatzitzios@pi.ac.ae
Saeed M. Alhassan, salhassan@pi.ac.ae
George Papavassiliou, g.papavassiliou@inn.demokritos.gr