- 1Department of Pathobiology, Institute of Immunology, University of Veterinary Medicine, Vienna, Austria
- 2dsm-firmenich, Animal Nutrition and Health R&D Center, Tulln, Austria
Introduction: The mycotoxins deoxynivalenol (DON) and zearalenone (ZEN), produced by Fusarium fungi, are frequently found in the cereal-rich diet of pigs and can modulate the immune system. Some enzymes or bacteria present in the digestive tract can de-epoxydize DON to deepoxy-deoxynivalenol (DOM-1) and biotransform ZEN into hydrolyzed ZEN (HZEN). The effects of these metabolites on immune cells, particularly with respect to the vaccine responses, are poorly documented. The aim of this study was to address the impact of DON and ZEN and their respective derivatives, on proliferation, and antibody production of porcine B cells in vitro.
Methods: Peripheral blood mononuclear cells (PBMCs), isolated from healthy pigs, were stimulated with the Toll-like receptor (TLR) 7/8-agonist Resiquimod (R848) or the TLR/1/2-agonist Pam3Cys-SKKKK in combination with DON [0.1-1.6 µM] or DOM-1 [1.6 µM and 16 µM] and ZEN [2.5-40 µM] or HZEN [40 µM].
Results: A strong decrease in B-cell proliferation was observed at DON concentrations equal to or exceeding 0.8 µM and at ZEN concentrations equal to or exceeding 20 µM. Treatment with 1.6 µM DON or 40 µM ZEN led to almost a complete loss of live CD79α+ B cells. Moreover, CD21 expression of proliferating IgG+ and IgM+ B-cell subsets was decreased at DON concentrations equal to and exceeding 0.4 µM and at ZEN concentrations equal to or exceeding 10 µM. ELISpot assays revealed a decrease of IgG-secreting B cells at concentrations of and exceeding 0.4 µM and at ZEN concentrations equal to and exceeding 10 µM. ELISA assays showed a decrease of IgM, IgG, and IgA secretion at concentrations equal to or exceeding 0.4 µM DON. ZEN reduced IgM secretion at 20-40 µM (both R848 and Pam3Cys-SKKKK), IgG secretion at 40 µM (both R848 and Pam3Cys-SKKKK) and IgA secretion at 20-40 µM.
Discussion: Our in vitro experiments show that while DON and ZEN impair immunoglobulin production and B-cell proliferation, this effect is abrogated by HZEN and DOM-1.
1 Introduction
As the most frequently occurring natural contaminants of food and feed, mycotoxins pose significant health threats to both humans and animals. A worldwide survey has confirmed mycotoxin contamination in more than 70% of agricultural commodities (1). Particularly prominent among the array of fungal metabolites are the fusariotoxins deoxynivalenol (DON) and zearalenone (ZEN) (2, 3), which do not only occur separately, but have recently been reported to frequently co-occur in feedstuffs and in complete feed for pigs (4, 5).
Depending on the severity and duration of exposure, the trichothecene DON can inflict acute (e.g. diarrhea, emesis, leucocytosis, haemorrhage, endotoxemia) as well as chronic (growth retardation, immunosuppression) toxicological effects (6). Its interaction with the peptidyl transferase center of the 60S ribosomal subunit induces a “ribotoxic stress” response, leading to the inhibition of elongation during protein synthesis. This causes the activation of mitogen-activated protein kinases (MAPKs), resulting in oxidative stress, local inflammation, and modulation of the immune response (6–8). ZEN on the other hand, is primarily known for its toxicity on the reproductive system, which is based on its structural similarity to estrogen, enabling it to induce activation of estrogen receptors α (ERα) and β (ERβ) (9). While in vitro studies confirm ZEN-induced proliferation of estrogen-dependent cells (e.g. MCF-7) (10), in vivo investigations report hyperestrogenism and severe reproductive disorders (e.g. compromised fertility, abnormal fetal development, swelling/reddening of the vulva, metaplasia of the uterus) following exposure to the mycotoxin (2, 11, 12). However, toxicology of ZEN goes far beyond the latter effects (2, 11, 13, 14), ranging from histopathological alterations with subsequent development of liver cancer (15, 16), to hematotoxic impacts (disruption of coagulation, modification of blood parameters) (15, 17, 18), to genotoxic effects (in vitro formation of DNA adducts, DNA fragmentation, micronucleus formation, chromosomal aberration, cell proliferation, and cell apoptosis) (15, 18).
Although the toxicological profiles of DON and ZEN are characterized in various studies, their effects on immune cells, particularly with respect to vaccine-related immune responses, has not been investigated in detail. Available in vitro investigations with porcine lymphocytes and other immune-related cells, confirm that DON impairs critical functions of these cells, including their survival, proliferation, and maturation (19–22). A comparative study of DON and DOM-1 confirmed DON-induced impairment of proliferation in concanavalin A (ConA) stimulated bovine, porcine, and chicken peripheral blood mononuclear cells (PBMCs), as well as a strong DON-induced reduction of CD4+, CD8+, and γδ T cell proliferation (19). Similarly, flow cytometry phenotyping has revealed DON-induced reduction of proliferation of major porcine T-cell subsets (CD4+, CD8+, and γδ T cells) as well as a reduction of the expression of co-stimulatory molecules CD27 and CD28 (21), which are critical for T-cell activation, proliferation, and survival (23, 24). DON has also been reported to modulate the expression of transcription factors and related cytokines, suggesting that for CD4+ and CD8+ T cells in particular, DON can modulate T cell differentiation into a pro-inflammatory type-1 direction, which could be favorable or unfavorable for ongoing immune responses to infection or vaccination (25). Furthermore, Toutounchi et al., 2021 investigated the effects of DON exposure on the development of allergies and vaccine responsiveness in a mouse model. It was shown that exposure to DON during pregnancy and lactation can lead to an imbalanced state of the immune system, resulting in increased allergic immune responses to food allergens and a decreased immune response to vaccination against influenza virus (26).
Like DON, also ZEN has significant effects on immune responses with immunostimulatory or immunosuppressive results (27). Despite the increasing number of studies analyzing ZEN-induced immune modulation, data are fragmentary, particularly with respect to vaccine-related effects. Most available studies confirm a ZEN-mediated reduction of serum IgG and IgM, regardless of the animal species (mice, rats, swine), toxin concentration, or duration of exposure (28–36). It is postulated that ZEN exposure may interfere with the ability to uphold an adequate immune response to vaccination and that the toxin can alter antibody synthesis to vaccine antigens. While some studies report decrease of antibody titer to porcine parvovirus (31) or swine plague vaccination (35) in ZEN-treated animals, more research is required to better understand the effects of ZEN on the underlying vaccine-related immune responses.
Feed additives leading to the biodegradation of DON and ZEN are currently available to reduce bioavailability and/or toxicity of these mycotoxins. DON is biotransformed to DOM-1 via the cleavage of the 12,13-epoxy ring by an epoxidase of the Gen. nov. (formerly Eubacterium) sp. nov. BBSH 797 of the Coriobacteriaceae family, isolated from bovine rumen fluid (37). With respect to ZEN, the bacterial enzyme zearalenone hydrolase (ZenA), applied as a feed additive, degrades ZEN in the gastrointestinal tract (38, 39), releasing hydrolyzed zearalenone (HZEN), which partially decarboxylates spontaneously to decarboxylated hydrolyzed ZEN (DHZEN). For the safe implementation of DOM-1 and HZEN, the continuous assessment of both metabolites is necessary. Concerning DOM-1, a few studies have confirmed its detoxified status compared to DON (19, 40–43). However, with respect to HZEN, only one study has shown a significantly reduced estrogenic activity of HZEN in vitro and in female pigs (44). No studies are yet available focusing on the effects of HZEN on the immune system.
Thus, this study presents the first investigation of the effects of the mycotoxins DON and ZEN as well as their derivatives DOM-1 and HZEN on proliferation and antibody production of porcine B cells. Antibodies, produced by terminally differentiated B cells (plasma cells and plasmablasts), are the most frequent correlate of protection in vaccines (45). Hence, we focused on in vitro experiments investigating the proliferation of B cells and major subsets therein as well as antibody production by ELISA and ELISpot. Particularly with respect to ZEN and HZEN our study delivers much needed information. It does not only provide valuable data regarding the detrimental effects of DON and ZEN on major functional B-cell parameters but also underlines the safety of the biodegradation products DOM-1 and HZEN, both of which did not affect the investigated immune parameters.
2 Material and methods
2.1 Mycotoxins and stimulants
Mycotoxins and their metabolites were supplied by Biopure (Romer Labs®, Tulln, Austria) and had a purity of ≥ 99%. Deoxynivalenol (DON) and deepoxy-deoxynivalenol (DOM-1) were dissolved in sterile water to obtain a 5 mM stock solution. Zearalenone (ZEN) and hydrolyzed-zearalenone (HZEN) were dissolved in sterile water and DMSO (1:1 dilution ratio) to obtain a 5 mM stock solution (46). Stock solutions were stored in aliquots at -20°C.
For B cell stimulation, PBMC cultures were treated either with the Toll-like receptor (TLR) 7/8-agonist Resiquimod (InvivoGen, Toulouse, France) or the TLR 1/2-agonist Pam3Cys-SKKKK (EMC microcollections GmbH, Tübingen, Germany) in the concentrations outlined below.
2.2 Isolation of peripheral blood mononuclear cells
For the isolation of PBMCs, blood of six 6-months-old pigs was obtained from an abattoir, which slaughters animals from different conventional finishing farms. Animals were subjected to electric high voltage anesthesia followed by exsanguination. This procedure is in accordance with the Austrian Animal Welfare Slaughter Regulation. Blood was collected during exsanguination into heparinized sample tubes. PBMCs were isolated by gradient centrifugation (Pancoll human, density: 1.077 g/mL, PAN Biotech, Aidenbach, Germany) and frozen at -150°C for further use.
2.3 Proliferation assays by violet proliferation staining
Prior to in vitro treatment, PBMCs were labelled with the CellTrace™ Violet Cell Proliferation Kit (Thermo Fisher Scientific, Waltham, MA), as described by Reutner et al. (2012) (47). Subsequently, labelled PBMCs [2 × 105/well] were cultivated in cell culture medium (RPMI1640 with stable glutamine [PAN-Biotech], supplemented with 10% [v/v] heat-inactivated fetal calf serum [FCS, Gibco™, Thermo Fisher Scientific]), with or without Pam3Cys-SKKKK [10 µM] or R848 [2.5 µg/ml] stimulation and in the presence or absence of DON [0.1-1.6 µM], DOM-1 [1.6 µM], ZEN [2.5-40 µM], or HZEN [40 µM] for 4 days at 37°C. Per condition, at least four wells were prepared (quadruplicates). Subsequently, wells treated with the same conditions were pooled and centrifuged (350g, 10 minutes room temperature). Supernatants were collected and stored at -20°C until use in ELISA. Cell pellets were resuspended and subjected to B-cell phenotyping and proliferation analysis by flow cytometry (FCM) as outlined below.
2.4 Phenotyping of B cells by FCM
Cells derived from in vitro cultures were labelled with primary antibodies (IgM and IgG) and second step reagents, as listed in Table 1. Cells were stained by incubation with the respective antibodies and second step reagents for 20 minutes at room temperature. Between incubation steps, cells were washed twice with PBS (PAN-Biotech) supplemented with 3% FCS. Prior to the third incubation, cells were washed only with PBS, to allow staining with a live/dead discrimination dye (Fixable Viability dye eFluor780, Thermo Fisher Scientific). In the same step free binding sites of secondary antibodies were blocked with mouse IgG (Jackson Immuno Research, Ely, UK, 1μg per sample). Following this, cells were again washed twice with PBS + 3% FCS and stained for CD21. Prior to the last incubation cells were washed again, subsequently fixed and permeabilized using the BD Cytofix/CytopermTM Fixation/Permeabilization Kit (BD Biosciences, San Jose, CA) according to manufacturer’s instructions. Fixed and permeabilized cells were then stained for intracellular CD79α expression for 30 minutes at 4°C. FCM analyses of stained cells were performed on a FACS Canto II (BD Biosciences, San Jose, CA) flow cytometer equipped with three lasers (405, 488, and 633 nm). Per sample, at least 100,000 lymphocytes were collected, based on FSC/SSC properties. Data analyses of flow cytometric raw data were performed by FACS Diva 6.1.3 and FlowJo version 10.4 (both BD Biosciences). Percentages of live PBMCs were identified by gating on all cellular events, as shown in Supplementary Figure 1). The gating strategies for proliferating B cells and B-cell subsets are also illustrated in Supplementary Figure 1.
2.5 Quantification of antibody secretion by ELISA
Total concentrations of major Ig classes (IgM, IgG, and IgA) in the culture supernatants of treated PBMCs were determined via ELISA (Bethyl, Interchim, Montluçon, France), which was performed according to manufacturer’s instructions and as previously described (48). Optical densities were measured in a Sunrise ELISA reader (Tecan, Crailsheim, Germany) at 450 nm. Standard curves were used to convert the optical densities to cytokine concentrations in the supernatants.
2.6 IgG B-cell ELISpot
ELISpot analysis (ELISpot Flex Porcine IgG [ALP], Mabtech) was performed according to the manufacturer’s instructions and as previously described (49). Briefly, three replicates of 2 × 105 cells/well were stimulated with PAM-3Cys-SKKKK or R848 and treated with DON [0.1-1.6 µM], DOM-1 [1.6 µM, 16 µM], ZEN [2.5-40 µM], or HZEN [40 µM] in cell culture medium (as above) for three days. Following treatment, cells were harvested, washed three times with PBS (PAN-Biotech) and resuspended in cell culture medium. Cells were then counted and transferred to B-cell ELISpot plates [2.5 × 104 cells/well] for an incubation period of 4 hours at 37°C. Subsequently, plates were washed with PBS and subjected to the detection antibody MT424 (Mabtech) for 2 hours at room temperature. Streptavidin-ALP (dilution: 1:2000, Roche, Vienna, Austria) was added for 1 hour at room temperature, followed by a 5-bromo-4-chloro-3-indolyl phosphate/nitro blue tetrazolium substrate, which was left to incubate in the dark for 5 minutes. Spots were counted with an AID ELISpot reader (AID, Strassberg, Germany).
2.7 Statistical analysis
Graphs were created and statistic calculations performed with Graphpad Prism V7.04 (GraphPad Software, San Diego, CA). The data were subjected to a one-way ANOVA followed by a Bonferroni’s multiple comparison test, with a p value < 0.05 considered as significant. In case data was not normally distributed, the Kruskall-Wallis test was applied.
3 Results
3.1 DON and ZEN affect survival of activated PBMCs
Following R848 or Pam3Cys-SKKKK stimulation of PBMCs in the presence or absence of DON [0.1-1.6 µM], DOM-1 [1.6 µM, 16 µM], ZEN [2.5-40 µM], or HZEN [40 µM] for four days, cells were harvested, labelled for B-cell phenotyping and subsequently analyzed by flow cytometry. Based on light scatter properties total intact cells were gated, followed by live cell analysis (Supplementary Figure 2).
Under R848-stimulation, the survival rate of PBMCs (percentage of live cells) was significantly decreased at 1.6 µM DON (p=0.0319) only (Figure 1A). Under Pam3Cys-SKKKK stimulation, the cell survival rate was significantly reduced when cells were subjected to 0.8 µM (p=0.0341) and 1.6 µM (p<0.0001) DON (Figure 1A) or 40 µM ZEN (p=0.001) (Figure 1B). Neither DOM-1 [1.6-16 µM] nor HZEN [40 µM] had any effect on the survival rate of PBMCs, regardless of the stimulus used (Figures 1A, B).
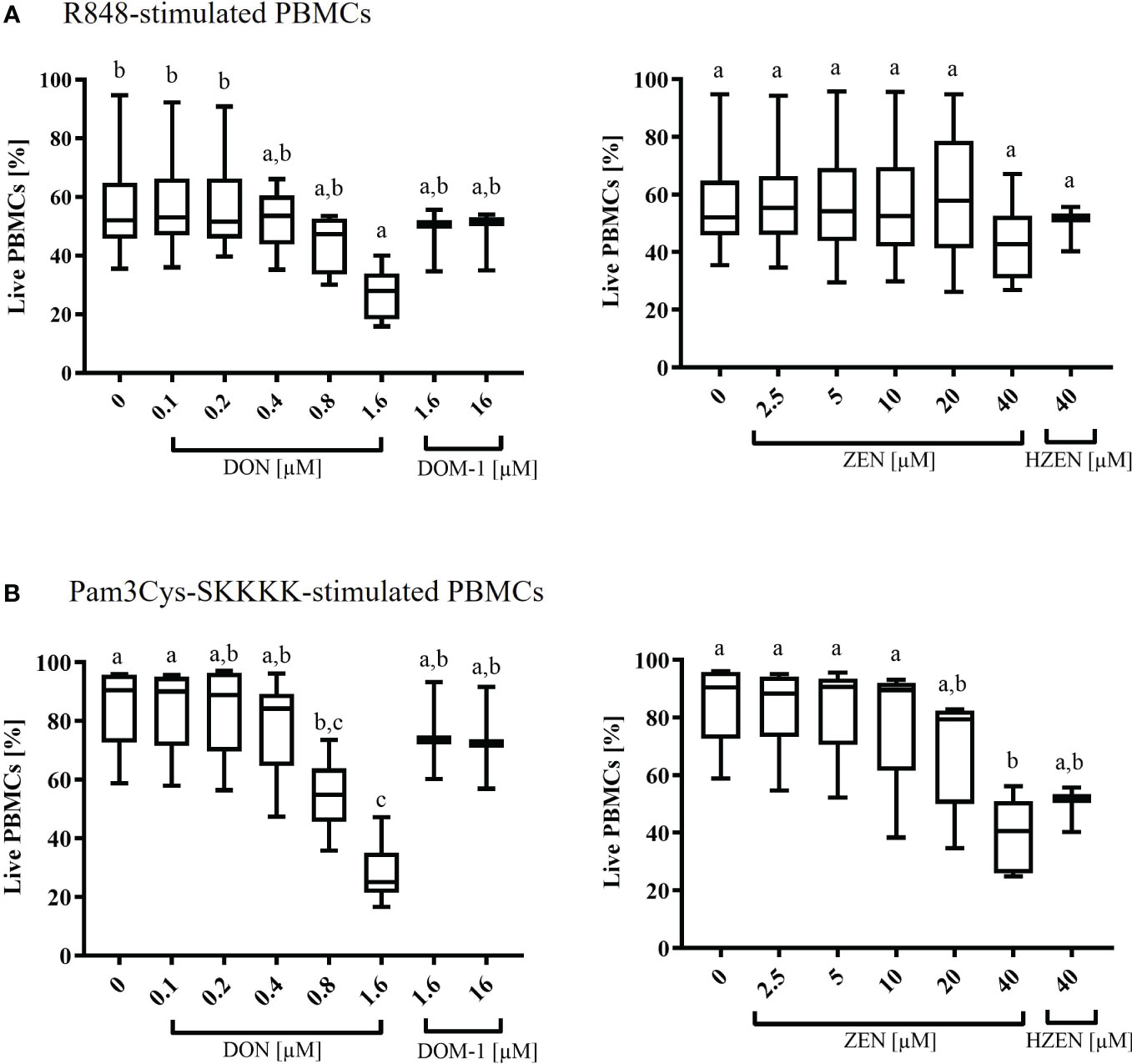
Figure 1 Percentages of live (A) R848- or (B) Pam3Cys-SKKKK-stimulated PBMCs in the presence of deoxynivalenol (DON) and zearalenone (ZEN) and their metabolites deepoxy-deoxynivalenol (DOM-1) and hydrolyzed zearalenone (HZEN). PBMCs stained with a violet proliferation dye were exposed to either of two stimuli (R848 or Pam3Cys-SKKKK) in the absence or presence of DON, DOM-1, ZEN or HZEN for a period of 4 days. Cells were then harvested and analyzed by flow cytometry. Boxplots display the percentage of live R848 or Pam3Cys-SKKKK stimulated PBMCs in presence of different mycotoxins concentrations. Different letters indicate significant differences between treatments (One Way ANOVA, Bonferroni post-hoc test, p<0.05, n=6 for the mycotoxins and n=3 for the metabolites).
The baseline response to DON, DOM-1, ZEN, and HZEN in the absence of a TLR-agonist was also investigated and results are shown in Supplementary Figure 3. Overall, survival rates in non-stimulated cultures were high but significantly reduced in the presence of DON (0.8 µM, 1.6 µM) and ZEN (20 µM, 40 µM). Again, DOM-1 and HZEN had no influence on cell survival.
3.2 DON and ZEN affect proliferation of total B cells and B-cell subsets
To evaluate total B-cell proliferation by FCM, dead cells were excluded as shown in Supplementary Figures 1, 2. This was followed by gating for CD79α+ total B cells and subsequently CD79α+ B cells were analyzed for violet proliferation dye fluorescence (Supplementary Figures 1, 4) to identify total proliferating B cells. Proliferation dye fluorescence profiles were also combined with expression profiles of CD21, IgM or IgG to identify proliferating CD21+, IgM+ or IgG+ within total B cells, respectively (Supplementary Figures 1, 5).
Compared to the control, total B-cell proliferation was significantly reduced in the presence of DON [0.8-1.6 µM] (p<0.0001), regardless of the applied stimulus (Figure 2A). Furthermore, DON-induced proliferation reductions were observed in all R848-stimulated B-cell subsets. DON led to reductions of CD21+ proliferating cells at concentrations of 0.4-1.6 µM (p<0.0001), IgM+ proliferating B cells at 0.8-1.6 µM (p<0.0001), and IgG+ proliferating B cells at 0.8 µM (p=0.0347) and 1.6 µM (p=0.002). Similarly, DON led to significant proliferation reductions in all Pam3Cys-SKKK stimulated B-cell subsets. DON significantly reduced CD21+ proliferating cells at 0.4 µM (p=0.0004), 0.8 µM, and 1.6 µM (both: p<0.0001) as well as IgM+ proliferating cells at 0.8 and 1.6 µM (both: p<0.0001). Regarding IgG+ proliferating cells, a DON induced reduction was observed at 0.8 (p=0.0565) and 1.6 µM (p=0.0029), however significance levels were only reached for the latter concentration. DOM-1 [1.6 and 16 µM] had no reducing effect on total B-cell proliferation or on the proliferation of the different B-cell subsets, regardless of the applied stimulus (Figure 2A).
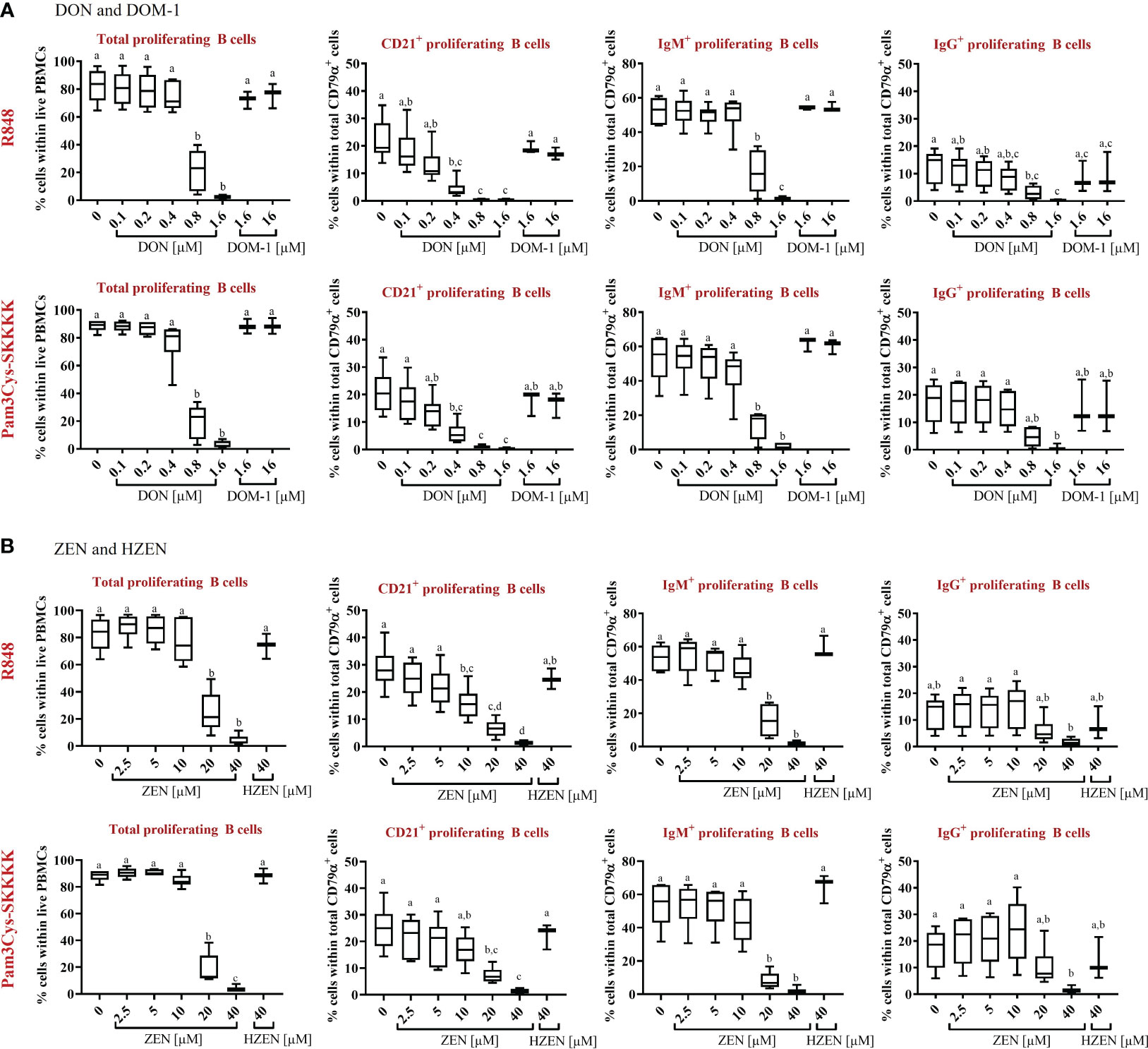
Figure 2 Percentages of total proliferating B cells and major subsets (CD21+, IgM+, and IgG+) in the presence or absence of (A) DON [0.1-1.6 µM] and DOM-1 [1.6 and 16 µM] or (B) ZEN [2.5-40 µM] and HZEN [40 µM] under R848 or Pam3Cys-SKKKK stimulation. Violet proliferation dye-stained PBMCs were stimulated with R848 or Pam3Cys-SKKKK and cultivated in the absence or presence of DON, DOM-1, ZEN, or HZEN for four days. After harvesting, cells were labelled for CD79α, CD21, IgM, and IgG. Gates were applied to identify proliferating total B cells or B-cell subsets within total B cells. Different letters indicate significant differences between treatments (One Way ANOVA, Bonferroni post-hoc test, p<0.05, n=6 for the mycotoxins and n=3 for the metabolites).
With respect to ZEN, total B-cell proliferation was significantly reduced at a concentration of and exceeding 20 µM (p<0.0001), regardless of the stimulus applied (Figure 2B). ZEN-induced proliferation reductions were detected in all R848-stimulated B-cell subsets. ZEN significantly reduced CD21+ proliferating B cells at 10 µM (p=0.0069), 20 µM (p<0.0001) and 40 µM (p<0.0001) and IgM+ proliferating B cells at 20 µM and 40 µM (both: p<0.0001). With respect to IgG+ proliferating B cells, ZEN-induced reductions were observed at 40 µM (p= 0.0586), however significance levels were not reached. Similar effects of ZEN were observed in Pam3Cys-SKKKK-stimulated B cells. ZEN led to significant reductions of CD21+ proliferating cells at 20 µM (p=0.0004) and 40 µM (p<0.0001), IgM+ proliferating cells at 20 and 40 µM (both p<0.0001) and IgG+ proliferating cells at 40 µM (p=0.0446). HZEN (40 µM) had no reducing effect on total B-cell proliferation or on the proliferation of different B-cell subsets, regardless of the applied stimulus (Figure 2B).
The effect of DON, DOM-1, ZEN and HZEN in the absence of TLR-agonists R848 and Pam3CysSKKKK stimulated cells was also investigated (Supplementary Figure 6). The observed effects were similar to those seen in stimulated cells, but on a much lower level since spontaneous proliferation of B cells and B-cell subsets is low. Due to this, significant differences in reduction of B-cell proliferation seen with DON and ZEN in R848 or Pam3Cys-SKKKK stimulated cultures were not always reproducible in non-stimulated cultures.
3.3 DON and ZEN affect the number of IgG-secreting cells
The effects of DON, DOM-1, ZEN, and HZEN on the number of IgG secreting B cells was assessed via ELISpot assays (Figure 3). Spots in such assays indicate that IgG was released and captured by anti-IgG antibodies at this particular spot, indicating the presence of an IgG secreting cell (Supplementary Figure 7). Stimulation of PBMCs with R848 or Pam3Cys-SKKKK led to a significant increase of IgG-secreting cells. DON [0.8-1.6 µM] (Figure 3A) and ZEN [20-40 µM] (Figure 3B) induced a significant decrease of IgG-secreting cells, regardless of the stimulus applied. Neither DOM-1 nor HZEN had any effect on the of IgG secreting cells.
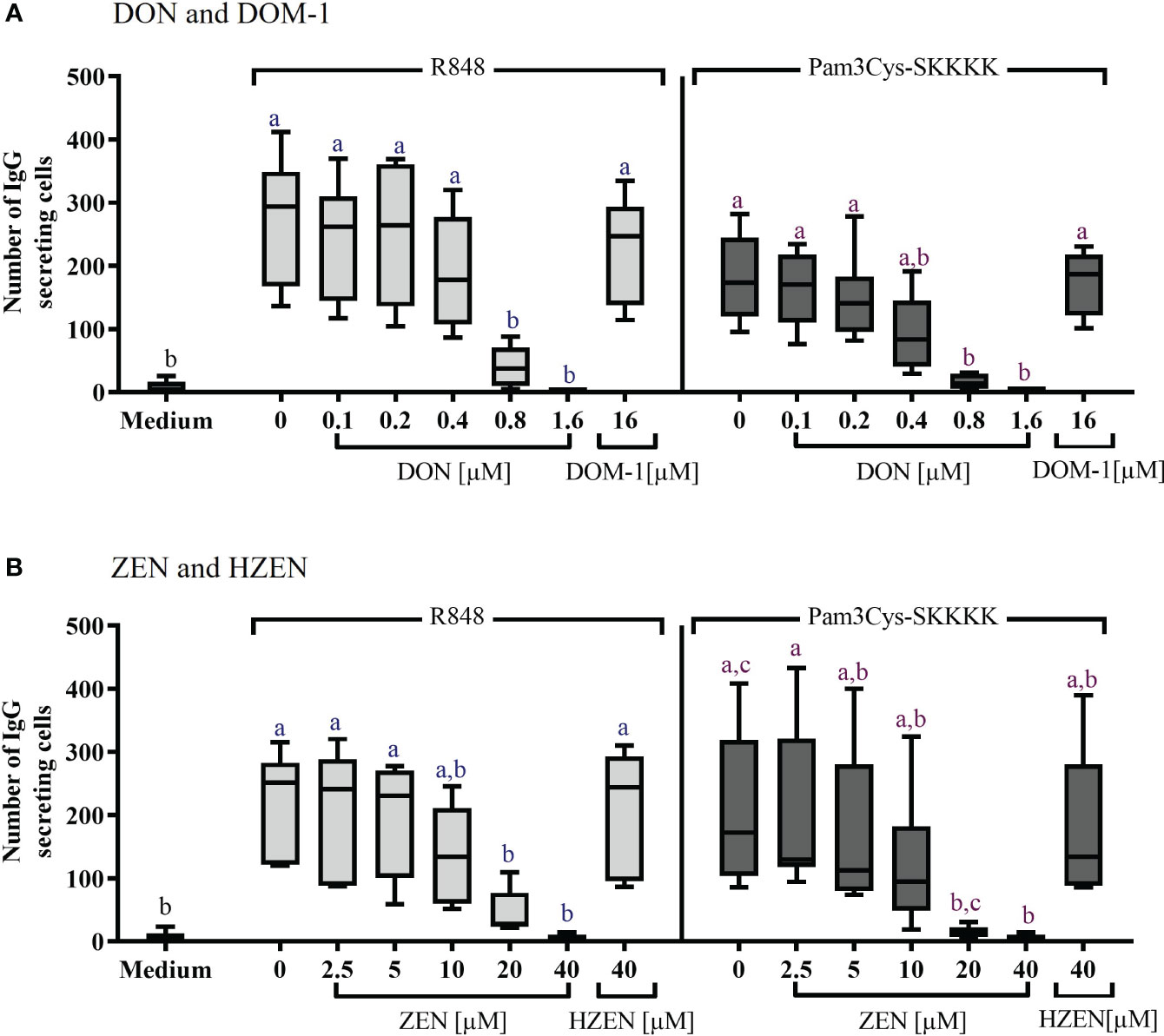
Figure 3 Number of IgG-secreting cells after stimulation with R848 or Pam3Cys-SKKKK and treatment with (A) DON [0.1-1.6 µM], DOM-1 [16 µM], (B) ZEN [2.5-40 µM], or HZEN [40 µM]. PBMCs were stimulated with R848 or Pam3Cys-SKKKK and cultivated in the absence or presence of DON, DOM-1, ZEN, or HZEN for three days. Thereafter, cells were transferred into ELISpot plates and IgG-secreting cells were quantified. Different letters indicate significant differences between the medium and treatments for each stimulation (One Way ANOVA, Bonferroni post-hoc test, p<0.05, n=6).
3.4 DON and ZEN reduce concentrations of IgM, IgG, and IgA in cell culture supernatants
The concentrations of IgM, IgG, and IgA in the supernatants of stimulated B cells, cultivated for FCM, were determined by ELISA. Significant increases of IgM, IgG, and IgA secretion were detected in supernatants of cells stimulated with either R848 or Pam3Cys-SKKKK, compared to cells exposed to cell culture medium alone (Figures 4A, B).
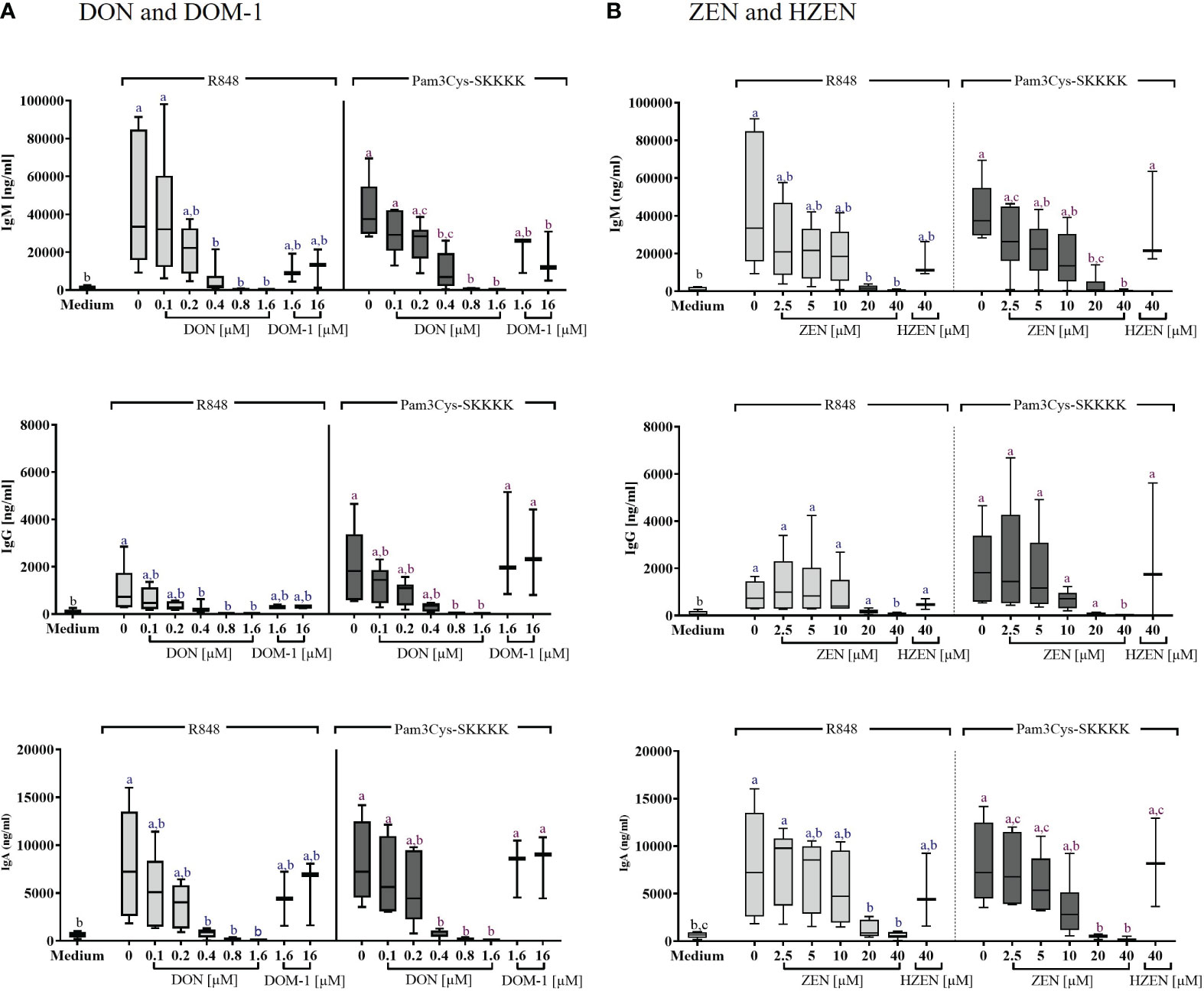
Figure 4 Concentrations of IgM, IgG, and IgA antibodies in the supernatant of R848 or Pam3CysSKKK stimulated PBMCs, following treatment with (A) DON [0.1-1.6 µM] or DOM-1 [1.6 and 16 µM] as well as (B) ZEN [2.5-40 µM] or HZEN [40 µM]. PBMCs were stimulated for 4 days with R848 or Pam3Cys-SKKKK in the presence or absence of the metabolites DON, DOM-1, ZEN, or HZEN. Antibody concentrations were determined in cell culture supernatants by ELISA. Different letters indicate significant differences between the medium control and treatments within each stimulation (One Way ANOVA, Bonferroni post-hoc test, p<0.05, n=6 for the mycotoxins and n=3 for the metabolites).
DON led to a significant reduction in IgM, IgG, and IgA levels of R848 stimulated B cells between 0.4 and 1.6 µM. In Pam3Cys-SKKKK stimulated B cells, IgM and IgA levels were reduced significantly between 0.4 and 1.6 µM DON, while IgG levels of Pam3Cys-SKKKK stimulated B cells were reduced significantly between 0.8 and 1.6 µM DON. DOM-1 [1.6 and 16 µM] did not induce significant changes of antibody concentrations in any of the applied treatments (Figure 4A).
ZEN significantly reduced IgM levels in supernatants of cells stimulated with R848 at a concentration of 20 µM (p=0.0027) and 40 µM (p=0.0018). Under Pam3Cys-SKKKK stimulation, ZEN induced a significant IgM reduction at 20 µM and 40 µM (both p<0.0001). Furthermore, IgG levels were decreased significantly by 40 µM ZEN (R848: p=0.0184; Pam3Cys-SKKKK: p=0.0019), regardless of the stimulus applied. Finally, ZEN significantly reduced IgA levels at both 20 µM (R848: p=0.0371; Pam3Cys-SKKKK: p=0.0009) and 40 µM (R848: p=0.0147; Pam3Cys-SKKKK: 0.0005), regardless of the stimulus used. Treatment with HZEN [40 µM] did not induce significant changes of antibody concentrations in any of the applied treatments (Figure 4B).
Effects of DON, DOM-1, ZEN and HZEN on the levels of IgM, IgG, and IgA in the supernatants of non-stimulated cells Supplementary Figure 8) showed similar results as in R848- and Pam3CysSKKK stimulated cells, however at a lower level, with significant reductions only seen for some conditions with high DON concentrations.
4 Discussion
The potential detrimental effects of the Fusarium-derived mycotoxins DON and ZEN have been extensively explored and documented, from both in vitro and in vivo perspectives (6, 12, 44, 50). Due to the consumption of cereal-rich feed, pigs – in particular – are heavily affected by both of these mycotoxins (12, 51). While acute DON consumption can cause diarrhea, emesis, leucocytosis, hemorrhage, endotoxemia and, ultimately, shock-like death, chronic low-dose exposure results in growth retardation and immunological impairments (6, 51). ZEN on the other hand, is predominantly known for inducing hyperestrogenism, causing diverse clinical symptoms including, among others, swelling and reddening of the vulva, metaplasia of uterus, ovarian atrophy, enlargement of the mammae, and reduced fertility (2, 11, 12).
The concentrations of DON and ZEN used throughout this study closely reflect the physiologically relevant concentrations in vivo. DON is absorbed in the upper gastrointestinal tract up to 100% suggesting a nearly complete systemic absorption and can be identified in the serum, exposing immune cells (52). Furthermore, Döll et al, 2003 (53) and Dänicke et al., 2004 (54) reported a comparable and linear relationship, with concentrations of up to 100 ng/mL DON (~ 0.3 µM) and 325 ng/mL DON (~1 µM) detected in serum. The systemic bioavailability of ZEN was reviewed to vary between 78 and 87% suggesting that just 22 to 13% of an oral bolus are excreted via feces and the rest gets in contact with immune cells (55). Studies have reported maximal plasma concentrations up to 15 µg/mL (47 µM) ZEN after oral ingestion of ZEN via feed (56). The metabolites DOM-1 and HZEN were tested in equivalent, but physiological not relevant concentrations, to example the non-toxic effects thereof. Overall, several studies have shown that especially DON and ZEN contamination in animal feed, even though regulated, are very common. In 74,821 samples of feed and feed raw materials (e.g., maize, wheat, soybean) collected from 100 countries, 88% of the samples were contaminated with at least one mycotoxin. In feed, maximal concentrations of DON was 84,860 µg/kg and of ZEN 105,000 µg/kg - making them both the most relevant and ubiquitously found mycotoxins (3).
Despite the substantial amount of information which has been generated for DON and ZEN-related health risks, detailed knowledge regarding impacts on immune parameters, particularly in the context of vaccine-related immune responses, is still lacking. We therefore investigated the influence of DON and ZEN on the proliferation and antibody production of porcine B cells, with antibody production ultimately resulting in humoral immunity. Our investigations uncovered detrimental effects of DON and ZEN on the survival rate of stimulated PBMCs as well as proliferation of total B cells and selected B-cell subsets (CD21+, IgM+, IgG+). In line with these findings, our results show that both DON and ZEN significantly decrease the number of IgG-secreting B cells as well as the secretion of IgM, IgG and IgA when measured in the supernatants of stimulated B cells. Finally, all analyses in our study include a direct comparison between the effects of DON and its metabolite DOM-1 as well as the impacts of ZEN and its detoxification product HZEN. For all tested parameters we report the absence of impairing effects of both metabolites, DOM-1 and HEZN on diverse parameters of B-cell functionality.
In order to analyze the effects of DON and ZEN, as well as their metabolites, PBMCs were stimulated using two commercially available Toll-like receptor (TLR) agonists, R848 (Resiquimod) and Pam3CysSKKKK. While R848 is a low molecular weight synthetic molecule which activates immune cells via the TLR7/TLR8 MyD88-dependent signaling pathway (57, 58), Pam3Cys-SKKKK is a synthetic analogue of naturally occurring lipoproteins, known for its activation of TLR 1/2. For both molecules it has been shown that they are powerful stimulators of porcine B cells, driving activation (CD25 up-regulation), proliferation and Ig-production (59). Effects of DON, DOM-1, ZEN, and HZEN on cell survival, proliferation of total B cells and B cell subsets, as well as production of Ig classes were also investigated in unstimulated cells (i.e. without TLR-agonist) (Supplementary Figures 3, 6, 8). It was clearly shown that the overall results obtained from such cultures were the same as in stimulated cells, but - unsurprisingly - at far lower levels. The latter is to be expected, since B cell proliferation and Ig production in vitro, in the absence of stimulation, is low. Due to this, for proliferation and Ig production significant reductions for the highest DON and ZEN concentration were not reached for several read-outs and conditions, but the data show exactly the same trend.
Irrespective of the TLR-agonist used, we demonstrated that DON [0.8-1.6 µM] leads to significant decreases of the proliferation of total B cell as well as specific B cell subsets, while DOM-1 left all these parameters unaffected. Similarly, several reports demonstrated a reduction in proliferation of whole PBMC cultures following ConA stimulation, which drives primarily T-cell proliferation (19, 20, 60, 61). In those studies, DON concentrations ranging from 0.33 µM to 0.94 µM started to impair cell proliferation, indicating some variability, probably depending on time of in vitro cultivation and methodology used for the analysis of proliferation.
With respect to ZEN, we observed that 40 µM of the mycotoxin leads to significant reductions of PBMC survival, at least when Pam3CysSKKK was used for stimulation. Furthermore, ZEN [20-40 µM] significantly reduced proliferation of total B cells as well as B-cell subsets. None of the ZEN-induced detrimental effects were observed after treatment with its metabolite HZEN. Studies have suggested that the damaging effects of ZEN on immune parameters such as cell viability and proliferation could be due to the fact that many cell types involved in the immune response have estrogenic surface receptors (62). Investigations have not only confirmed that ZEN induces apoptosis and necrosis in different immune cells, particularly in B and T cells, it has also been suggested that ZEN-induced immunosuppression is likely to be a direct result of the detrimental effects of the mycotoxin on B- and T-cell proliferation (30, 63). According to Forsell et al. (64), a study cited in the EFSA Scientific Opinion (2011) (65), proliferation of human lymphocytes stimulated with different mitogens was reduced by 50% in the presence of 3.5 µg/mL (=10.9 µM) ZEN. Furthermore, Berek et al. (63), compared the effects of ZEN and its derivatives alpha-zearalenol and ß-zeralenol with that of trichothecences on the proliferation of PBMCs, thereby showing – in accordance with our findings – that only high concentrations (>15 µM) of these toxins reduced PBMC proliferation. This was also confirmed by another investigation (66) which treated freshly isolated human PBMCs with ZEN [0.1-30 µg/mL (94 µM)] and showed proliferation inhibition and necrosis induction of B and T lymphocytes only that the highest concentration of 30 µg/mL. In comparison to ZEN, HZEN did not have any effect on the proliferation of human immune cells.
While the impacts of DON and ZEN on immune cell proliferation have been shown in our study, but also other published work, we provide new information with regard to their effects on specific B-cell subsets (CD21+, IgM+, IgG+). IgM is expressed as a transmembrane receptor in B1 cells and naïve B2 cells. Following recognition of cognate antigen some naïve B2 cells do not undergo class switch and can differentiate into memory B cells which still express IgM as their B-cell receptor (BCR) (67). For porcine B cells, it has been suggested that naïve B2 cells express CD21 and IgM, whereas B1 cells have an IgM+CD21- phenotype (59). We observed that proliferation of CD21+ B cells was already impaired at lower concentrations of both DON [0.4 μM] and ZEN [10 μM, but only for R848 stimulation] than IgM+ and IgG+ subsets. This may indicate that CD21+ naïve B2 cells are more susceptible to the immunosuppressive effects of DON and ZEN than B1 cells and class-switched IgG+ B cells, although the underlying molecular mechanisms await elucidation. For DON, this might be due to differences in the capacity of different B-cell subsets to enter a ‘ribotoxic stress mode’ (see introduction), whereas for ZEN, differences in estrogen-receptor expression might be relevant. Nevertheless, from 0.4 μM DON and 20 μM ZEN onwards, IgM+ B cells also showed reduced proliferation rates and IgG+ B cells were affected at the highest DON and ZEN concentrations tested.
While this suggests that IgG+ B cells are less susceptible to DON and ZEN, their capacity for IgG secretion tested by ELISpot assays was already reduced at 20 μM of ZEN (Figure 3B), indicating that capacity for proliferation and antibody secretion may not respond in the same way to the actions of ZEN. Still, available ELISA tests allowed us to investigate IgM, IgA, and IgG in supernatants from the same samples (Figure 4) and also here, IgM and IgA production was affected at lower concentrations for DON and ZEN than IgG production.
Taken together, these findings may indicate that in particular naïve B2 cells are affected by the mycotoxins under investigation in our study. In the context of vaccination, this could imply that the induction of a primary immune response is more affected. This may result in an impaired formation of immunological memory, which in turn could lead to reduced vaccine efficacy.
In addition to providing significant information regarding the effects of DON and ZEN on cellular and humoral immune parameters, we demonstrated the DON metabolite DOM-1 and ZEN metabolite H-ZEN did not affect the above-mentioned parameters, thereby confirming the detoxifying nature of the underlying biotransformation processes. This is particularly important because even though common pre- and post-harvest mitigation strategies (68–74) as well as other technological approaches (14, 75) successfully reduce contamination levels, they fail to sufficiently clear agricultural products of mycotoxins. The use of feed additives, which enable biodegradation (38, 76, 77) or absorption (78, 79) of residual mycotoxins in the gastrointestinal tract, thereby reducing bioavailability and/or toxicity, is essential. We therefore provide valuable information, confirming the lack of negative effects of both DOM-1 and HZEN on B-cell functionality. It should be mentioned that with respect to HZEN, only a single investigation has been published, reporting a significantly reduced estrogenic effect of HZEN, compared to ZEN, in female pigs [43]. Our study is thus the first to provide information regarding the effects of HZEN on the proliferation and antibody production of immune cells.
Thus, the current study confirms that DON and ZEN, however not their derivatives DOM-1 and HZEN, compromise PBMC survival, proliferation of total B cells, as well as B cell subsets, decrease the number of IgG secreting cells and impair secretion of IgM, IgG, and IgA. We thereby not only provide additional insights regarding the negative effects of DON and especially ZEN on porcine B-cell function, we also confirm the efficacy of the detoxification process of DON to DOM-1 and ZEN to HZEN. Considering the pivotal function of B cells in the formation of protective humoral immunity, our findings emphasize the relevance of strict mycotoxin monitoring programs and the benefit of deactivators, which should contribute to improved animal health and welfare.
Data availability statement
The raw data supporting the conclusions of this article will be made available by the authors, without undue reservation.
Ethics statement
Ethical approval was not required for the study involving animals in accordance with the local legislation and institutional requirements because PBMCs were isolated from the blood of six 6-months-old pigs from an abattoir, which slaughters animals from different conventional finishing farms. Animals were subjected to electric high voltage anesthesia followed by exsanguination. This procedure is in accordance with the Austrian Animal Welfare Slaughter Regulation.
Author contributions
AP: Conceptualization, Data curation, Formal analysis, Investigation, Methodology, Visualization, Writing – original draft, Writing – review & editing. AK: Data curation, Visualization, Writing – original draft, Writing – review & editing. EM: Conceptualization, Funding acquisition, Investigation, Methodology, Project administration, Resources, Supervision, Validation, Writing – review & editing. WG: Conceptualization, Funding acquisition, Investigation, Methodology, Project administration, Resources, Supervision, Validation, Writing – review & editing.
Funding
The author(s) declare financial support was received for the research, authorship, and/or publication of this article. This research and the position of AP were funded by the Austrian Research Promotion Agency (FFG), grant number 855707.
Acknowledgments
The authors thank Maria Stadler and Martina Patzl for their help in the establishment of IgG ELISpot assays and IgM/IgG/IgA ELISAs.
Conflict of interest
EM and AK are employed by dsm-firmenich, which produces animal feed additives. This, however, did not influence the design of the experimental studies or bias the presentation and interpretation of results.
The remaining authors declare that the research was conducted in the absence of any commercial or financial relationships that could be construed as a potential conflict of interest.
Publisher’s note
All claims expressed in this article are solely those of the authors and do not necessarily represent those of their affiliated organizations, or those of the publisher, the editors and the reviewers. Any product that may be evaluated in this article, or claim that may be made by its manufacturer, is not guaranteed or endorsed by the publisher.
Supplementary material
The Supplementary Material for this article can be found online at: https://www.frontiersin.org/articles/10.3389/fimmu.2024.1338937/full#supplementary-material
References
1. Streit E, Schatzmayr G, Tassis P, Tzika E, Marin D, Taranu I, et al. Current situation of mycotoxin contamination and co-occurrence in animal feed focus on Europe. Toxins (Basel) (2012) 4(10):788–809. doi: 10.3390/toxins4100788
2. Zinedine A, Soriano JM, Moltó JC, Mañes J. Review on the toxicity, occurrence, metabolism, detoxification, regulations and intake of zearalenone: an oestrogenic mycotoxin. Food Chem Toxicol an Int J Publ Br Ind Biol Res Assoc (2007) 45(1):1–18. doi: 10.1016/j.fct.2006.07.030
3. Gruber-Dorninger C, Jenkins T, Schatzmayr G. Global mycotoxin occurrence in feed: A ten-year survey. Toxins (Basel) (2019) 11(7):375. doi: 10.3390/toxins11070375
4. Schatzmayr G, Streit E. Global occurrence of mycotoxins in the food and feed chain: Facts and figures. World Mycotoxin J (2013) 6(3):213–22. doi: 10.3920/WMJ2013.1572
5. Tolosa J, Rodríguez-Carrasco Y, Ruiz MJ, Vila-Donat P. Multi-mycotoxin occurrence in feed, metabolism and carry-over to animal-derived food products: A review. Food Chem Toxicol (2021) 158:112661. doi: 10.1016/j.fct.2021.112661
6. Pestka JJ. Deoxynivalenol: mechanisms of action, human exposure, and toxicological relevance. Arch Toxicol (2010) 84(9):663–79. doi: 10.1007/s00204-010-0579-8
7. Garreau de Loubresse N, Prokhorova I, Holtkamp W, Rodnina MV, Yusupova G, Yusupov M. Structural basis for the inhibition of the eukaryotic ribosome. Nature (2014) 513(7519):517–22. doi: 10.1038/nature13737
8. Pestka JJ. Deoxynivalenol-induced proinflammatory gene expression: mechanisms and pathological sequelae. Toxins (Basel) (2010) 2(6):1300–17. doi: 10.3390/toxins2061300
9. Vrtačnik P, Ostanek B, Mencej-Bedrač S, Marc J. The many faces of estrogen signaling. Biochem medica (2014) 24(3):329–42. doi: 10.11613/BM.2014.035
10. Kowalska K, Ewa H-G, Piastowska-Ciesielska A. Zearalenone as an endocrine disruptor in humans. Environ Toxicol Pharmacol (2016) 48:141–9. doi: 10.1016/j.etap.2016.10.015
11. Fink-Gremmels J, Malekinejad H. Clinical effects and biochemical mechanisms associated with exposure to the mycoestrogen zearalenone. Anim Feed Sci Technol (2007) 137(3):326–41. doi: 10.1016/j.anifeedsci.2007.06.008
12. Ropejko K, Twarużek M. Zearalenone and its metabolites-general overview, occurrence, and toxicity. Toxins (Basel) (2021) 13(1):35. doi: 10.3390/toxins13010035
13. Zhang GL, Feng YL, Song JL, Zhou XS. Zearalenone: A mycotoxin with different toxic effect in domestic and laboratory animals’ Granulosa cells. Front Genet (2018) 9(December):1–8. doi: 10.3389/fgene.2018.00667
14. Rogowska A, Pomastowski P, Sagandykova G, Buszewski B. Zearalenone and its metabolites: Effect on human health, metabolism and neutralisation methods. Toxicon (2019) 162:46–56. doi: 10.1016/j.toxicon.2019.03.004
15. Rai A, Das M, Tripathi A. Occurrence and toxicity of a fusarium mycotoxin, zearalenone. Crit Rev Food Sci Nutr (2020) 60(16):2710–29. doi: 10.1080/10408398.2019.1655388
16. Marin DE, Pistol GC, Bulgaru CV, Taranu. Cytotoxic and inflammatory effects of individual and combined exposure of HepG2 cells to zearalenone and its metabolites. Naunyn Schmiedebergs Arch Pharmacol (2019) 392(8):937–47. doi: 10.1007/s00210-019-01644-z
17. Zinedine A, Ruiz M-J. Zearalenone. In: Mycotoxins and their Implications in Food Safety. Future Science Ltd (2014). p. 52–66. doi: 10.4155/ebo.13.660
18. Gil-Serna J, Vázquez C, Patino B. Mycotoxins | Toxicology. Ref Mod Food Sci (2019). doi: 10.1016/B978-0-08-100596-5.22630-9
19. Novak B, Vatzia E, Springler A, Pierron A, Gerner W, Reisinger N, et al. Bovine peripheral blood mononuclear cells are more sensitive to deoxynivalenol than those derived from poultry and swine. Toxins (Basel) (2018) 10(4):152. doi: 10.3390/toxins10040152
20. Taranu I, Marin DE, Burlacu R, Pinton P, Damian V, Oswald IP. Comparative aspects of in vitro proliferation of human and porcine lymphocytes exposed to mycotoxins. Arch Anim Nutr (2010) 64(5):383–93. doi: 10.1080/1745039X.2010.492140
21. Vatzia E, Pierron A, Saalmüller A, Mayer E, Gerner W. Deoxynivalenol affects proliferation and expression of activation-related molecules in major porcine T-cell subsets. Toxins (Basel) (2019) 11(11):644. doi: 10.3390/toxins11110644
22. Bimczok D, Döll S, Rau H, Goyarts T, Wundrack N, Naumann M, et al. The Fusarium toxin deoxynivalenol disrupts phenotype and function of monocyte-derived dendritic cells in vivo and in vitro. Immunobiology (2007) 212(8):655–66. doi: 10.1016/j.imbio.2007.05.002
23. Hendriks J, Xiao Y, Borst J. CD27 promotes survival of activated T cells and complements CD28 in generation and establishment of the effector T cell pool. J Exp Med (2003) 198(9):1369–80. doi: 10.1084/jem.20030916
24. Burr JS, Savage NDL, Messah GE, Kimzey SL, Shaw AS, Arch RH, et al. Cutting edge: distinct motifs within CD28 regulate T cell proliferation and induction of bcl-XL1. J Immunol (2001) 166(9):5331–5. doi: 10.4049/jimmunol.166.9.5331
25. Vatzia E, Pierron A, Hoog AM, Saalmüller A, Mayer E, Gerner W. Deoxynivalenol has the capacity to increase transcription factor expression and cytokine production in porcine T cells. Front Immunol (2020) 11(August):1–17. doi: 10.3389/fimmu.2020.02009
26. Seyed Toutounchi N, Braber S, van’t Land B, Thijssen S, Garssen J, Kraneveld AD, et al. Exposure to deoxynivalenol during pregnancy and lactation enhances food allergy and reduces vaccine responsiveness in the offspring in a mouse model. Front Immunol (2021) 12(December):1–15. doi: 10.3389/fimmu.2021.797152
27. Pierron A, Alassane-Kpembi I, Oswald IP. Impact of mycotoxin on immune response and consequences for pig health. Anim Nutr (2016) 2(2):63–8. doi: 10.1016/j.aninu.2016.03.001
28. Islam MR, Kim JW, Roh Y-S, Kim J-H, Han KM, Kwon H-J, et al. Evaluation of immunomodulatory effects of zearalenone in mice. J Immunotoxicol (2017) 14(1):125–36. doi: 10.1080/1547691X.2017.1340371
29. Salah-Abbès JB, Abbès S, Houas Z, Abdel-Wahhab MA, Oueslati R. Zearalenone induces immunotoxicity in mice: possible protective effects of radish extract (Raphanus sativus). J Pharm Pharmacol (2008) 60(6):761–70. doi: 10.1211/jpp.60.6.0012
30. Abbès S, Salah-Abbès JB, Ouanes Z, Houas Z, Othman O, Bacha H, et al. Preventive role of phyllosilicate clay on the Immunological and Biochemical toxicity of zearalenone in Balb/c mice. Int Immunopharmacol (2006) 6(8):1251–8. doi: 10.1016/j.intimp.2006.03.012
31. Choi B-K, Cho J-H, Jeong S-H, Shin H-S, Son S-W, Yeon Y-K, et al. Zearalenone affects immune-related parameters in lymphoid organs and serum of rats vaccinated with porcine parvovirus vaccine. Toxicol Res (2012) 28(4):279–86. doi: 10.5487/TR.2012.28.4.279
32. Wu F, Cui J, Yang X, Liu S, Han S, Chen B. Effects of zearalenone on genital organ development, serum immunoglobulin, antioxidant capacity, sex hormones and liver function of prepubertal gilts. Toxicon (2021) 189:39–44. doi: 10.1016/j.toxicon.2020.11.005
33. Reddy KE, Lee W, Lee SD, Jeong JY, Kim DW, Kim M, et al. 411 Effects of dietary deoxynivalenol and zearalenone on the organ pro-inflammatory gene expressions and serum immunoglobulins of pigs. J Anim Sci (2017) 95(suppl_4):203. doi: 10.2527/asasann.2017.411
34. Ren ZH, Zhou R, Deng JL, Zuo ZC, Peng X, Wang YC, et al. Effects of the Fusarium toxin zearalenone (ZEA) and/or deoxynivalenol (DON) on the serum IgA, IgG and IgM levels in mice. Food Agric Immunol (2014) 25(4):600–6. doi: 10.1080/09540105.2013.867928
35. Yang L, Yang W, Feng Q, Huang L, Zhang G, Liu F, et al. Effects of purified zearalenone on selected immunological measurements of blood in post-weaning gilts. Anim Nutr (2016) 2(3):142–8. doi: 10.1016/j.aninu.2016.04.008
36. Marin DE, Taranu I, Burlacu R, Manda G, Motiu M, Neagoe I, et al. Effects of zearalenone and its derivatives on porcine immune response. Toxicol Vitr (2011) 25(8):1981–8. doi: 10.1016/j.tiv.2011.06.022
37. Fuchs E, Binder EM, Heidler D, Krska R. Structural characterization of metabolites after the microbial degradation of type A trichothecenes by the bacterial strain BBSH 797. Food Addit Contam (2002) 19(4):379–86. doi: 10.1080/02652030110091154
38. Gruber-Dorninger C, Faas J, Doupovec B, Aleschko M, Stoiber C, Höbartner-Gußl A, et al. Metabolism of zearalenone in the rumen of dairy cows with and without application of a zearalenone-degrading enzyme. Toxins (2021) 13:84. doi: 10.3390/toxins13020084
39. Gruber-Dorninger C, Killinger M, Höbartner-Gußl A, Rosen R, Doupovec B, Aleschko M, et al. Enzymatic degradation of zearalenone in the gastrointestinal tract of pigs, chickens, and rainbow trout. Toxins (Basel) (2023) 15(1):1–19. doi: 10.3390/toxins15010048
40. Ruhnau D, Hess C, Doupovec B, Grenier B, Schatzmayr D, Hess M, et al. Deepoxy-deoxynivalenol (DOM-1), a derivate of deoxynivalenol (DON), exhibits less toxicity on intestinal barrier function, Campylobacter jejuni colonization and translocation in broiler chickens. Gut Pathog (2021) 13(1):44. doi: 10.1186/s13099-021-00440-6
41. Mayer E, Novak B, Springler A, Schwartz-Zimmermann HE, Nagl V, Reisinger N, et al. Effects of deoxynivalenol (DON) and its microbial biotransformation product deepoxy-deoxynivalenol (DOM-1) on a trout, pig, mouse, and human cell line. Mycotoxin Res (2017) 33(4):297–308. doi: 10.1007/s12550-017-0289-7
42. Springler A, Hessenberger S, Schatzmayr G, Mayer E. Early activation of MAPK p44/42 is partially involved in DON-induced disruption of the intestinal barrier function and tight junction network. Toxins (Basel) (2016) 8(9):264. doi: 10.3390/toxins8090264
43. Springler A, Hessenberger S, Reisinger N, Kern C, Nagl V, Schatzmayr G, et al. Deoxynivalenol and its metabolite deepoxy-deoxynivalenol: multi-parameter analysis for the evaluation of cytotoxicity and cellular effects. Mycotoxin Res (2017) 33(1):25–37. doi: 10.1007/s12550-016-0260-z
44. Fruhauf S, Novak B, Nagl V, Hackl M, Hartinger D, Rainer V, et al. Biotransformation of the Mycotoxin Zearalenone to its Metabolites Hydrolyzed Zearalenone (HZEN) and Decarboxylated Hydrolyzed Zearalenone (DHZEN) Diminishes its Estrogenicity In Vitro and In Vivo. Toxins (Basel) (2019) 11(8):481. doi: 10.3390/toxins11080481
45. Pollard AJ, Bijker EM. A guide to vaccinology: from basic principles to new developments. Nat Rev Immunol (2021) 21(2):83–100. doi: 10.1038/s41577-020-00479-7
46. Fruhauf S, Pühringer D, Thamhesl M, Fajtl P, Kunz-Vekiru E, Höbartner-Gußl A, et al. Bacterial lactonases ZenA with Noncanonical Structural Features Hydrolyze the Mycotoxin Zearalenone. United States: ACS Catal.
47. Reutner K, Leitner J, Essler SE, Witter K, Patzl M, Steinberger P, et al. Porcine CD27: Identification, expression and functional aspects in lymphocyte subsets in swine. Dev Comp Immunol (2012) 38(2):321–31. doi: 10.1016/j.dci.2012.06.011
48. Bracarense APFL, Pierron A, Pinton P, Gerez JR, Schatzmayr G, Moll WD, et al. Reduced toxicity of 3-epi-deoxynivalenol and de-epoxy-deoxynivalenol through deoxynivalenol bacterial biotransformation: In vivo analysis in piglets. Food Chem Toxicol (2020) 140(February):111241. doi: 10.1016/j.fct.2020.111241
49. Villanueva-Hernández S, Adib Razavi M, van Dongen KA, Stadler M, de Luca K, Beyersdorf N, et al. Co-expression of the B-cell key transcription factors blimp-1 and IRF4 identifies plasma cells in the pig. Front Immunol (2022) 13(April):1–18. doi: 10.3389/fimmu.2022.854257
50. Pierron A, Mimoun S, Murate LS, Loiseau N, Lippi Y, Bracarense A-PFL, et al. Microbial biotransformation of DON: molecular basis for reduced toxicity. Sci Rep (2016) 6(1):29105. doi: 10.1038/srep29105
51. Rotter BA, Prelusky DB, Pestka JJ. Toxicology of deoxynivalenol (vomitoxin). J Toxicol Environ Health (1996) 48(1):1–34. doi: 10.1080/009841096161447
52. Dänicke S, Brezina U. Kinetics and metabolism of the Fusarium toxin deoxynivalenol in farm animals: Consequences for diagnosis of exposure and intoxication and carry over. Food Chem Toxicol (2013) 60:58–75. doi: 10.1016/j.fct.2013.07.017
53. Döll S, Dänicke S, Ueberschär K-H, Valenta H, Schnurrbusch U, Ganter M, et al. Effects of graded levels of Fusarium toxin contaminated maize in diets for female weaned piglets. Arch Anim Nutr (2003) 57(5):311–34. doi: 10.1080/00039420310001607680
54. Dänicke S, Goyarts T, Valenta H, Razzazi E, Böhm J. On the effects of deoxynivalenol (DON) in pig feed on growth performance, nutrients utilization and DON metabolism. J Anim Feed Sci (2004) 13(4):539–56. doi: 10.22358/jafs/67624/2004
55. Dänicke S, Winkler J. Invited review: Diagnosis of zearalenone (ZEN) exposure of farm animals and transfer of its residues into edible tissues (carry over). Food Chem Toxicol (2015) 84:225–49. doi: 10.1016/j.fct.2015.08.009
56. Kostro K, Gajecka M, Lisiecka U, Majer-Dziedzic B, Obremski K, Zielonka L, et al. Subpulation of lymphocytes CD4+and CD8+ in peropheral blood of sheep with zearalenone mycotoxicosis. Bull Vet Inst Puławy (2011) 55(2):41–246.
57. Hemmi H, Kaisho T, Takeuchi O, Sato S, Sanjo H, Hoshino K, et al. Small anti-viral compounds activate immune cells via the TLR7 MyD88-dependent signaling pathway. Nat Immunol (2002) 3(2):196–200. doi: 10.1038/ni758
58. Jurk M, Heil F, Vollmer J, Schetter C, Krieg AM, Wagner H, et al. Human TLR7 or TLR8 independently confer responsiveness to the antiviral compound R-848. Nat Immunol (2002) 3:499. doi: 10.1038/ni0602-499
59. Braun RO, Python S, Summerfield A. Porcine B cell subset responses to toll-like receptor ligands. Front Immunol (2017) 8(AUG). doi: 10.3389/fimmu.2017.01044
60. Goyarts T, Grove N, Dänicke S. Effects of the Fusarium toxin deoxynivalenol from naturally contaminated wheat given subchronically or as one single dose on the in vivo protein synthesis of peripheral blood lymphocytes and plasma proteins in the pig. Food Chem Toxicol an Int J Publ Br Ind Biol Res Assoc (2006) 44(12):1953–65. doi: 10.1016/j.fct.2006.06.017
61. Dänicke S, Hegewald A-K, Kahlert S, Kluess J, Rothkötter H-J, Breves G, et al. Studies on the toxicity of deoxynivalenol (DON), sodium metabisulfite, DON-sulfonate (DONS) and de-epoxy-DON for porcine peripheral blood mononuclear cells and the Intestinal Porcine Epithelial Cell lines IPEC-1 and IPEC-J2, and on effects of DON and DON. Food Chem Toxicol an Int J Publ Br Ind Biol Res Assoc (2010) 48(8–9):2154–62. doi: 10.1016/j.fct.2010.05.022
62. Wang YC, Deng JL, Xu SW, Peng X, Zuo ZC, Cui HM, et al. Effects of zearalenone on IL-2, IL-6, and IFN-γ mRNA levels in the splenic lymphocytes of chickens. Sci World J (2012) 2012. doi: 10.1128/aem.49.6.1523-1526.1985
63. Berek L, Petri IB, Mesterházy Á, Téren J, Molnár J. Effects of mycotoxins on human immune functions in vitro. Toxicol Vitr (2001) 15(1):25–30. doi: 10.1016/S0887-2333(00)00055-2
64. Forsell JH, Kateley JR, Yoshizawa T, Pestka JJ. Inhibition of mitogen-induced blastogenesis in human lymphocytes by T-2 toxin and its metabolites. Appl Environ Microbiol (1985) 49(6):1523–6. doi: 10.1128/aem.49.6.1523-1526.1985
65. Opinion S. Scientific opinion on genotoxicity testing strategies applicable to food and feed safety assessment. EFSA J (2011) 9(9). doi: 10.2903/j.efsa.2011.2379
66. Vlata Z, Porichis F, Tzanakakis G, Tsatsakis A, Krambovitis E. A study of zearalenone cytotoxicity on human peripheral blood mononuclear cells. Toxicol Lett (2006) 165(3):274–81. doi: 10.1016/j.toxlet.2006.05.001
67. Baumgarth N. The shaping of a B cell pool maximally responsive to infections. Annu Rev Immunol (2021) 39:103–29. doi: 10.1146/annurev-immunol-042718-041238
68. Cheli F, Pinotti L, Novacco M, Ottoboni M, Tretola M, Orto VD‘. Mycotoxins in Wheat and Mitigation Measures. In: Wheat Improvement, Management and Utilization (2017). doi: 10.5772/67240
69. Edwards SG. Influence of agricultural practices on fusarium infection of cereals and subsequent contamination of grain by trichothecene mycotoxins. Toxicol Lett (2004) 153(1):29–35. doi: 10.1016/j.toxlet.2004.04.022
70. Steiner B, Buerstmayr M, Michel S, Schweiger W, Lemmens M, Buerstmayr H. Breeding strategies and advances in line selection for Fusarium head blight resistance in wheat. Trop Plant Pathol (2017) 42(3):165–74. doi: 10.1007/s40858-017-0127-7
71. Mesterházy A. Types and components of resistance to Fusarium head blight of wheat. Plant Breed (1995) 114(5):377–86. doi: 10.1111/j.1439-0523.1995.tb00816.x
72. Leslie JF, Moretti A, Mesterházy Á, Ameye M, Audenaert K, Singh PK, et al. Key global actions for mycotoxin management in wheat and other small grains. Toxins (2021) 13(10):725. doi: 10.3390/toxins13100725
73. Steinkellner S, Langer I. Impact of tillage on the incidence ofFusarium spp. in soil. Plant Soil (2004) 267(1):13–22. doi: 10.1007/s11104-005-2574-z
74. Mutiga SK, Mushongi AA, Kangéthe EK. Enhancing food safety through adoption of long-term technical advisory, financial, and storage support services in maize growing areas of east Africa. Sustainability (2019) 11:2827. doi: 10.3390/su11102827
75. Ji C, Fan Y, Zhao L. Review on biological degradation of mycotoxins. Anim Nutr (Zhongguo xu mu shou yi xue hui) (2016) 2(3):127–33. doi: 10.1016/j.aninu.2016.07.003
76. Masching S, Naehrer K, Schwartz-Zimmermann H-E, Sărăndan M, Schaumberger S, Dohnal I, et al. Gastrointestinal degradation of fumonisin B1 by carboxylesterase fumD prevents fumonisin induced alteration of sphingolipid metabolism in Turkey and swine. Toxins (2016) 8:84. doi: 10.3390/toxins8030084
77. Grenier B, Bracarense A-PFL, Schwartz HE, Lucioli J, Cossalter A-M, Moll W-D, et al. Biotransformation approaches to alleviate the effects induced by fusarium mycotoxins in swine. J Agric Food Chem (2013) 61(27):6711–9. doi: 10.1021/jf400213q
78. Phillips TD, Wang M, Elmore SE, Hearon SE, Wang J-S. NovaSil clay for the protection of humans and animals from aflatoxins and other contaminants. Clays Clay Miner (2019) 67:99–110. doi: 10.1007/s42860-019-0008-x
Keywords: deoxynivalenol, de-epoxy-deoxynivalenol, zearalenone, hydrolyzed zearalenone, B cells, immune system
Citation: Pierron A, Kleber A, Mayer E and Gerner W (2024) Effect of DON and ZEN and their metabolites DOM-1 and HZEN on B cell proliferation and antibody production. Front. Immunol. 15:1338937. doi: 10.3389/fimmu.2024.1338937
Received: 15 November 2023; Accepted: 24 January 2024;
Published: 21 February 2024.
Edited by:
Reiko Shinkura, The University of Tokyo, JapanReviewed by:
Marit Zuurveld, Utrecht University, NetherlandsKay L. Medina, Mayo Clinic, United States
Copyright © 2024 Pierron, Kleber, Mayer and Gerner. This is an open-access article distributed under the terms of the Creative Commons Attribution License (CC BY). The use, distribution or reproduction in other forums is permitted, provided the original author(s) and the copyright owner(s) are credited and that the original publication in this journal is cited, in accordance with accepted academic practice. No use, distribution or reproduction is permitted which does not comply with these terms.
*Correspondence: Alexandra Kleber, Alexandra.Kleber@dsm.com
†Present addresses: Alix Pierron, ENVT (National Veterinary School of Toulouse), IHAP, Toulouse, France
Wilhelm Gerner, The Pirbright Institute, Woking, United Kingdom
‡These authors share first authorship