- 1Department of Fundamental and Applied Neurobiology, V. P. Serbsky National Medical Research Center of Psychiatry and Narcology, The Ministry of Health of the Russian Federation, Moscow, Russia
- 2Department of Medical Nanobiotechnology, Institute of Translational Medicine, N.I. Pirogov Russian National Research Medical University, The Ministry of Health of the Russian Federation, Moscow, Russia
- 3Center for Precision Genome Editing and Genetic Technologies for Biomedicine, Engelhardt Institute of Molecular Biology, Russian Academy of Sciences, Moscow, Russia
Despite significant advances in our knowledge regarding the genetics and molecular biology of gliomas over the past two decades and hundreds of clinical trials, no effective therapeutic approach has been identified for adult patients with newly diagnosed glioblastoma, and overall survival remains dismal. Great hopes are now placed on combination immunotherapy. In clinical trials, immunotherapeutics are generally tested after standard therapy (radiation, temozolomide, and steroid dexamethasone) or concurrently with temozolomide and/or steroids. Only a minor subset of patients with progressive/recurrent glioblastoma have benefited from immunotherapies. In this review, we comprehensively discuss standard therapy-related systemic immunosuppression and lymphopenia, their prognostic significance, and the implications for immunotherapy/oncolytic virotherapy. The effectiveness of immunotherapy and oncolytic virotherapy (viro-immunotherapy) critically depends on the activity of the host immune cells. The absolute counts, ratios, and functional states of different circulating and tumor-infiltrating immune cell subsets determine the net immune fitness of patients with cancer and may have various effects on tumor progression, therapeutic response, and survival outcomes. Although different immunosuppressive mechanisms operate in patients with glioblastoma/gliomas at presentation, the immunological competence of patients may be significantly compromised by standard therapy, exacerbating tumor-related systemic immunosuppression. Standard therapy affects diverse immune cell subsets, including dendritic, CD4+, CD8+, natural killer (NK), NKT, macrophage, neutrophil, and myeloid-derived suppressor cell (MDSC). Systemic immunosuppression and lymphopenia limit the immune system’s ability to target glioblastoma. Changes in the standard therapy are required to increase the success of immunotherapies. Steroid use, high neutrophil-to-lymphocyte ratio (NLR), and low post-treatment total lymphocyte count (TLC) are significant prognostic factors for shorter survival in patients with glioblastoma in retrospective studies; however, these clinically relevant variables are rarely reported and correlated with response and survival in immunotherapy studies (e.g., immune checkpoint inhibitors, vaccines, and oncolytic viruses). Our analysis should help in the development of a more rational clinical trial design and decision-making regarding the treatment to potentially improve the efficacy of immunotherapy or oncolytic virotherapy.
1 Introduction
The principal therapeutic armamentarium for patients with newly diagnosed glioblastoma (grade IV glioma) includes maximal safe resection, conventionally fractionated radiotherapy with the concurrent and adjuvant DNA-alkylating drug temozolomide, and the corticosteroid dexamethasone to treat vasogenic cerebral edema. Despite multifaceted standard therapeutic interventions, five-year overall survival remains dismal (≤7% overall by age) (1, 2).
Clinical trials of immunotherapies, including vaccines and oncolytic viruses, have demonstrated encouraging efficacy in minor subsets of patients with progressive/recurrent glioblastoma (3–8). Different patient selection criteria, recombinant viruses, protocols for vaccine preparation, and schedules and routes of vaccine or virus administration have been applied in trials. However, within individual studies, some patients exhibited significant, moderate, or no therapeutic response and infiltration of lymphocytes to the tumor site following treatment, indicating that some patients’ intrinsic factors may determine a response to therapy. Several pretreatment variables, such as age, performance status, extent of tumor resection, O-6-methylguanine-DNA methyltransferase (MGMT) promoter methylation status, and isocitrate dehydrogenase (IDH) mutation status, are known independent prognostic and/or stratification factors in patients with glioblastoma/gliomas (9–11). However, increasing clinical evidence suggests that additional intrinsic factors in patients, which may determine the efficacy of immunotherapeutics and correlate with overall survival, are immune-related.
The largely negative results from phase I/II, II and III clinical trials of oncolytic viruses, vaccines, and immune checkpoint inhibitors in glioblastoma are supposed to be due to a number of potential barriers to the efficacy of immunotherapeutics, including inter- and intratumoral spatial and temporal cellular genetic and phenotypic heterogeneity and plasticity, insufficient immunogenicity, neoantigenic loss under therapy pressure (e.g., epidermal growth factor receptor variant III, EGFRvIII), the low expression of major histocompatibility complex, upregulation of diverse immune checkpoint inhibitors on infiltrating T lymphocytes, and increased levels/ratios of tumor-infiltrating immunosuppressive immune subsets [e.g., macrophages, neutrophils, myeloid-derived suppressor cells (MDSCs), and regulatory T cells (Tregs)] (12–20). Although the blood-brain barrier in glioblastoma is partially disrupted (21), leading to the infiltration of innate and adaptive immune cells (20), glioblastoma is nonetheless largely characterized by the absence or exclusion of T cells in the tumor microenvironment (“cold”, “immune-desert”/”immune-excluded” phenotype) (22, 23) and T cell dysfunction, including tolerance and exhaustion (23, 24). In addition to hypoxia, the glioblastoma cell metabolism contributes to immunotherapy resistance (25). In the tumor microenvironment, anti-tumor immune cells compete with tumor cells for various nutrients (e.g., glucose, glutamine, arginine, and lipids). Increased tumor glycolysis and glutaminolysis, altered tryptophan (via the kynurenine pathway), arginine, and lipid metabolism are associated not only with limited availability of critical nutrients for immune cell functions in the tumor microenvironment but also lead to acidosis, increased accumulation of lactic acid, α-ketoglutarate, and kynurenine metabolites, negatively affecting metabolism and effector function of cytotoxic lymphocytes and promoting recruitment, differentiation, and function of immunosuppressive immune cells (25). Separately, it is worth highlighting the unique immune microenvironment of IDH-mutant gliomas that produce the oncometabolite R-2-hydroxyglutarate. These tumors are characterized by reduced infiltration of T lymphocytes, macrophages, and neutrophils, and R-2-hydroxyglutarate was shown to impair effector functions of dendritic cells, T cells, and NK cells but promote immunosuppressive phenotype of macrophages (26, 27). Finally, glioblastoma-related systemic immunosuppression involves reduced lymphocyte counts (lymphopenia), increased neutrophil, MDSCs, and Tregs counts, and defective functions of antigen-presenting, helper, and effector immune cell subsets due to altered expression of different soluble and membrane proteins (28–36).
The goal of immunotherapy is to break immunological tolerance, enhance antigen presentation, re-engage innate and adaptive immune effectors in the tumor, and establish a long-term persistent population of cytotoxic tumor-specific memory T cells. Treatment efficacy may critically depend on tumor immunogenicity and the baseline systemic immunological competence of a patient, including the counts, ratios, and functional states of different circulating and tumor-infiltrating immune cell subsets. Differentiating between immunological responders and non-responders before immunotherapy/oncolytic virotherapy is clinically relevant. It is very likely that patients with low systemic immune suppression may benefit from immunotherapy/oncolytic virotherapy much better than severely immunocompromised patients who might be non-responsive to any extent. Is the response to immunotherapy/oncolytic virotherapy correlated with the degree of lymphopenia, neutrophilia, distinct immune cell subset ratios, or other systemic and local tumor immune-related signatures or biomarkers? In clinical trials, immunotherapeutics are typically tested concurrently with, or after, standard therapy. However, standard therapy-related (iatrogenic) systemic immunosuppression, long-lasting lymphopenia, and contraction of T cell receptor repertoire diversity may negatively impact the efficacy of immunotherapy/oncolytic virotherapy. In this review, we comprehensively discuss standard therapy-promoted immunotoxicity and its implications for immunotherapy/oncolytic virotherapy clinical trials. In our accompanying review in Frontiers in Immunology (Systemic and local immunosuppression in glioblastoma and its prognostic significance), we focused on immunological data from patients with glioblastoma/gliomas before standard therapy, namely, tumor-related immunosuppression at baseline and the prognostic significance of different circulating and tumor-infiltrating immune cell subsets [CD4+ and CD8+ T cells, natural killer (NK) cells, neutrophils, macrophages, MDSCs, and Tregs], including neutrophil-to-lymphocyte ratio (NLR), and specifically discussed the immune landscape of IDH-mutant gliomas, proneural, classical, and mesenchymal molecular subtypes, as well as the features of immune surveillance of the brain.
2 Standard therapy-related immunosuppression in glioblastoma
2.1 A low post-treatment total lymphocyte count is a prognostic factor for shorter survival
Standard therapy is a major cause of immune deficiency in patients with glioblastoma, exacerbating tumor-related systemic immunosuppression. Analysis of peripheral blood before, during, and after completing chemoradiotherapy showed that standard therapy may significantly affect diverse immune cell subsets (Table 1) (37–42). Patients with recurrent glioblastoma have lower levels of total immune effector cells, including circulating CD3+, CD4+, and CD8+ T cell subsets, B cells, and NK cells, with reductions in naïve, central memory, and effector memory subsets, activated T cells, and proliferating Ki67+ cells, than patients with newly diagnosed glioblastoma (43). Interestingly, despite its lymphocyte-depleting effect, standard therapy (34, 37, 38, 40) or a dose-intensified temozolomide regimen (44, 45) increases the proportion of circulating Tregs.
The total lymphocyte count (TLC) is classified as normal (≥1000 cells/mm3) or abnormal (<1000 cells/mm3), and grade 3/4 lymphopenia (<500 cells/mm3 for CD8+ T cells and <200 cells/mm3 for CD4+ T cells) is considered severe. Although the number of affected patients with grade 3/4 versus 1/2 lymphopenia varied significantly between studies, lymphopenia was among the most frequent hematologic adverse effects in patients with glioblastoma/gliomas during or after standard radio- and/or chemotherapy (46–54). A lower TLC before therapy predicts severe lymphopenia during treatment (46, 48, 55, 56). Furthermore, increasing age is an important factor that significantly contributes to the severity of therapy-induced lymphopenia; in the elderly group of patients (median age, 71 years), only 57% (out of n=72) had a normal baseline TLC (50) versus 97% (out of n=336) in the adult group (median age, 58 years) (57). Older age is independently associated with severe lymphopenia in patients with glioblastoma (55). Sex is another confounding factor of therapy-related lymphopenia. In two large cohort studies (n>700 and n>2000), women were found to be more likely to develop lymphopenia than men (29, 58). Remarkably, 21-47% of patients with glioblastoma/gliomas treated with radiochemotherapy developed long-lasting grade 3/4 lymphopenia (29, 47–52, 58–60), although there were studies reporting a less frequent occurrence of grade 3/4 lymphopenia during or after standard therapy (2.9-10%) (53, 61, 62). Moreover, patients with recurrent glioblastoma exhibited a significantly lower TLC compared with patients with newly diagnosed glioblastoma (63), and lymphopenia reached 76.5% for a standard temozolomide regimen in patients with recurrent glioblastoma (64). The post-treatment TLCs remained significantly lower than the baseline counts, even after 12 months, in adult (52) and elderly (50) patients with glioblastoma and in children with central nervous system tumors (65). Patients who developed severe CD4+ lymphopenia during standard therapy experienced higher hospitalization and infection rates (66, 67). However, the major cause of death in patients with grade 3/4 lymphopenia was tumor progression rather than infection (67).
It is widely accepted that lymphocytes are key effector cells in the immune response to tumors, and that anti-cancer T cell-mediated immunity is key to improving survival outcomes, although the contribution of other immune cell types should not be underestimated (68). Unsurprisingly, standard therapy-related grade 3/4 lymphopenia was associated with shorter overall survival in multivariate analyses (Table 2) (50, 56, 57, 60, 67) and meta-analysis (69). However, in Byun et al.’s study (57), severe lymphopenia was an independent prognostic factor only when the authors included patients with grade 3/4 lymphopenia at 3-month time of therapy but not when patients were included at any time point within 3 months of therapy. Similarly, Le Rhun et al. (29) reported that grade 3/4 lymphopenia during concomitant radiochemotherapy but not during maintenance temozolomide chemotherapy was significantly associated with inferior overall survival in univariate and multivariate analysis. Deng et al. (29) reported that lymphopenia during standard therapy was associated with overall survival in a multivariate analysis; however, no association was found between overall survival and lymphopenia at other time points (preoperative, pre-radiotherapy, or first recurrence). It may be concluded that the recovery rate from severe lymphopenia and the use of different time points to define treatment-related lymphopenia may be important modifiers of the prognostic power of TLC (57).
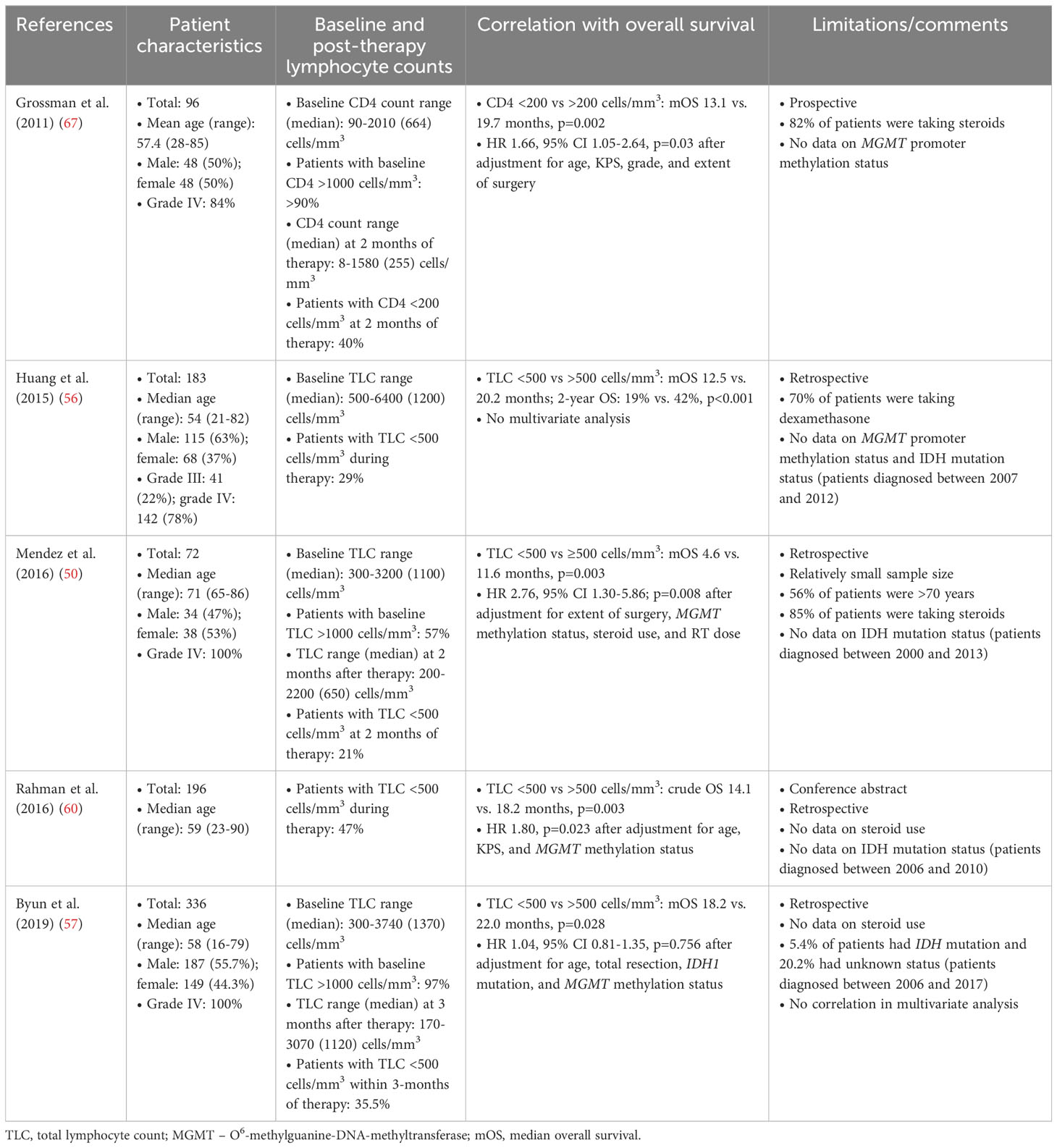
Table 2 Standard therapy-related grade 3/4 lymphopenia is a predictor of shorter overall survival in patients with glioblastoma/gliomas.
2.2 Radiation-induced lymphopenia is a prognostic factor for mortality in virtually all solid cancers
Lymphocytes are one of the most radiation sensitive cells in the body (70). T lymphocytes are more sensitive to radiation than neutrophils, monocytes, NK cells, dendritic cells, or macrophages (71, 72). Only 30-50% of the T cells were found viable 48-72 hours post-exposure ex vivo to a single dose of 2 Gy (73). Exposure to low single dose radiation (0.3-0.5 Gy) also decreased the number of peripheral blood T lymphocytes (73). It also should be noted that not only total lymphocyte counts but also lymphocyte diversity and activity are affected by radiation (72). For instance, helper T cells (CD4+) are more radiosensitive than cytotoxic T cells (CD8+) (72). A comprehensive discussion of the effects of radiation on various immune cell populations in humans and mice is presented elsewhere (72).
A significant drop in the peripheral lymphocyte counts after extracorporeal irradiation of the circulating blood was observed in calves (74). Similarly, radiation given only to circulating blood within a dialysis unit in humans awaiting kidney transplants produced severe and long-lasting lymphopenia (75, 76). The standard radiotherapy regimen for glioblastoma (60 Gy delivered in 30 fractions to a partial brain field) may cause toxicity in up to 98% of circulating lymphocytes (77). The development of severe lymphopenia after radiation without concurrent chemotherapy or steroids use has been reported in patients with brain, head and neck, breast, pancreatic, esophageal, cervical, uterine, and lung cancer (52, 78). Notably, according to systematic reviews and meta-analyses, post-radiation lymphopenia is associated with shorter survival in pancreatic and lung cancer (79–81), head and neck and esophageal cancer (79, 82–84), urological cancer (85), nasopharyngeal and cervical cancer (47) and many other solid tumors (69, 78, 79, 86–88). In agreement, a meta-analysis including 16 cancer types supports that radiation-induced lymphopenia is a significant prognostic factor for mortality in virtually all solid cancers (79). Taken together, radiotherapy per se is a strong and primary inducer of lymphopenia regardless of the type of chemotherapy received by cancer patients.
2.3 Chemotherapy and corticosteroids may exacerbate radiation-induced lymphopenia
Temozolomide and dexamethasone are also lymphodepleting agents that may exacerbate radiation-induced lymphopenia in patients with glioblastoma/gliomas (43, 59, 89). In patients with grade II-III glioma (n=151), concurrent radiochemotherapy and the duration of adjuvant chemotherapy were significantly associated with lymphopenia in multivariate analysis (62). In a cohort of 39 patients with advanced metastatic neuroendocrine tumors who received temozolomide (no radiation, minimal use of concurrent steroids by 6/39 patients), the incidence of severe lymphopenia was 46% after 4 months and >60% after 6 months of temozolomide treatment, and persisted in at least 30% of patients during the 12 months following treatment discontinuation (90). The majority of patients with melanoma (>60-70%) developed lymphopenia after serial treatment cycles with different temozolomide dosing regimens and schedules (91–93). The development of lymphopenia by temozolomide can be caused by the negligible expression of MGMT and/or multidrug resistance proteins in peripheral blood lymphocytes. It should be noted that not only temozolomide may cause or promote long-term lymphopenia. For instance, in patients with primary breast cancer receiving other types of chemotherapy regimens without radiotherapy and steroids, CD4+ T cells remained significantly depleted even 9 months post-chemotherapy (reaching medians of only 60% of initial levels) (94).
2.4 Steroid-induced immunosuppression
Dexamethasone has comparatively low mineralocorticoid properties, high glucocorticoid potency, and a long biological half-life. However, corticosteroids exhibit adverse systemic effects, the most common of which are immunosuppression, hyperglycemia, hypertension, osteoporosis, myopathy, diabetes, and thromboembolic events (95, 96). The severity of these adverse effects is usually correlated with the total daily dose and duration of steroid application.
In vitro and ex vivo studies have shown that steroids alter the maturation of dendritic cells, inducing tolerogenic cells that express low levels of major histocompatibility complex, costimulatory molecules, and cytokines, resulting in hypo-responsiveness and an anergic state in naïve and memory T cells primed by such dendritic cells (97–100). Steroids impair T cell receptor signaling and the expression of many cytokines, chemokines, and adhesion molecules, affecting the development, polarization, activation, and migration of T cells, and promoting the formation of Tregs (97–100).
In chemoradiotherapy- and resection-naïve patients with glioblastoma, baseline lymphopenia is more frequently observed in dexamethasone-treated patients than in dexamethasone-naïve patients (28). In general, dexamethasone use is significantly associated with lower lymphocyte counts in patients with glioblastoma (43, 89). Dexamethasone use >2 versus ≤2 mg/day was independently associated with severe lymphopenia (3-month rate: 43.7% versus 19.8%; p<0.001) (101). To elucidate how short-term dexamethasone treatment affects the immune system of patients with glioblastoma, Gustafson et al. analyzed peripheral blood samples collected perioperatively and before radiochemotherapy (102). The CD4+ cell count was significantly lower in dexamethasone-treated patients than in dexamethasone-naïve patients (102). Changes in the expression of costimulatory and antigen-presenting molecules in peripheral monocytes were more pronounced in patients receiving dexamethasone (102). Patient-derived CD14+ monocytes had an immunosuppressive phenotype with defective direct T cell stimulation and dendritic cell differentiation capacity (102). In another study, dexamethasone treatment before glioblastoma resection affected the main immune cell populations, including CD4+ and CD8+ T cells, CD66b+ neutrophils, CD14+ monocytes, non-Vδ2 γδT cells, and NK cells (especially CD56high) (103). Similarly, analysis of pre-surgery blood samples revealed that patients with glioma (n=139) who received dexamethasone (45.3%) had the lowest B, NK, monocyte, CD4+, and CD8+ T cell, and total lymphocyte counts (104). Cook et al. examined peripheral blood samples from patients with malignant pleural mesothelioma who received 4 mg dexamethasone thrice prior to undergoing standard chemotherapy (105). The authors observed substantial immunomodulatory effects in response to dexamethasone administration, particularly affecting CD4+ and CD8+ T cells and dendritic cell subtypes. The proportion of Tregs did not change; however, a significant increase in their proliferation and activation was observed (105). In contrast, the absolute number of Tregs was significantly lower in dexamethasone-treated patients with glioblastoma than in normal controls or dexamethasone-untreated patients (102, 103).
Moreover, patients with glioblastoma receiving steroids had higher counts of blood MDSCs than those who did not receive steroids (35) or were on steroids for a shorter period (36). Dexamethasone has been also shown to induce MDSCs in preclinical transplantation models, and its dose positively correlated with the absolute number of MDSCs in transplant recipients (106). In addition, patients with glioblastoma receiving steroids had significantly higher neutrophil counts (58, 69, 89, 103, 104, 107). However, other research groups found no significant difference in neutrophil counts in patients receiving or not receiving steroid therapy (108) or revealed only a weak correlation between the dexamethasone dose and NLR (109). Finally, perioperative corticosteroid treatment impaired tumor-infiltrating dendritic cells in patients with newly diagnosed gliomas (110). Altogether, in accordance with in vitro and ex vivo studies, dexamethasone (without radiochemotherapy) adversely affects the immune system of patients with cancer.
2.5 Dexamethasone use is a prognostic factor for shorter survival
Steroid use as a negative prognostic indicator of survival in patients with glioblastoma/gliomas was recognized approximately three decades ago (111, 112) and confirmed by modern retrospective research (Table 3) (9, 55, 113–119). In congruence, a large cohort study (n=2002) (58) and meta-analysis (120) reported that steroid use was associated with worse overall survival. However, some studies have demonstrated no association between steroid use and outcomes in patients with newly diagnosed glioblastoma in univariate or multivariate analyses (50, 67, 107, 121–124). In some retrospective studies, steroid use was associated with worse overall survival in patients treated with radiotherapy only but not in those treated with both radiation and chemotherapy (9, 116), and vice versa (116). Nevertheless, when the patient cohorts were analyzed as a whole, dexamethasone use was associated with a negative prognosis (Table 3).
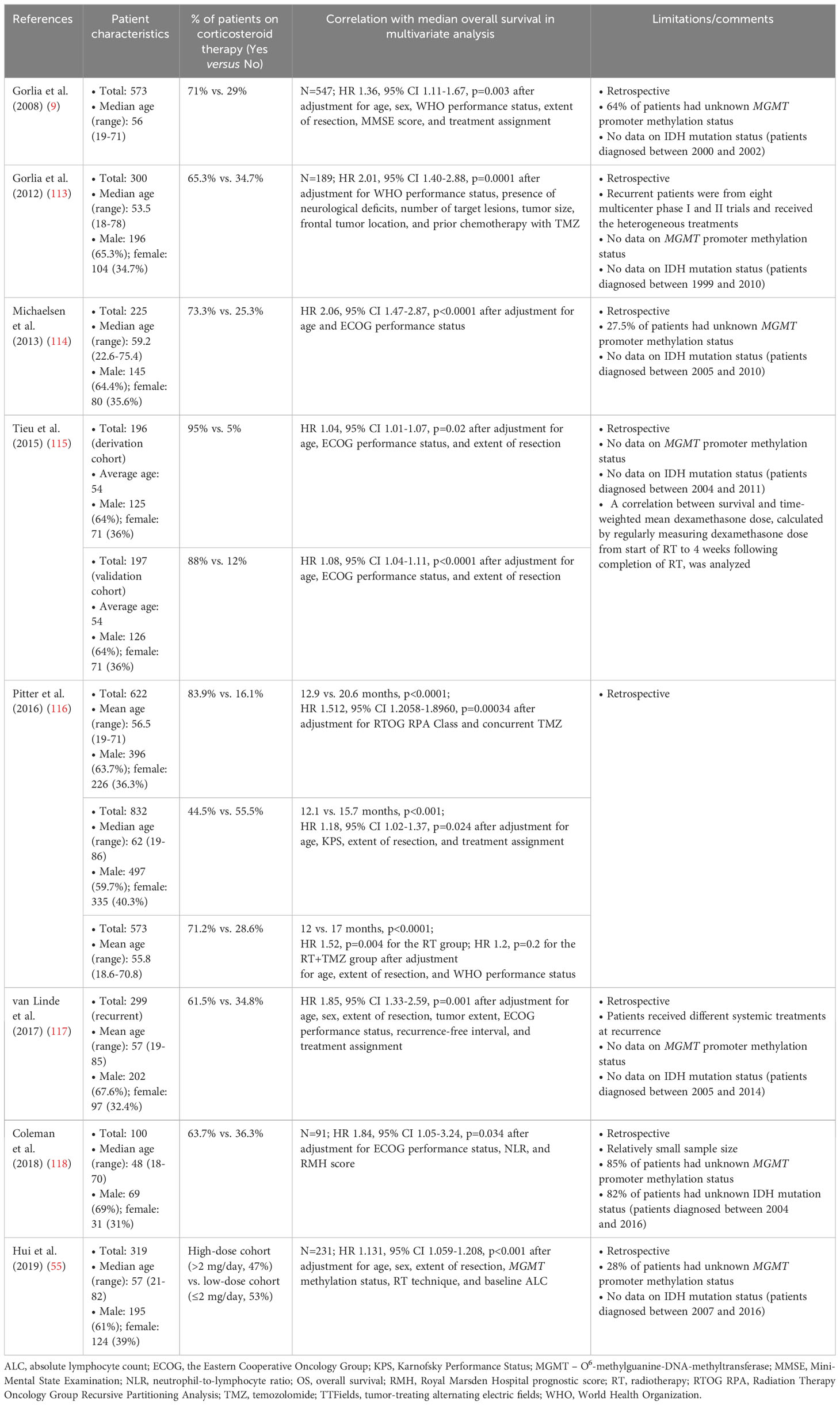
Table 3 The use of dexamethasone is a predictor of shorter overall survival in patients with glioblastoma/gliomas.
In addition, multivariate analyses revealed that there were negative associations between baseline corticosteroid use and overall survival in patients with glioblastoma receiving checkpoint inhibitors (125, 126). In systematic reviews and meta-analyses, administration of steroids for supportive care (e.g., disease-related symptoms) or brain metastases was associated with significantly worse overall survival in non-small cell lung cancer and melanoma patients receiving immune checkpoint inhibitors (127, 128). In contrast, steroids used to mitigate adverse events did not negatively affect overall survival (128). However, steroid timing for immune-related adverse events may be associated with survival in patients receiving immune checkpoint inhibitor therapy. In large multicenter retrospective cohort study (n=20163), systemic steroids for immune-related adverse events were associated with significantly improved survival compared with those who received steroids for other reasons or no steroid treatment (mOS, 21.3 versus 13.6 versus 15.8 months; P<0.001) (129). However, among those who received steroids for immune-related adverse events, early steroid use (<2 months after immune checkpoint inhibitor initiation) was associated with reduced relative survival benefit in comparison to later steroid use, regardless of immune checkpoint inhibitor continuation or cessation following steroid initiation (mOS after immune checkpoint inhibitor cessation, 4.4 versus 16.0 months; mOS after immune checkpoint inhibitor continuation, 16.0 versus 29.2 months; P<0.001) (129).
2.6 Can corticosteroids compromise survival in glioblastoma?
Dexamethasone treatment significantly decreased survival in irradiated glioma-bearing mice in a genetically engineered mouse model of glioma (116) and increased proliferation, invasion, and angiogenesis in a human glioma stem cell-derived orthotopic tumor model (130). However, in a pediatric low-grade glioma cohort (n=191), no significant difference was observed in the short- or long-term tumor growth rates or in the progression-free survival of patients treated with or without perioperative dexamethasone, irrespective of gross total or incomplete resection (131). The negative correlation between dexamethasone use and overall survival reported in most large cohort studies (Table 3) might be due to less extensive surgery, more aggressive tumor growth, and edema in patients requiring steroid treatment (116, 132). A higher daily steroid dose (>2 mg/day) in comparison to a lower daily steroid dose (≤2 mg/day) was independently associated with median overall survival (n=319, 12.6 versus 17.9 months, p<0.001); however, in patients with gross total resection, the steroid dose was not prognostic for overall survival (55). Moreover, overall survival of patients receiving ≤4 mg/day dexamethasone did not differ significantly from that of patients who did not receive steroids (55). These data suggest that tumor size might still be a dominating confounding factor in the association between dexamethasone use and overall survival. However, in patients with recurrent glioblastoma receiving >4.1 versus ≤4.1 mg/day dexamethasone and treated with tumor-treating alternating electric fields (TTFields) (n=120), median overall survival was 4.8 and 11.0 months (p<0.0001), respectively, and tumor size did not differ statistically between patient cohorts (119). Furthermore, the analysis of three large independent cohorts (total n>2000) confirmed a negative association between dexamethasone use and overall survival after adjustment for age, extent of resection, and performance status (116). Overall, there can be confounding factors other than tumor size that modify the prognostic significance of steroid use. Steroid treatment-related systemic immunosuppression or other steroid-related adverse effects, such as the deregulation of blood glucose levels, may also affect patient survival. Hyperglycemia before surgery, during radiation, or radiochemotherapy was independently associated with poorer progression-free and overall survival in patients with glioblastoma after adjusting for the mean daily dexamethasone dose and other prognostic variables (115, 133–138). As each patient receives personalized dexamethasone treatment, it is not possible to prospectively determine how dexamethasone use, its daily dose, and the duration of treatment affect cancer patient survival.
2.7 Lymphopenia is significantly associated with response and survival outcomes in patients with advanced cancer on immune checkpoint inhibitor therapy
Patients with solid tumors treated with programmed cell death 1 (PD-1) checkpoint inhibitors (n=167) with absolute lymphocyte counts >2000 cells/μl at baseline had an increased risk of immune-related adverse events on multivariate analysis (139). At the same time, patients with lymphopenia at baseline and persistent lymphopenia at three months (largely radiation-related) had a shorter time to progression compared to those who had baseline lymphopenia but recovered with absolute lymphocyte counts >1000 cells/μl at 3 months (HR 2.76, p<0.05) (139). These data suggest that lymphopenia may dramatically reduce the incidence of immune-related adverse effects but is associated with an efficiency of immune checkpoint inhibitors. In support, pretreatment absolute lymphocyte count (<600 cells/μl) was significantly associated with response to PD-1 inhibitors in patients with head and neck squamous cell carcinomas (n=34) (140). In another study, lymphopenia (<1000 cells/μl) upon initiation of PD-1 inhibitor nivolumab was not associated with poorer survival in patients with head and neck squamous cell carcinomas (n=100), but persistent lymphopenia under nivolumab was associated with poorer overall survival in multivariate analysis (HR 3.96, 1.19-13.17, p=0.034) (141). Similarly, patients with metastatic melanoma (n=116) with a normal lymphocyte count at baseline but who developed lymphopenia during immune checkpoint inhibitor therapy had significantly shorter progression-free survival (13.3 versus 16.9 months, p=0.025) and overall survival (28.1 versus 36.8 months, p=0.01) compared with patients who did not develop (142). In a prospective, observational study of patients with advanced non-small cell lung cancer (n=123) treated with immune checkpoint inhibitors, high absolute lymphocyte count (>1.01×109/L) and absence of liver metastases were significantly associated with a durable clinical benefit defined as progression-free survival >6 months (143). Finally, a meta-analysis of patients with lung cancer who were treated with immune checkpoint inhibitors confirms that lymphopenia is associated with poor survival (144).
3 Implications of standard therapy-related immunosuppression for immunotherapy or oncolytic virotherapy clinical trials
3.1 Standard therapy-related immunosuppression is a barrier to immunotherapies
It is rationalized that standard radio/chemotherapy is important for reducing tumor mass and increasing neoantigenic tumor load, and that it can enhance the immunogenicity of the tumor microenvironment by inducing immunogenic cell death, which promotes anti-tumor immunity (145). On the other hand, radiotherapy induces systemic and intratumoral lymphopenia (146). Furthermore, glioblastoma cells are intrinsically resistant or acquire resistance to chemotherapy, which may promote aggressiveness and enhance their immunosuppressive properties (101, 147–149). Temozolomide is not a potent inducer of bona fide immunogenic cell death in human glioblastoma cells (150–152) and is not included in the shortlist of anti-cancer chemotherapeutics with the capacity to consistently induce immunogenic cell death (153). Although ionizing radiation can induce immunogenic cell death, it is critical to realize that conventional radiation treatment trigger a combination of cell death processes, such as apoptosis, necrosis, autophagy, mitotic catastrophe, and senescence, eliciting both immune-activating and suppressing responses (154). Additionally, it is widely recognized that immunogenic cell death cannot drive anti-cancer immunity in the presence of general immunological defects. A growing list of intrinsic and acquired immune-related resistance mechanisms that limit the efficient exploitation of immunogenic cell death in cancer immunotherapy has emerged (153, 155, 156). In most studies, comparative analyses of newly diagnosed and recurrent (matched or unmatched) glioblastoma samples revealed no significant differences in the density of immune cell subsets or exhaustion profiles of tumor-infiltrating T cells (157–165). On the contrary, monocyte-derived tumor-associated macrophages are incessantly recruited in recurrent glioblastoma, and the macrophage/microglia ratio is increased in both the central and marginal areas of the recurrent tumors in comparison to newly diagnosed glioblastoma (159, 166). The lack of significant changes in the immune infiltration profiles of cytotoxic lymphocytes in recurrent glioblastoma questions the putative positive immunomodulatory role of standard radiochemotherapy within the tumor microenvironment.
Furthermore, T cell receptor sequencing demonstrated a contracted T cell receptor repertoire diversity concomitant with an increased frequency of activated memory T cells among tumor-infiltrating lymphocytes in patients with recurrent glioblastoma after standard therapy (63). The youngest naïve T cells are continuously exported from the thymus as “recent thymic emigrants” and maintain T cell diversity in the periphery with a particularly important contribution in adults recovering from lymphopenia (167, 168). There is emerging evidence that thymic emigrants can mount robust immune responses (169). CD8+ thymic emigrants have been found to account for the majority of tumor antigen-binding cells in peripheral blood mononuclear cells in patients with glioblastoma (170). In patients vaccinated with autologous tumor lysate-pulsed dendritic cells, the levels of expanding CD8+ recent thymic emigrants strongly correlated with vaccine-elicited cytokine responses and predicted survival outcomes (170). As the thymus significantly degenerates with age (thymus involution) (65, 171), in adult and elderly patients, the restoration of heterogeneous populations of T cells and re-establishment of T cell immunocompetence after standard therapy is a slow and frequently incomplete process, which may progress primarily through thymic-independent peripheral expansion of the remaining mature T cell populations with reduced T cell receptor repertoire diversity (63, 172). Therapy-related contraction in the T cell receptor repertoire diversity may potently decrease the chances of a successful anti-tumor response.
Since immunotherapeutics are tested in patients with glioblastoma concurrently with or after standard therapy, standard therapy-related immunosuppression presents a barrier to the success of immunotherapies (86). It has been repeatedly reported that systemic chemotherapy (173–177) or CD4+/CD8+ T cell depletion (150, 178–186) abrogates the survival benefit of immunotherapies or oncolytic virotherapy in preclinical models. Therefore, it appears counterintuitive to combine lymphotoxic radiotherapy/chemotherapy with immunotherapy or virotherapy. Pellegatta et al. reported that standard therapy significantly decreased CD8+, CD4+, and NK cell counts in patients with glioblastoma (n=24), and the administration of adjuvant temozolomide had a negative effect on the increase of the CD8+ T cell subset and the generation of CD8+ T cell-associated anti-tumor memory elicited by dendritic cell vaccination (42). A negative effect on anti-tumor immunity of temozolomide administration as an adjuvant to dendritic cell vaccination in patients with recurrent glioblastoma was also observed in another clinical study (187). It is also noteworthy that in patients with newly diagnosed MGMT-unmethylated glioblastoma, personalized neoantigen peptide vaccines induced circulating neoantigen-specific T cell responses and a significant increase in infiltrating CD8+ T cells at relapse only in those who did not receive dexamethasone during vaccine priming (188). Conversely, the potential positive interaction of radio/chemotherapy with immunotherapy or oncolytic virotherapy was also demonstrated in many immunocompetent rodent models, and the rationale for their combination and possible limitations are intensively debated in the literature. A few studies have argued that immunosuppression does not prevent immune responses induced by a vaccine in patients with glioblastoma (189) and have pointed to a putative positive immunomodulatory role of temozolomide in dendritic cell vaccination or multimodal immunotherapy (190, 191). However, no large, well-designed randomized controlled trials have been conducted to prove the positive effects of the addition of immunotherapy or oncolytic virotherapy to standard therapy, and only minor subsets of patients have benefited from conducted clinical trials (3–7).
3.2 The need for shifting the treatment paradigm
An increasing number of studies have reported an association between the radiotherapy dose/volume and lymphopenia in glioblastoma (56, 77, 192, 193). The severity of radiation-induced lymphopenia depends on the technique of radiotherapy, fraction number (fractionation regimen), dose per fraction (irradiation dosage), field size, and other variables (78, 194–197).
Patients with glioblastoma treated with moderately hypofractionated radiotherapy (58.5 Gy in 25 fractions, n=78) had a significantly reduced rate of grade ≥2 lymphopenia at 6 months post-radiotherapy in comparison with conventionally fractionated radiotherapy (60 Gy in 30 fractions, n=145), with no difference in overall survival between groups (27.2 versus 26.6 months) (198). In addition, in patients with pancreatic cancer, hypofractionated radiotherapy in comparison to standard radiotherapy also considerably reduced the risk and severity of radiation-induced lymphopenia (78, 199). In a study of patients with glioblastoma aged ≥60 years (n=100), there was no difference in survival between those receiving standard radiotherapy (60 Gy in 30 fractions over 6 weeks) or short-course radiotherapy (40 Gy in 15 fractions over 3 weeks) (200). In congruence, a meta-analysis of controlled trials testing the impact of radiotherapy hypofractionation on the survival of patients with glioblastoma reported comparable survival outcomes between hypofractionation and standard radiotherapy (201).
Patients with glioblastoma receiving intensity-modulated radiotherapy (IMRT, n=150) had a significantly decreased incidence of severe lymphopenia (20% versus 37%; p=0.005) compared with patients treated with three-dimensional conformal radiotherapy (3D-CRT, n=186) (57). Compared with standard-field radiotherapy (n=164), limited-field radiotherapy (n=46) was associated with less grade 3/4 lymphopenia after radiochemotherapy, though not statistically significant (15.5% versus 33.8%; p=0.12), and did not adversely affect progression-free and overall survival in patients with glioblastoma (193). In a prospective, randomized phase II glioblastoma trial, a comparison of the Radiation Therapy Oncology Group (RTOG) and The University of Texas MD Anderson Cancer Center (MDACC) radiation treatment guidelines revealed that overall survival was superior in the MDACC group (17 versus 12 months, p=0.015, n=25 per arm) (192). In locally advanced pancreatic cancer, 13.8% and 13.6% of patients in the stereotactic body radiation therapy group versus 71.7% and 46.0% of patients in the conventional chemoradiation therapy group had severe lymphopenia at 1 month and 2 months, respectively, after starting radiotherapy (202).
In a randomized phase II glioblastoma trial of proton versus X-ray (photon) therapy with concurrent temozolomide, the rates of grade 3/4 lymphopenia occurrence were lower for proton therapy (4/28, 14%) than for X-ray (photon) therapy (22/56, 39%, p=0.024) (203). Grade 3/4 lymphopenia was significantly associated with baseline absolute lymphocyte counts, whole-brain mean dose, and brain volumes receiving 5–40 Gy (203). Similarly, in esophageal cancer patients undergoing neoadjuvant chemoradiotherapy, a greater proportion of patients in the intensity modulated radiation therapy group (55/136, 40.4%) developed grade 4 lymphopenia compared with patients in the proton therapy group (24/136, 17.6%, P<0.0001) (204). On multivariate analysis, proton therapy was significantly associated with a reduction in grade 4 lymphopenia risk (204). In a retrospective nonrandomized study of the comparative effectiveness of proton (n=391) and photon (n=1092) therapy as part of concurrent chemoradiotherapy for locally advanced cancer, proton chemoradiotherapy was associated with significantly reduced acute adverse events but with similar disease-free and overall survival (205). Numerous comparative studies, including prospective and/or randomized, support the hypothesis that proton therapy is associated with improved toxicity and results in outcomes at least equivalent to those of photon therapy (206, 207). Results from the large randomized NRG BN001 (NCT02179086) phase II trial comparing dose-escalated photon IMRT or proton beam radiation therapy versus standard-dose radiation therapy and temozolomide in treating patients with newly diagnosed glioblastoma are awaited. Moreover, three phase III trials directly comparing proton therapy to photon therapy across a broad variety of malignancies are currently accruing (206).
Taken together, although all these studies should be currently considered as hypothesis-generating rather than conclusive, and require multi-center validation in larger cohorts of patients, they clearly suggest that modifications in the standard radiation treatment paradigm might reduce negative effects on the immune system without compromising overall survival, potentially strengthening the therapeutic efficacy of prospective immunotherapies (208).
Temozolomide exerts a negligible therapeutic effect in patients with glioblastoma with an unmethylated MGMT promoter (209) and has been omitted from first-line therapy in some clinical trials (87). Temozolomide therapy could be excluded for patients with unmethylated MGMT promoter in immunotherapy/oncolytic virotherapy trials. The administration of immunotherapeutics as neoadjuvants (210–212) and local administration of therapeutics to the brain should also be considered (213).
Immunotherapy trials generally restrict steroid use at enrollment. Interestingly, the proportion of patients with glioma who were steroid-free at the end of chemoradiotherapy varied significantly in different studies (e.g., 78%, 55.5%, 28.6%, 27%, and 16%) (214). There is no standard treatment regimen for the steroid dexamethasone in neuro-oncology (215), which means that the choice of daily dose, duration of treatment, and tapering schemes are adapted for each patient. Body weight or patient age were not considered a modifier of the daily dose (96). It is not recommended to use high doses of dexamethasone for prophylaxis in asymptomatic patients; instead, the lowest dose must be considered to balance the desired effect and multiple adverse effects (216, 217). To avoid toxicity associated with prolonged exposure, dexamethasone should be tapered after achieving maximum clinical benefit with decrements of the previous dose until the lowest dose needed to maintain optimum neurological function is reached. Since it is important to control steroid use in patients with gliomas, the Response Assessment in Neuro-Oncology (RANO) Working Group developed consensus recommendations on steroid use endpoints in clinical trials in both adults and children with brain tumors (218). Nevertheless, based on accumulating clinical evidence, a less toxic and comparably effective alternative to dexamethasone is urgently required to treat edema in patients with brain tumors.
The search for methods to improve lymphocyte count recovery following prolonged lymphopenia is another important direction for clinical research (88). In patients with lymphopenia before standard therapy, T cells are sequestered in the bone marrow owing to decreased levels of sphingosine-1-phosphate receptor 1 (S1PR1) on the surface of T cells (28). Pharmacological stabilization of S1PR1 on the T cell surface has been suggested as a potential strategy to ameliorate bone marrow T cell sequestration and reverse baseline lymphopenia (28). However, S1PR1 plays a role in both adaptive and innate immune responses by regulating the recruitment, trafficking, and function of T cells and most innate immune cells (219). Moreover, S1PRs are expressed in glioblastoma and sphingosine-1-phosphate signaling is active in glioblastoma cells, contributing to the pathobiology of brain tumors (220). It is likely that modulators that stabilize S1PR1 might exert pleiotropic effects, including potentially adverse pro-tumorigenic effects.
In patients with glioblastoma and lymphopenia after standard therapy, neither the total lymphocyte count nor CD4+ cell recovery was augmented by the reinfusion of autologous lymphocytes harvested using apheresis prior to therapy (40). A recent prospective correlative study of patients with glioblastoma after radiochemotherapy (n=20) suggested that the expansion of circulating MDSCs due to increased myelopoiesis in the bone marrow may be associated with lymphopenia, which was supported by preclinical in vivo data (221). Moreover, in patients with glioblastoma and lymphopenia, a compensatory increase in the concentration of interleukin-7 (IL-7) and interleukin-15 (IL-15) was not observed (39). IL-7 and IL-15 are essential for homeostasis of circulating T lymphocytes (88). In a prospective cohort of patients with hepatocellular carcinoma (n=98), post-radiotherapy IL-7 levels were significantly positively correlated with total lymphocyte counts at 2 months (222). hIL-7-hyFc is a homodimeric IL-7, fused to the hybridizing IgD/IgG4 immunoglobulin domain. hIL-7-hyFc was well-tolerated and increased absolute lymphocyte counts in healthy humans after a single administration (223). In patients with recurrent glioblastoma (n=18), treatment with rhIL-7-hyFc restored and maintained total lymphocyte counts without serious toxicity and irrespective of steroid use during treatment with salvage therapies, such as temozolomide and/or bevacizumab (224). A large randomized controlled trial is required to validate the clinical benefits of rIhL-7-hyFc treatment in cancer. In general, based on available clinical data for the treatment of severe lymphopenia in cancer and non-cancer patients, rhIL-7 is a potent therapeutic candidate for immune reconstitution (88).
3.3 Detailed blood and/or tumor immunophenotyping may be valuable for immunotherapy and oncolytic virotherapy trials
In dendritic cell vaccinated patients with glioblastoma, CD8+ T cells levels (170, 225), the maximum count of CD3+/CD4+ T cells (226), the increased vaccination/baseline ratio of NK cells (227) and fold change in Tregs frequency (228) in peripheral blood, post-vaccination IFN-γ T cell responsiveness (225, 229, 230), higher tumor-infiltrating lymphocyte density (231), a low PD-1+/CD8+ ratio in tumor tissue (232), a low B7-H4 expression level in tumor tissue (233), a low cytotoxic T-lymphocyte-associated antigen 4 (CTLA-4) expression level on T cells (228), and a low programmed cell death 1 ligand 1 (PD-L1) expression level on myeloid cells (234) predicted therapy responses and/or were correlated with overall survival. In a phase II trial of a whole-cell lysate dendritic cell vaccine combined with standard therapy for newly diagnosed glioblastoma, patients with a low PD-1+/CD8+ ratio in tumor tissue had a median overall survival of 61 versus 20.7 months for patients with a high PD-1+/CD8+ ratio (232). Similarly, in a phase II trial of autologous heat shock protein peptide vaccine combined with standard therapy in newly diagnosed glioblastoma, a median overall survival in patients with low PD-L1 expression on myeloid cells was 44.7 versus 18.0 months for patients with high PD-L1 expression (234). There have also been attempts to identify responders by hierarchical clustering of a set of post-vaccine lymphocyte functional parameters (235). However, no prospectively validated robust predictive biomarkers for immunotherapy in patients with glioblastoma have been established.
The correlation between immune cell subsets, signatures, or markers and response to immunotherapy/oncolytic virotherapy and overall survival should be monitored in clinical trials to identify new or validate the proposed immunological-based predictive and prognostic variables. The first prospective, explorative, and observational IMMO-GLIO-01 trial (NCT02022384) was launched to examine the immune status of approximately 50 patients with glioblastoma or anaplastic astrocytoma during standard therapy. As stated in the goals of the study, it would be useful to estimate individual responses, stratify patients, and find suitable time points for the inclusion of additional immunotherapy (236). Since only 21% of patients with recurrent glioblastoma treated with the recombinant oncolytic poliovirus PVSRIPO experienced long-term survival (>3 years), and 79% of patients did not respond (237), researchers developed a robust method for cellular immunome monitoring to identify biomarkers that predict the response to virotherapy (238). This method is exploited in a phase II study in patients with recurrent glioblastoma (NCT02986178) to assess baseline and therapy-mediated changes in local and peripheral cellular immunomes.
4 Conclusion
Delivering the right combinations with the right dosages at the right place during the right time is a difficult task in cancer treatment but may be highly relevant to immunotherapeutics. Although the tumor itself is immunosuppressive, clinical data provide compelling evidence that standard therapy exacerbates immune deficiency in patients with glioblastoma by promoting lymphopenia and systemic immunosuppression (Figure 1A). Prior to the initiation of standard therapy, patients with glioblastoma/gliomas display varying degrees of immunosuppression but rarely have severe lymphopenia. In contrast, clinical data indicate that standard therapy affects diverse immune cell subsets, with primary immunodeficiency related to long-lasting T cell lymphopenia. However, standard therapy differentially affects the immune system of each patient, and the rate and extent of lymphocyte count recovery after standard therapy differ significantly between patients. Most patients do not recover to baseline levels.
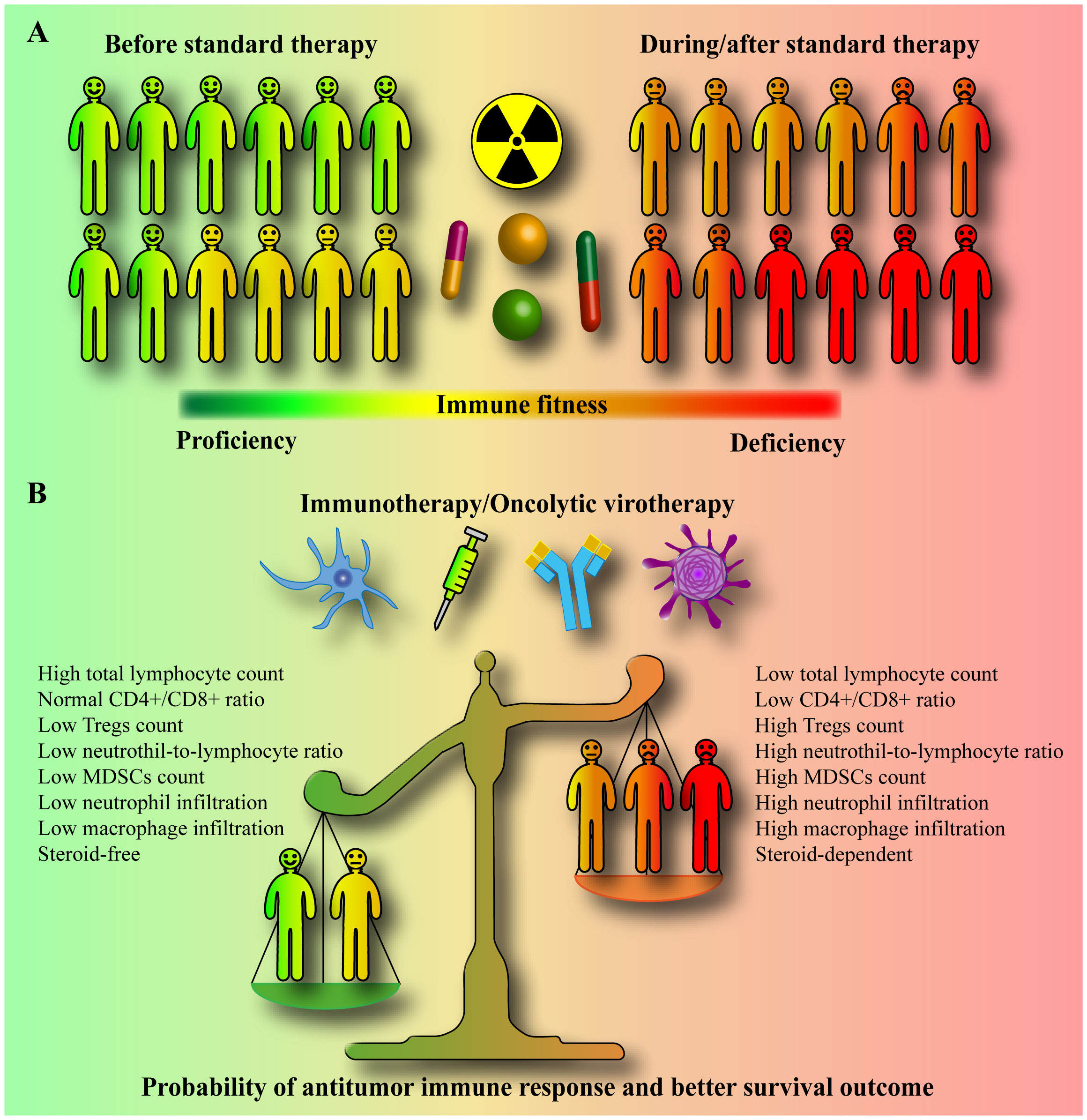
Figure 1 Standard therapy-promoted systemic immunosuppression may compromise the efficacy of immunotherapy/oncolytic virotherapy. (A) Prior to initiation of standard therapy, patients with glioblastoma/gliomas display varying degrees of immunosuppression. However, standard therapy, which includes radiation, a genotoxic drug temozolomide, and steroid dexamethasone, is a major cause of immune deficiency in patients, inducing long-lasting severe systemic immunosuppression and lymphopenia with a poor survival prognosis. The standard therapy differentially affects the immune system of each patient, with some patients developing moderate and some severe immunosuppression. Moreover, the rate and extent of lymphocyte count recovery after standard therapy also differ significantly between patients. (B) Immunotherapy and oncolytic virotherapy rely on the activity of the host’s own immune cells. The circulating and tumor-infiltrating CD4+, CD8+, NK, NKT, neutrophil, macrophage, myeloid-derived suppressor cell (MDSC), and regulatory T cell (Treg) counts, and subsets, and their relative ratios determine the immunological fitness of a patient. In clinical trials, immunotherapy and oncolytic virotherapy are largely tested in patients treated concurrently with or after standard therapy (in progressive/recurrent patients). Standard therapy-promoted immunosuppression/lymphopenia may limit the ability of the immune system to target glioblastoma. It is very likely that patients with lower systemic immune suppression may generally benefit from immunotherapy or oncolytic virotherapy much better than severely immune compromised patients who might be non-responsive to any extent. A high baseline neutrophil to lymphocyte ratio (NLR), a low post-treatment total lymphocyte count (TLC), and dexamethasone use are significant prognostic factors for shorter overall survival.
Standard therapy-promoted systemic immunosuppression and lymphopenia may limit the immune system’s ability to target glioblastoma (Figure 1B). Hence, changes in the standard therapy paradigm are required to increase the success of immunotherapies. Eligibility criteria, particularly relevant in neuro-oncology, and inadequate phase II study designs have been critically reviewed elsewhere (239). As for the design of clinical trials for glioblastoma to improve the effectiveness of immunotherapy/oncolytic virotherapy in general, the following aspects, supported by clinical data, must be taken into account (196, 197, 240, 241). First, the circulating blood within the blood vessels is recognized as an organ at risk for radiotherapy. Photon therapy, larger planning target volume, and higher brain dose were associated with increased risk of severe lymphopenia in glioblastoma, which correlates with poor survival. Therefore, the best efforts should be aimed to find and implement an efficient lymphocyte-sparing radiation modality (regimen and technique). Irradiation to the minimum necessary target using high-precision imaging (a minimal target definition), reduction of low-dose irradiation around the target (e.g., proton therapy), and a smaller number of fractions (i.e., hypo-fractionation) would be the strategy. Second, concurrent temozolomide and overall corticosteroid exposure during radiotherapy are contributing factors to lymphopenia. For trial enrollment, particular consideration should be given to patients without grade 2-4 lymphopenia, who are not expected to require steroids, and with an unmethylated MGMT promoter, for whom lymphotoxic temozolomide have to be completely omitted. Third, absolute/total lymphocyte counts should be monitored after radiotherapy, and appropriate effective interventions overcoming radiation-induced lymphopenia should be established and applied. Fourth, high NLR (31–33) and low post-treatment TLC are significant prognostic factors for shorter survival in patients with glioblastoma/gliomas. These and other cost-effective, widely and easily available clinically relevant prognostic immune variables associated with systemic inflammation and adaptive immunity (e.g., platelet-to-lymphocyte ratio (PLR), lymphocyte-to-monocyte ratio (LMR), systemic immune-inflammation index (SII), systemic immune response index (SIRI), or combinations thereof) (33, 242–248) should be reported and correlated with response and survival in immunotherapy/oncolytic virotherapy studies. In addition, since the use of different time points to define treatment-related lymphopenia has been reported to modify the prognostic power of lymphocyte counts, uniform time-points in standard therapy-related lymphopenia assessment should be established. Finally, detailed immunophenotyping of blood and/or tumor samples to assess the predictive/prognostic clinical significance of immune-related variables may also be valuable for future therapy decision-making in immunotherapy/oncolytic virotherapy trials.
Author contributions
AAS: Conceptualization, Funding acquisition, Investigation, Visualization, Writing – original draft, Writing – review & editing. AOS: Writing – review & editing. MPV: Writing – review & editing. AAC: Writing – review & editing. OVA: Writing – review & editing. VAN: Writing – review & editing. VPC: Writing – review & editing.
Funding
The author(s) declare financial support was received for the research, authorship, and/or publication of this article. The work was financially supported by the Russian Science Foundation (RSF), grant no. 22-75-10087. The funder had no role in data collection and analysis, decision to publish, or preparation of the manuscript.
Conflict of interest
The authors declare that the research was conducted in the absence of any commercial or financial relationships that could be construed as a potential conflict of interest.
Publisher’s note
All claims expressed in this article are solely those of the authors and do not necessarily represent those of their affiliated organizations, or those of the publisher, the editors and the reviewers. Any product that may be evaluated in this article, or claim that may be made by its manufacturer, is not guaranteed or endorsed by the publisher.
References
1. Miller KD, Ostrom QT, Kruchko C, Patil N, Tihan T, Cioffi G, et al. Brain and other central nervous system tumor statistics, 2021. CA Cancer J Clin (2021) 71:381–406. doi: 10.3322/caac.21693
2. Walker EV, Zhou Y, Wu Y, Liu J, Climans SA, Davis FG, et al. The incidence and prevalence of primary central nervous system (CNS) tumours in Canada (2010–2017), and the survival of patients diagnosed with CNS tumours (2008–2017). Curr Oncol (2023) 30:4311–28. doi: 10.3390/curroncol30040329
3. Olivier T, Migliorini D. Autologous tumor lysate-loaded dendritic cell vaccination in glioblastoma: What happened to the evidence? Rev Neurol (Paris) (2023) 179:502–5. doi: 10.1016/j.neurol.2023.03.014
4. Vatu BI, Artene S-A, Staicu A-G, Turcu-Stiolica A, Folcuti C, Dragoi A, et al. Assessment of efficacy of dendritic cell therapy and viral therapy in high grade glioma clinical trials. A meta-analytic review. J Immunoassay Immunochem (2019) 40:70–80. doi: 10.1080/15321819.2018.1551804
5. Najafi M, Jahanbakhshi A, Finocchi Ghersi S, Giaccherini L, Botti A, Cavallieri F, et al. Clinical effects of immuno-oncology therapy on glioblastoma patients: A systematic review. Brain Sci (2023) 13:159. doi: 10.3390/brainsci13020159
6. Stepanenko AA, Chekhonin VP. Recent advances in oncolytic virotherapy and immunotherapy for glioblastoma: A glimmer of hope in the search for an effective therapy? Cancers (Basel) (2018) 10:492. doi: 10.3390/cancers10120492
7. Lara-Velazquez M, Shireman JM, Lehrer EJ, Bowman KM, Ruiz-Garcia H, Paukner MJ, et al. A comparison between chemo-radiotherapy combined with immunotherapy and chemo-radiotherapy alone for the treatment of newly diagnosed glioblastoma: A systematic review and meta-analysis. Front Oncol (2021) 11:662302. doi: 10.3389/fonc.2021.662302
8. Agosti E, Zeppieri M, De Maria L, Tedeschi C, Fontanella MM, Panciani PP, et al. Glioblastoma immunotherapy: A systematic review of the present strategies and prospects for advancements. Int J Mol Sci (2023) 24:15037. doi: 10.3390/ijms242015037
9. Gorlia T, van den Bent MJ, Hegi ME, Mirimanoff RO, Weller M, Cairncross JG, et al. Nomograms for predicting survival of patients with newly diagnosed glioblastoma: prognostic factor analysis of EORTC and NCIC trial 26981-22981/CE.3. Lancet Oncol (2008) 9:29–38. doi: 10.1016/S1470-2045(07)70384-4
10. Gittleman H, Lim D, Kattan MW, Chakravarti A, Gilbert MR, Lassman AB, et al. An independently validated nomogram for individualized estimation of survival among patients with newly diagnosed glioblastoma: NRG Oncology RTOG 0525 and 0825. Neuro Oncol (2017) 19:669–77. doi: 10.1093/neuonc/now208
11. Horbinski C, Berger T, Packer RJ, Wen PY. Clinical implications of the 2021 edition of the WHO classification of central nervous system tumours. Nat Rev Neurol (2022) 18:515–29. doi: 10.1038/s41582-022-00679-w
12. Sharma P, Aaroe A, Liang J, Puduvalli VK. Tumor microenvironment in glioblastoma: Current and emerging concepts. Neuro-Oncol Adv (2023) 5. doi: 10.1093/noajnl/vdad009
13. Arrieta VA, Dmello C, McGrail DJ, Brat DJ, Lee-Chang C, Heimberger AB, et al. Immune checkpoint blockade in glioblastoma: from tumor heterogeneity to personalized treatment. J Clin Invest (2023) 133. doi: 10.1172/JCI163447
14. Gillette JS, Wang EJ, Dowd RS, Toms SA. Barriers to overcoming immunotherapy resistance in glioblastoma. Front Med (2023) 10:1175507. doi: 10.3389/fmed.2023.1175507
15. Ott M, Tomaszowski K-H, Marisetty A, Kong L-Y, Wei J, Duna M, et al. Profiling of patients with glioma reveals the dominant immunosuppressive axis is refractory to immune function restoration. JCI Insight (2020) 5. doi: 10.1172/jci.insight.134386
16. Butt NS, Kurdi M, Fadul MM, Hakamy S, Addas BMJ, Faizo E, et al. Major Histocompatibility Class-I (MHC-I) downregulation in glioblastoma is a poor prognostic factor but not a predictive indicator for treatment failure. Pathol - Res Pract (2023) 250:154816. doi: 10.1016/j.prp.2023.154816
17. Liu J, Piranlioglu R, Ye F, Shu K, Lei T, Nakashima H. Immunosuppressive cells in oncolytic virotherapy for glioma: challenges and solutions. Front Cell Infect Microbiol (2023) 13:1141034. doi: 10.3389/fcimb.2023.1141034
18. Lin Y-J, Wu CY-J, Wu JY, Lim M. The role of myeloid cells in GBM immunosuppression. Front Immunol (2022) 13:887781. doi: 10.3389/fimmu.2022.887781
19. Guo X, Wang G. Advances in research on immune escape mechanism of glioma. CNS Neurosci Ther (2023) 29:1709–20. doi: 10.1111/cns.14217
20. Jain P, Vashist S, Panjiyar BK. Navigating the immune challenge in glioblastoma: exploring immunotherapeutic avenues for overcoming immune suppression. Cureus (2023) 15(9):e46089. doi: 10.7759/cureus.46089
21. Sarkaria JN, Hu LS, Parney IF, Pafundi DH, Brinkmann DH, Laack NN, et al. Is the blood–brain barrier really disrupted in all glioblastomas? A critical assessment of existing clinical data. Neuro Oncol (2018) 20:184–91. doi: 10.1093/neuonc/nox175
22. Hu X, Deng Q, Ma L, Li Q, Chen Y, Liao Y, et al. Meningeal lymphatic vessels regulate brain tumor drainage and immunity. Cell Res (2020) 30:229–43. doi: 10.1038/s41422-020-0287-8
23. Thorsson V, Gibbs DL, Brown SD, Wolf D, Bortone DS, Ou Yang T-H, et al. The immune landscape of cancer. Immunity (2018) 48:812–830.e14. doi: 10.1016/j.immuni.2018.03.023
24. Watowich MB, Gilbert MR, Larion M. T cell exhaustion in Malignant gliomas. Trends Cancer (2023) 9:270–92. doi: 10.1016/j.trecan.2022.12.008
25. Choudhary N, Osorio RC, Oh JY, Aghi MK. Metabolic barriers to glioblastoma immunotherapy. Cancers (Basel) (2023) 15:1519. doi: 10.3390/cancers15051519
26. Richardson LG, Miller JJ, Kitagawa Y, Wakimoto H, Choi BD, Curry WT. Implications of IDH mutations on immunotherapeutic strategies for Malignant glioma. Neurosurg Focus (2022) 52:E6. doi: 10.3171/2021.11.FOCUS21604
27. Yan D, Li W, Liu Q, Yang K. Advances in immune microenvironment and immunotherapy of isocitrate dehydrogenase mutated glioma. Front Immunol (2022) 13:914618. doi: 10.3389/fimmu.2022.914618
28. Chongsathidkiet P, Jackson C, Koyama S, Loebel F, Cui X, Farber SH, et al. Sequestration of T cells in bone marrow in the setting of glioblastoma and other intracranial tumors. Nat Med (2018) 24:1459–68. doi: 10.1038/s41591-018-0135-2
29. Deng D, Hammoudeh L, Youssef G, Chen Y-H, Shin K-Y, Lim-Fat MJ, et al. Evaluating hematologic parameters in newly diagnosed and recurrent glioblastoma: Prognostic utility and clinical trial implications of myelosuppression. Neuro-oncol Adv (2023) 5:vdad083. doi: 10.1093/noajnl/vdad083
30. Gardam B, Gargett T, Brown MP, Ebert LM. Targeting the dendritic cell-T cell axis to develop effective immunotherapies for glioblastoma. Front Immunol (2023) 14:1261257. doi: 10.3389/fimmu.2023.1261257
31. Lei Y, Li Y, Hu Q, Wang J, Sui A. Prognostic impact of neutrophil-to-lymphocyte ratio in gliomas: a systematic review and meta-analysis. World J Surg Oncol (2019) 17:152. doi: 10.1186/s12957-019-1686-5
32. Guo X, Jiao H, Zhang T, Zhang Y. Pre-treatment and preoperative neutrophil-to-lymphocyte ratio predicts prognostic value of glioblastoma: A meta-analysis. Brain Sci (2022) 12:675. doi: 10.3390/brainsci12050675
33. Jarmuzek P, Kozlowska K, Defort P, Kot M, Zembron-Lacny A. Prognostic values of systemic inflammatory immunological markers in glioblastoma: A systematic review and meta-analysis. Cancers (Basel) (2023) 15:3339. doi: 10.3390/cancers15133339
34. Fecci PE, Mitchell DA, Whitesides JF, Xie W, Friedman AH, Archer GE, et al. Increased regulatory T-cell fraction amidst a diminished CD4 compartment explains cellular immune defects in patients with Malignant glioma. Cancer Res (2006) 66:3294–302. doi: 10.1158/0008-5472.CAN-05-3773
35. Gabrusiewicz K, Rodriguez B, Wei J, Hashimoto Y, Healy LM, Maiti SN, et al. Glioblastoma-infiltrated innate immune cells resemble M0 macrophage phenotype. JCI Insight (2016) 1. doi: 10.1172/jci.insight.85841
36. Gielen PR, Schulte BM, Kers-Rebel ED, Verrijp K, Petersen-Baltussen HMJM, ter Laan M, et al. Increase in both CD14-positive and CD15-positive myeloid-derived suppressor cell subpopulations in the blood of patients with glioma but predominance of CD15-positive myeloid-derived suppressor cells in glioma tissue. J Neuropathol Exp Neurol (2015) 74:390–400. doi: 10.1097/NEN.0000000000000183
37. Chiba Y, Hashimoto N, Tsuboi A, Oka Y, Murao A, Kinoshita M, et al. Effects of concomitant temozolomide and radiation therapies on WT1-specific T-cells in Malignant glioma. Jpn J Clin Oncol (2010) 40:395–403. doi: 10.1093/jjco/hyp196
38. Fadul CE, Fisher JL, Gui J, Hampton TH, Cote AL, Ernstoff MS. Immune modulation effects of concomitant temozolomide and radiation therapy on peripheral blood mononuclear cells in patients with glioblastoma multiforme. Neuro Oncol (2011) 13:393–400. doi: 10.1093/neuonc/noq204
39. Ellsworth S, Balmanoukian A, Kos F, Nirschl CJ, Nirschl TR, Grossman SA, et al. Sustained CD4 + T cell-driven lymphopenia without a compensatory IL-7/IL-15 response among high-grade glioma patients treated with radiation and temozolomide. Oncoimmunology (2014) 3:e27357. doi: 10.4161/onci.27357
40. Campian JL, Ye X, Gladstone DE, Ambady P, Nirschl TR, Borrello I, et al. Pre-radiation lymphocyte harvesting and post-radiation reinfusion in patients with newly diagnosed high grade gliomas. J Neurooncol (2015) 124:307–16. doi: 10.1007/s11060-015-1841-y
41. Campian JL, Piotrowski AF, Ye X, Hakim FT, Rose J, Yan X-Y, et al. Serial changes in lymphocyte subsets in patients with newly diagnosed high grade astrocytomas treated with standard radiation and temozolomide. J Neurooncol (2017) 135:343–51. doi: 10.1007/s11060-017-2580-z
42. Pellegatta S, Eoli M, Cuccarini V, Anghileri E, Pollo B, Pessina S, et al. Survival gain in glioblastoma patients treated with dendritic cell immunotherapy is associated with increased NK but not CD8+ T cell activation in the presence of adjuvant temozolomide. Oncoimmunology (2018) 7:e1412901. doi: 10.1080/2162402X.2017.1412901
43. Nayak L, Standifer N, Dietrich J, Clarke JL, Dunn GP, Lim M, et al. Circulating immune cell and outcome analysis from the phase II study of PD-L1 blockade with durvalumab for newly diagnosed and recurrent glioblastoma. Clin Cancer Res (2022) 28:2567–78. doi: 10.1158/1078-0432.CCR-21-4064
44. Batich KA, Reap EA, Archer GE, Sanchez-Perez L, Nair SK, Schmittling RJ, et al. Long-term survival in glioblastoma with cytomegalovirus pp65-targeted vaccination. Clin Cancer Res (2017) 23:1898–909. doi: 10.1158/1078-0432.CCR-16-2057
45. Sampson JH, Aldape KD, Archer GE, Coan A, Desjardins A, Friedman AH, et al. Greater chemotherapy-induced lymphopenia enhances tumor-specific immune responses that eliminate EGFRvIII-expressing tumor cells in patients with glioblastoma. Neuro Oncol (2011) 13:324–33. doi: 10.1093/neuonc/noq157
46. Garcia CR, Myint ZW, Jayswal R, Wang C, Morgan RM, Butts AR, et al. Hematological adverse events in the management of glioblastoma. J Neurooncol (2022) 156:153–61. doi: 10.1007/s11060-021-03891-8
47. Gupta T, Mohanty S, Moiyadi A, Jalali R. Factors predicting temozolomide induced clinically significant acute hematologic toxicity in patients with high-grade gliomas: A clinical audit. Clin Neurol Neurosurg (2013) 115:1814–9. doi: 10.1016/j.clineuro.2013.05.015
48. Ishikawa E, Yamamoto T, Sakamoto N, Nakai K, Akutsu H, Tsuboi K, et al. Low peripheral lymphocyte count before focal radiotherapy plus concomitant temozolomide predicts severe lymphopenia during Malignant glioma treatment. Neurol Med Chir (Tokyo) (2010) 50:638–44. doi: 10.2176/nmc.50.638
49. Gerber DE, Grossman SA, Zeltzman M, Parisi MA, Kleinberg L. The impact of thrombocytopenia from temozolomide and radiation in newly diagnosed adults with high-grade gliomas1. Neuro Oncol (2007) 9:47–52. doi: 10.1215/15228517-2006-024
50. Mendez JS, Govindan A, Leong J, Gao F, Huang J, Campian JL. Association between treatment-related lymphopenia and overall survival in elderly patients with newly diagnosed glioblastoma. J Neurooncol (2016) 127:329–35. doi: 10.1007/s11060-015-2037-1
51. Yovino S, Grossman SA. Severity, etiology and possible consequences of treatment-related lymphopenia in patients with newly diagnosed high-grade gliomas. CNS Oncol (2012) 1:149–54. doi: 10.2217/cns.12.14
52. Grossman SA, Ellsworth S, Campian J, Wild AT, Herman JM, Laheru D, et al. Survival in patients with severe lymphopenia following treatment with radiation and chemotherapy for newly diagnosed solid tumors. J Natl Compr Canc Netw (2015) 13:1225–31. doi: 10.6004/jnccn.2015.0151
53. Kim WJ, Dho Y-S, Ock C-Y, Kim JW, Choi SH, Lee S-T, et al. Clinical observation of lymphopenia in patients with newly diagnosed glioblastoma. J Neurooncol (2019) 143:321–8. doi: 10.1007/s11060-019-03167-2
54. Ahn S, Park J-S, Jang J, Ahn K-J, Hong Y-K, Yang SH, et al. The association between total lymphocyte count after concomitant chemoradiation and overall survival in patients with newly diagnosed glioblastoma. J Clin Neurosci (2020) 71:21–5. doi: 10.1016/j.jocn.2019.11.017
55. Hui CY, Rudra S, Ma S, Campian JL, Huang J. Impact of overall corticosteroid exposure during chemoradiotherapy on lymphopenia and survival of glioblastoma patients. J Neurooncol (2019) 143(1):129–36. doi: 10.1007/s11060-019-03146-7
56. Huang J, DeWees TA, Badiyan SN, Speirs CK, Mullen DF, Fergus S, et al. Clinical and dosimetric predictors of acute severe lymphopenia during radiation therapy and concurrent temozolomide for high-grade glioma. Int J Radiat Oncol (2015) 92:1000–7. doi: 10.1016/j.ijrobp.2015.04.005
57. Byun HK, Kim N, Yoon HI, Kang S-G, Kim SH, Cho J, et al. Clinical predictors of radiation-induced lymphopenia in patients receiving chemoradiation for glioblastoma: clinical usefulness of intensity-modulated radiotherapy in the immuno-oncology era. Radiat Oncol (2019) 14:51. doi: 10.1186/s13014-019-1256-6
58. Le Rhun E, Oppong FB, Vanlancker M, Stupp R, Nabors B, Chinot O, et al. Prognostic significance of therapy-induced myelosuppression in newly diagnosed glioblastoma. Neuro Oncol (2022) 24:1533–45. doi: 10.1093/neuonc/noac070
59. Perry JR, Laperriere N, O’Callaghan CJ, Brandes AA, Menten J, Phillips C, et al. Short-course radiation plus temozolomide in elderly patients with glioblastoma. N Engl J Med (2017) 376:1027–37. doi: 10.1056/NEJMoa1611977
60. Rahman R, Catalano PJ, Arvold ND, Aizer AA, Weiss SE, Pinnell N, et al. Chemoradiation-related lymphopenia is common among glioblastoma patients and is associated with worse progression-free and overall survival. Int J Radiat Oncol (2016) 96:E123. doi: 10.1016/j.ijrobp.2016.06.2512
61. Song AJ, Ding K, Alnahhas I, Laperriere NJ, Perry J, Mason WP, et al. Impact of lymphopenia on survival for elderly patients with glioblastoma: A secondary analysis of the CCTG CE.6 (EORTC 26062-22061, TROG03.01) randomized clinical trial. Neuro-oncol Adv (2021) 3:vdab153. doi: 10.1093/noajnl/vdab153
62. Lin AJ, Campian JL, Hui C, Rudra S, Rao YJ, Thotala D, et al. Impact of concurrent versus adjuvant chemotherapy on the severity and duration of lymphopenia in glioma patients treated with radiation therapy. J Neurooncol (2018) 136:403–11. doi: 10.1007/s11060-017-2668-5
63. Mohme M, Schliffke S, Maire CL, Rünger A, Glau L, Mende KC, et al. Immunophenotyping of newly diagnosed and recurrent glioblastoma defines distinct immune exhaustion profiles in peripheral and tumor-infiltrating lymphocytes. Clin Cancer Res (2018) 24(17):4187–200. doi: 10.1158/1078-0432.CCR-17-2617
64. Wei W, Chen X, Ma X, Wang D, Guo Z. The efficacy and safety of various dose-dense regimens of temozolomide for recurrent high-grade glioma: a systematic review with meta-analysis. J Neurooncol (2015) 125:339–49. doi: 10.1007/s11060-015-1920-0
65. Miljković MD, Grossman SA, Ye X, Ellsworth S, Terezakis S. Patterns of radiation-associated lymphopenia in children with cancer. Cancer Invest (2016) 34:32–8. doi: 10.3109/07357907.2015.1086366
66. Hughes MA, Parisi M, Grossman S, Kleinberg L. Primary brain tumors treated with steroids and radiotherapy: Low CD4 counts and risk of infection. Int J Radiat Oncol (2005) 62:1423–6. doi: 10.1016/j.ijrobp.2004.12.085
67. Grossman SA, Ye X, Lesser G, Sloan A, Carraway H, Desideri S, et al. Immunosuppression in patients with high-grade gliomas treated with radiation and temozolomide. Clin Cancer Res (2011) 17:5473–80. doi: 10.1158/1078-0432.CCR-11-0774
68. Murphy K, Griffith T. CD8 T cell–independent antitumor response and its potential for treatment of Malignant gliomas. Cancers (Basel) (2016) 8:71. doi: 10.3390/cancers8080071
69. Zhang Y, Chen S, Chen H, Chen S, Li Z, Feng E, et al. Prognostic value and risk factors of treatment-related lymphopenia in Malignant glioma patients treated with chemoradiotherapy: A systematic review and meta-analysis. Front Neurol (2021) 12:726561. doi: 10.3389/fneur.2021.726561
70. Neff RD, Cassen B. Relative radiation sensitivity of circulating small and large lymphocytes. J Nucl Med (1968) 9:402–5.
71. Heylmann D, Rödel F, Kindler T, Kaina B. Radiation sensitivity of human and murine peripheral blood lymphocytes, stem and progenitor cells. Biochim Biophys Acta - Rev Cancer (2014) 1846:121–9. doi: 10.1016/j.bbcan.2014.04.009
72. Paganetti H. A review on lymphocyte radiosensitivity and its impact on radiotherapy. Front Oncol (2023) 13:1201500. doi: 10.3389/fonc.2023.1201500
73. Falcke S, Rühle P, Deloch L, Fietkau R, Frey B, Gaipl U. Clinically relevant radiation exposure differentially impacts forms of cell death in human cells of the innate and adaptive immune system. Int J Mol Sci (2018) 19:3574. doi: 10.3390/ijms19113574
74. Cronkite EP, Jansen CR, Mather GC, Nielsen NO, Usenik EA, Adamik ER, et al. Studies on lymphocytes. I. Lymphopenia produced by prolonged extracorporeal irradiation of circulating blood. Blood (1962) 20:203–13. doi: 10.1182/blood.V20.2.203.203
75. Weeke E. The development of lymphopenia in uremic patients undergoing extracorporeal irradiation of the blood with portable beta units. Radiat Res (1973) 56:554–9. doi: 10.2307/3573724
76. Weeke E. Extracorporeal irradiation of the blood. Further investigations on the effect of varying transit dose, blood flow rate and frequency of treatment of the development of lymphopenia in uremic patients. Acta Med Scand (1974) 195:149–54. doi: 10.1111/j.0954-6820.1974.tb08116.x
77. Yovino S, Kleinberg L, Grossman SA, Narayanan M, Ford E. The etiology of treatment-related lymphopenia in patients with Malignant gliomas: modeling radiation dose to circulating lymphocytes explains clinical observations and suggests methods of modifying the impact of radiation on immune cells. Cancer Invest (2013) 31:140–4. doi: 10.3109/07357907.2012.762780
78. Ellsworth SG. Field size effects on the risk and severity of treatment-induced lymphopenia in patients undergoing radiation therapy for solid tumors. Adv Radiat Oncol (2018) 3:512–9. doi: 10.1016/j.adro.2018.08.014
79. El Houat Y, Massard C, Quillien V, de Crevoisier R, Castelli J. Meta-analysis and critical review: association between radio-induced lymphopenia and overall survival in solid cancers. Adv Radiat Oncol (2023) 8:101038. doi: 10.1016/j.adro.2022.101038
80. Venkatesulu BP, Chan DP, Giridhar P, Upadhyay R, Sharma A, Elghazawy H, et al. A systematic review and meta-analysis of the impact of radiation-related lymphopenia on outcomes in pancreatic cancer. Futur Oncol (2022) 18:1885–95. doi: 10.2217/fon-2021-0483
81. Upadhyay R, Venkatesulu BP, Giridhar P, Kim BK, Sharma A, Elghazawy H, et al. Risk and impact of radiation related lymphopenia in lung cancer: A systematic review and meta-analysis. Radiother Oncol (2021) 157:225–33. doi: 10.1016/j.radonc.2021.01.034
82. Dai D, Tian Q, Shui Y, Li J, Wei Q. The impact of radiation induced lymphopenia in the prognosis of head and neck cancer: A systematic review and meta-analysis. Radiother Oncol (2022) 168:28–36. doi: 10.1016/j.radonc.2022.01.003
83. Wang X, Wang P, Zhao Z, Mao Q, Yu J, Li M. A review of radiation-induced lymphopenia in patients with esophageal cancer: an immunological perspective for radiotherapy. Ther Adv Med Oncol (2020) 12:175883592092682. doi: 10.1177/1758835920926822
84. Dai D, Tian Q, Yu G, Shui Y, Jiang H, Wei Q. Severe radiation-induced lymphopenia affects the outcomes of esophageal cancer: A comprehensive systematic review and meta-analysis. Cancers (Basel) (2022) 14:3024. doi: 10.3390/cancers14123024
85. Danckaert W, Spaas M, Vandecasteele K, De Wagter C, Ost P. Impact of radiotherapy parameters on the risk of lymphopenia in urological tumors: A systematic review of the literature. Radiother Oncol (2022) 170:64–9. doi: 10.1016/j.radonc.2022.02.030
86. Kleinberg L, Sloan L, Grossman S, Lim M. Radiotherapy, lymphopenia, and host immune capacity in glioblastoma: A potentially actionable toxicity associated with reduced efficacy of radiotherapy. Neurosurgery (2019) 85:441–53. doi: 10.1093/neuros/nyz198
87. Venkatesulu BP, Mallick S, Lin SH, Krishnan S. A systematic review of the influence of radiation-induced lymphopenia on survival outcomes in solid tumors. Crit Rev Oncol Hematol (2018) 123:42–51. doi: 10.1016/j.critrevonc.2018.01.003
88. Ménétrier-Caux C, Ray-Coquard I, Blay J-Y, Caux C. Lymphopenia in Cancer Patients and its Effects on Response to Immunotherapy: an opportunity for combination with Cytokines? J Immunother Cancer (2019) 7:85. doi: 10.1186/s40425-019-0549-5
89. Hsu EJ, Thomas J, Maher EA, Youssef M, Timmerman RD, Wardak Z, et al. Neutrophilia and post-radiation thrombocytopenia predict for poor prognosis in radiation-treated glioma patients. Front Oncol (2022) 12:1000280. doi: 10.3389/fonc.2022.1000280
90. Schwarzberg AB, Stover EH, Sengupta T, Michelini A, Vincitore M, Baden LR, et al. Selective lymphopenia and opportunistic infections in neuroendocrine tumor patients receiving temozolomide. Cancer Invest (2007) 25:249–55. doi: 10.1080/07357900701206380
91. Rietschel P, Wolchok JD, Krown S, Gerst S, Jungbluth AA, Busam K, et al. Phase II study of extended-dose temozolomide in patients with melanoma. J Clin Oncol (2008) 26:2299–304. doi: 10.1200/JCO.2007.14.5292
92. Iversen TZ, Brimnes MK, Nikolajsen K, Andersen RS, Hadrup SR, Andersen MH, et al. Depletion of T lymphocytes is correlated with response to temozolomide in melanoma patients. Oncoimmunology (2013) 2:e23288. doi: 10.4161/onci.23288
93. Su YB, Sohn S, Krown SE, Livingston PO, Wolchok JD, Quinn C, et al. Selective CD4+ lymphopenia in melanoma patients treated with temozolomide: a toxicity with therapeutic implications. J Clin Oncol (2004) 22:610–6. doi: 10.1200/JCO.2004.07.060
94. Verma R, Foster RE, Horgan K, Mounsey K, Nixon H, Smalle N, et al. Lymphocyte depletion and repopulation after chemotherapy for primary breast cancer. Breast Cancer Res (2016) 18:10. doi: 10.1186/s13058-015-0669-x
95. Kostaras X, Cusano F, Kline GA, Roa W, Easaw J. Use of dexamethasone in patients with high-grade glioma: a clinical practice guideline. Curr Oncol (2014) 21:e493–503. doi: 10.3747/co.21.1769
96. Roth P, Happold C, Weller M. Corticosteroid use in neuro-oncology: an update. Neuro-oncol Pract (2015) 2:6–12. doi: 10.1093/nop/npu029
97. Coutinho AE, Chapman KE. The anti-inflammatory and immunosuppressive effects of glucocorticoids, recent developments and mechanistic insights. Mol Cell Endocrinol (2011) 335:2–13. doi: 10.1016/j.mce.2010.04.005
98. Zen M, Canova M, Campana C, Bettio S, Nalotto L, Rampudda M, et al. The kaleidoscope of glucorticoid effects on immune system. Autoimmun Rev (2011) 10:305–10. doi: 10.1016/j.autrev.2010.11.009
99. Cain DW, Cidlowski JA. Immune regulation by glucocorticoids. Nat Rev Immunol (2017) 17:233–47. doi: 10.1038/nri.2017.1
100. Sengupta S, Thaci B, Crawford AC, Sampath P. Interleukin-13 receptor alpha 2-targeted glioblastoma immunotherapy. BioMed Res Int (2014) 2014:1–8. doi: 10.1155/2014/952128
101. Stepanenko A, Andreieva S, Korets K, Mykytenko D, Huleyuk N, Vassetzky Y, et al. Step-wise and punctuated genome evolution drive phenotype changes of tumor cells. Mutat Res (2015) 771:56–69. doi: 10.1016/j.mrfmmm.2014.12.006
102. Gustafson MP, Lin Y, New KC, Bulur PA, O’Neill BP, Gastineau DA, et al. Systemic immune suppression in glioblastoma: the interplay between CD14+HLA-DRlo/neg monocytes, tumor factors, and dexamethasone. Neuro Oncol (2010) 12:631–44. doi: 10.1093/neuonc/noq001
103. Chitadze G, Flüh C, Quabius ES, Freitag-Wolf S, Peters C, Lettau M, et al. In-depth immunophenotyping of patients with glioblastoma multiforme: Impact of steroid treatment. Oncoimmunology (2017) 6:e1358839. doi: 10.1080/2162402X.2017.1358839
104. Bracci PM, Rice T, Hansen HM, Francis SS, Lee S, McCoy LS, et al. Pre-surgery immune profiles of adult glioma patients. J Neurooncol (2022) 159:103–15. doi: 10.1007/s11060-022-04047-y
105. Cook AM, McDonnell AM, Lake RA, Nowak AK. Dexamethasone co-medication in cancer patients undergoing chemotherapy causes substantial immunomodulatory effects with implications for chemo-immunotherapy strategies. Oncoimmunology (2016) 5:e1066062. doi: 10.1080/2162402X.2015.1066062
106. Nakamura T, Ushigome H. Myeloid-derived suppressor cells as a regulator of immunity in organ transplantation. Int J Mol Sci (2018) 19:2357. doi: 10.3390/ijms19082357
107. Vaios EJ, Nahed BV, Muzikansky A, Fathi AT, Dietrich J. Bone marrow response as a potential biomarker of outcomes in glioblastoma patients. J Neurosurg (2017) 127:132–8. doi: 10.3171/2016.7.JNS16609
108. Fossati G, Ricevuti G, Edwards SW, Walker C, Dalton A, Rossi ML. Neutrophil infiltration into human gliomas. Acta Neuropathol (1999) 98:349–54. doi: 10.1007/s004010051093
109. Mason M, Maurice C, McNamara MG, Tieu MT, Lwin Z, Millar B-A, et al. Neutrophil–lymphocyte ratio dynamics during concurrent chemo-radiotherapy for glioblastoma is an independent predictor for overall survival. J Neurooncol (2017) 132:463–71. doi: 10.1007/s11060-017-2395-y
110. Carenza C, Franzese S, Castagna A, Terzoli S, Simonelli M, Persico P, et al. Perioperative corticosteroid treatment impairs tumor-infiltrating dendritic cells in patients with newly diagnosed adult-type diffuse gliomas. Front Immunol (2022) 13:1074762. doi: 10.3389/fimmu.2022.1074762
111. Hohwieler Schloss M, Freidberg SR, Heatley GJ, Lo TC. Glucocorticoid dependency as a prognostic factor in radiotherapy for cerebral gliomas. Acta Oncol (1989) 28:51–5. doi: 10.3109/02841868909111181
112. Watne K, Hannisdal E, Nome O, Hager B, Hirschberg H. Prognostic factors in Malignant gliomas with special reference to intra-arterial chemotherapy. Acta Oncol (1993) 32:307–10. doi: 10.3109/02841869309093600
113. Gorlia T, Stupp R, Brandes AA, Rampling RR, Fumoleau P, Dittrich C, et al. New prognostic factors and calculators for outcome prediction in patients with recurrent glioblastoma: a pooled analysis of EORTC Brain Tumour Group phase I and II clinical trials. Eur J Cancer (2012) 48:1176–84. doi: 10.1016/j.ejca.2012.02.004
114. Michaelsen SR, Christensen IJ, Grunnet K, Stockhausen M-T, Broholm H, Kosteljanetz M, et al. Clinical variables serve as prognostic factors in a model for survival from glioblastoma multiforme: an observational study of a cohort of consecutive non-selected patients from a single institution. BMC Cancer (2013) 13:402. doi: 10.1186/1471-2407-13-402
115. Tieu MT, Lovblom LE, McNamara MG, Mason W, Laperriere N, Millar B-A, et al. Impact of glycemia on survival of glioblastoma patients treated with radiation and temozolomide. J Neurooncol (2015) 124:119–26. doi: 10.1007/s11060-015-1815-0
116. Pitter KL, Tamagno I, Alikhanyan K, Hosni-Ahmed A, Pattwell SS, Donnola S, et al. Corticosteroids compromise survival in glioblastoma. Brain (2016) 139:1458–71. doi: 10.1093/brain/aww046
117. van Linde ME, Brahm CG, de Witt Hamer PC, Reijneveld JC, Bruynzeel AME, Vandertop WP, et al. Treatment outcome of patients with recurrent glioblastoma multiforme: a retrospective multicenter analysis. J Neurooncol (2017) 135(1):183–92. doi: 10.1007/s11060-017-2564-z
118. Coleman N, Michalarea V, Alken S, Rihawi K, Lopez RP, Tunariu N, et al. Safety, efficacy and survival of patients with primary Malignant brain tumours (PMBT) in phase I (Ph1) trials: the 12-year Royal Marsden experience. J Neurooncol (2018) 139:107–16. doi: 10.1007/s11060-018-2847-z
119. Wong ET, Lok E, Gautam S, Swanson KD. Dexamethasone exerts profound immunologic interference on treatment efficacy for recurrent glioblastoma. Br J Cancer (2015) 113:232–41. doi: 10.1038/bjc.2015.238
120. Petrelli F, De Stefani A, Ghidini A, Bruschieri L, Riboldi V, Dottorini L, et al. Steroids use and survival in patients with glioblastoma multiforme: a pooled analysis. J Neurol (2021) 268:440–7. doi: 10.1007/s00415-020-09731-5
121. Schernberg A, Nivet A, Dhermain F, Ammari S, Escande A, Pallud J, et al. Neutrophilia as a biomarker for overall survival in newly diagnosed high-grade glioma patients undergoing chemoradiation. Clin Transl Radiat Oncol (2018) 10:47–52. doi: 10.1016/j.ctro.2018.04.002
122. Lee C, Ahn S, Park J-S, Song JH, Hong Y-K, Jeun S-S. Effect of cumulative dexamethasone dose during concomitant chemoradiation on lymphopenia in patients with newly diagnosed glioblastoma. Brain Tumor Res Treat (2020) 8:71. doi: 10.14791/btrt.2020.8.e12
123. Dubinski D, Won S-Y, Gessler F, Quick-Weller J, Behmanesh B, Bernatz S, et al. Dexamethasone-induced leukocytosis is associated with poor survival in newly diagnosed glioblastoma. J Neurooncol (2018) 137(3):503–10. doi: 10.1007/s11060-018-2761-4
124. Kmiecik J, Poli A, Brons NHC, Waha A, Eide GE, Enger PØ, et al. Elevated CD3+ and CD8+ tumor-infiltrating immune cells correlate with prolonged survival in glioblastoma patients despite integrated immunosuppressive mechanisms in the tumor microenvironment and at the systemic level. J Neuroimmunol (2013) 264:71–83. doi: 10.1016/j.jneuroim.2013.08.013
125. Iorgulescu JB, Gokhale PC, Speranza MC, Eschle BK, Poitras MJ, Wilkens MK, et al. Concurrent dexamethasone limits the clinical benefit of immune checkpoint blockade in glioblastoma. Clin Cancer Res (2021) 27:276–87. doi: 10.1158/1078-0432.ccr-20-2291
126. Swildens KX, Sillevis Smitt PAE, van den Bent MJ, French PJ, Geurts M. The effect of dexamethasone on the microenvironment and efficacy of checkpoint inhibitors in glioblastoma: a systematic review. Neuro-oncol Adv (2022) 4:vdac087. doi: 10.1093/noajnl/vdac087
127. Jessurun CAC, Hulsbergen AFC, de Wit AE, Tewarie IA, Snijders TJ, Verhoeff JJC, et al. The combined use of steroids and immune checkpoint inhibitors in brain metastasis patients: a systematic review and meta-analysis. Neuro Oncol (2021) 23:1261–72. doi: 10.1093/neuonc/noab046
128. Petrelli F, Signorelli D, Ghidini M, Ghidini A, Pizzutilo EG, Ruggieri L, et al. Association of steroids use with survival in patients treated with immune checkpoint inhibitors: A systematic review and meta-analysis. Cancers (Basel) (2020) 12:546. doi: 10.3390/cancers12030546
129. Van Buren I, Madison C, Kohn A, Berry E, Kulkarni RP, Thompson RF. Survival among veterans receiving steroids for immune-related adverse events after immune checkpoint inhibitor therapy. JAMA Netw Open (2023) 6:e2340695. doi: 10.1001/jamanetworkopen.2023.40695
130. Luedi MM, Singh SK, Mosley JC, Hassan ISA, Hatami M, Gumin J, et al. Dexamethasone-mediated oncogenicity in vitro and in an animal model of glioblastoma. J Neurosurg (2018) 129:1446–55. doi: 10.3171/2017.7.JNS17668
131. Gorodezki D, Zipfel J, Queudeville M, Holzer U, Bevot A, Schittenhelm J, et al. Evaluating the safety of perioperative dexamethasone treatment: A retrospective analysis of a single center pediatric low-grade glioma cohort. Int J Cancer (2023) 152:1875–83. doi: 10.1002/ijc.34399
132. Upadhyayula PS, Higgins DM, Argenziano MG, Spinazzi EF, Wu C-C, Canoll P, et al. The sledgehammer in precision medicine: dexamethasone and immunotherapeutic treatment of glioma. Cancer Invest (2022) 40:554–66. doi: 10.1080/07357907.2021.1944178
133. Hagan K, Bhavsar S, Arunkumar R, Grasu R, Dang A, Carlson R, et al. Association between perioperative hyperglycemia and survival in patients with glioblastoma. J Neurosurg Anesthesiol (2017) 29:21–9. doi: 10.1097/ANA.0000000000000339
134. Adeberg S, Bernhardt D, Foerster R, Bostel T, Koerber SA, Mohr A, et al. The influence of hyperglycemia during radiotherapy on survival in patients with primary glioblastoma. Acta Oncol (Madr) (2016) 55:201–7. doi: 10.3109/0284186X.2015.1043397
135. McGirt MJ, Chaichana KL, Gathinji M, Attenello F, Than K, Ruiz AJ, et al. Persistent outpatient hyperglycemia is independently associated with decreased survival after primary resection of malignant brain astrocytomas. Neurosurgery (2008) 63:286–91. doi: 10.1227/01.NEU.0000315282.61035.48
136. Derr RL, Ye X, Islas MU, Desideri S, Saudek CD, Grossman SA. Association between hyperglycemia and survival in patients with newly diagnosed glioblastoma. J Clin Oncol (2009) 27:1082–6. doi: 10.1200/JCO.2008.19.1098
137. Mayer A, Vaupel P, Struss H-G, Giese A, Stockinger M, Schmidberger H. Strong adverse prognostic impact of hyperglycemic episodes during adjuvant chemoradiotherapy of glioblastoma multiforme. Strahlenther und Onkol (2014) 190:933–8. doi: 10.1007/s00066-014-0696-z
138. Barami K, Lyon L, Conell C. Type 2 diabetes mellitus and glioblastoma multiforme–assessing risk and survival: results of a large retrospective study and systematic review of the literature. World Neurosurg (2017) 106:300–7. doi: 10.1016/j.wneu.2017.06.164
139. Diehl A, Yarchoan M, Hopkins A, Jaffee E, Grossman SA. Relationships between lymphocyte counts and treatment-related toxicities and clinical responses in patients with solid tumors treated with PD-1 checkpoint inhibitors. Oncotarget (2017) 8:114268–80. doi: 10.18632/oncotarget.23217
140. Ho WJ, Yarchoan M, Hopkins A, Mehra R, Grossman S, Kang H. Association between pretreatment lymphocyte count and response to PD1 inhibitors in head and neck squamous cell carcinomas. J Immunother Cancer (2018) 6:84. doi: 10.1186/s40425-018-0395-x
141. Césaire M, Rambeau A, Clatot F, Johnson A, Heutte N, Thariat J. Impact of lymphopenia on efficacy of nivolumab in head and neck cancer patients. Eur Arch Oto-Rhino-Laryngol (2023) 280:2453–61. doi: 10.1007/s00405-022-07800-1
142. Tomsitz D, Schlaak M, Zierold S, Pesch G, Schulz TU, Müller G, et al. Development of lymphopenia during therapy with immune checkpoint inhibitors is associated with poor outcome in metastatic cutaneous melanoma. Cancers (Basel) (2022) 14:3282. doi: 10.3390/cancers14133282
143. Mouritzen MT, Ladekarl M, Hager H, Mattesen TB, Lippert JB, Frank MS, et al. Gene expressions and high lymphocyte count may predict durable clinical benefits in patients with advanced non-small-cell lung cancer treated with immune checkpoint inhibitors. Cancers (Basel) (2023) 15:4480. doi: 10.3390/cancers15184480
144. Zhang Y, Huang C, Li S. Influence of treatment-related lymphopenia on the efficacy of immune checkpoint inhibitors in lung cancer: a meta-analysis. Front Oncol (2023) 13:1287555. doi: 10.3389/fonc.2023.1287555
145. Awada H, Paris F, Pecqueur C. Exploiting radiation immunostimulatory effects to improve glioblastoma outcome. Neuro Oncol (2023) 25:433–46. doi: 10.1093/neuonc/noac239
146. Koukourakis MI, Giatromanolaki A. Lymphopenia and intratumoral lymphocytic balance in the era of cancer immuno-radiotherapy. Crit Rev Oncol Hematol (2021) 159:103226. doi: 10.1016/j.critrevonc.2021.103226
147. Stepanenko AA, Andreieva SV, Korets KV, Mykytenko DO, Baklaushev VP, Huleyuk NL, et al. Temozolomide promotes genomic and phenotypic changes in glioblastoma cells. Cancer Cell Int (2016) 16:36. doi: 10.1186/s12935-016-0311-8
148. Johnson BE, Mazor T, Hong C, Barnes M, Aihara K, McLean CY, et al. Mutational analysis reveals the origin and therapy-driven evolution of recurrent glioma. Science (2014) 343:189–93. doi: 10.1126/science.1239947
149. Authier A, Farrand KJ, Broadley KWR, Ancelet LR, Hunn MK, Stone S, et al. Enhanced immunosuppression by therapy-exposed glioblastoma multiforme tumor cells. Int J Cancer (2015) 136:2566–78. doi: 10.1002/ijc.29309
150. Fritzell S, Sandén E, Eberstål S, Visse E, Darabi A, Siesjö P. Intratumoral temozolomide synergizes with immunotherapy in a T cell-dependent fashion. Cancer Immunol Immunother (2013) 62:1463–74. doi: 10.1007/s00262-013-1449-z
151. Rubner Y, Muth C, Strnad A, Derer A, Sieber R, Buslei R, et al. Fractionated radiotherapy is the main stimulus for the induction of cell death and of Hsp70 release of p53 mutated glioblastoma cell lines. Radiat Oncol (2014) 9:89. doi: 10.1186/1748-717X-9-89
152. Pasi F, Paolini A, Nano R, Di Liberto R, Capelli E. Effects of single or combined treatments with radiation and chemotherapy on survival and danger signals expression in glioblastoma cell lines. BioMed Res Int (2014) 2014:453497. doi: 10.1155/2014/453497
153. Garg AD, More S, Rufo N, Mece O, Sassano ML, Agostinis P, et al. Trial watch: Immunogenic cell death induction by anticancer chemotherapeutics. Oncoimmunology (2017) 6:e1386829. doi: 10.1080/2162402X.2017.1386829
154. Chew MT, Jones B, Hill M, Bradley DA. Radiation, a two-edged sword: From untoward effects to fractionated radiotherapy. Radiat Phys Chem (2021) 178:108994. doi: 10.1016/j.radphyschem.2020.108994
155. van Vloten JP, Workenhe ST, Wootton SK, Mossman KL, Bridle BW. Critical interactions between immunogenic cancer cell death, oncolytic viruses, and the immune system define the rational design of combination immunotherapies. J Immunol (2018) 200:450–8. doi: 10.4049/jimmunol.1701021
156. Pitt JM, Vétizou M, Daillère R, Roberti MP, Yamazaki T, Routy B, et al. Resistance mechanisms to immune-checkpoint blockade in cancer: tumor-intrinsic and -extrinsic factors. Immunity (2016) 44:1255–69. doi: 10.1016/j.immuni.2016.06.001
157. Wang F, Cathcart SJ, DiMaio DJ, Zhao N, Chen J, Aizenberg MR, et al. Comparison of tumor immune environment between newly diagnosed and recurrent glioblastoma including matched patients. J Neurooncol (2022) 159:163–75. doi: 10.1007/s11060-022-04053-0
158. Fu W, Wang W, Li H, Jiao Y, Huo R, Yan Z, et al. Single-cell atlas reveals complexity of the immunosuppressive microenvironment of initial and recurrent glioblastoma. Front Immunol (2020) 11:835. doi: 10.3389/fimmu.2020.00835
159. Magri S, Musca B, Bonaudo C, Tushe A, Russo MG, Masetto E, et al. Sustained accumulation of blood-derived macrophages in the immune microenvironment of patients with recurrent glioblastoma after therapy. Cancers (Basel) (2021) 13:6178. doi: 10.3390/cancers13246178
160. Kim Y-H, Jung T-Y, Jung S, Jang W-Y, Moon K-S, Kim I-Y, et al. Tumour-infiltrating T-cell subpopulations in glioblastomas. Br J Neurosurg (2012) 26:21–7. doi: 10.3109/02688697.2011.584986
161. Berghoff AS, Kiesel B, Widhalm G, Rajky O, Ricken G, Wöhrer A, et al. Programmed death ligand 1 expression and tumor-infiltrating lymphocytes in glioblastoma. Neuro Oncol (2015) 17:1064–75. doi: 10.1093/neuonc/nou307
162. Jacobs JFM, Idema AJ, Bol KF, Grotenhuis JA, de Vries IJM, Wesseling P, et al. Prognostic significance and mechanism of Treg infiltration in human brain tumors. J Neuroimmunol (2010) 225:195–9. doi: 10.1016/j.jneuroim.2010.05.020
163. Sayour EJ, McLendon P, McLendon R, De Leon G, Reynolds R, Kresak J, et al. Increased proportion of FoxP3+ regulatory T cells in tumor infiltrating lymphocytes is associated with tumor recurrence and reduced survival in patients with glioblastoma. Cancer Immunol Immunother (2015) 64:419–27. doi: 10.1007/s00262-014-1651-7
164. Rahman M, Kresak J, Yang C, Huang J, Hiser W, Kubilis P, et al. Analysis of immunobiologic markers in primary and recurrent glioblastoma. J Neurooncol (2018) 137(2):249–57. doi: 10.1007/s11060-017-2732-1
165. Alanio C, Binder ZA, Chang RB, Nasrallah MP, Delman D, Li JH, et al. Immunologic features in de novo and recurrent glioblastoma are associated with survival outcomes. Cancer Immunol Res (2022) 10:800–10. doi: 10.1158/2326-6066.CIR-21-1050
166. Pombo Antunes AR, Scheyltjens I, Lodi F, Messiaen J, Antoranz A, Duerinck J, et al. Single-cell profiling of myeloid cells in glioblastoma across species and disease stage reveals macrophage competition and specialization. Nat Neurosci (2021) 24:595–610. doi: 10.1038/s41593-020-00789-y
167. Houston EG, Higdon LE, Fink PJ. Recent thymic emigrants are preferentially incorporated only into the depleted T-cell pool. Proc Natl Acad Sci U.S.A. (2011) 108:5366–71. doi: 10.1073/pnas.1015286108
168. Fink PJ. The biology of recent thymic emigrants. Annu Rev Immunol (2013) 31:31–50. doi: 10.1146/annurev-immunol-032712-100010
169. Cunningham CA, Helm EY, Fink PJ. Reinterpreting recent thymic emigrant function: defective or adaptive? Curr Opin Immunol (2017) 51:1–6. doi: 10.1016/j.coi.2017.12.006
170. Wheeler CJ, Black KL, Liu G, Ying H, Yu JS, Zhang W, et al. Thymic CD8+ T cell production strongly influences tumor antigen recognition and age-dependent glioma mortality. J Immunol (2003) 171:4927–33. doi: 10.4049/jimmunol.171.9.4927
171. Blackman MA, Woodland DL. The narrowing of the CD8 T cell repertoire in old age. Curr Opin Immunol (2011) 23:537–42. doi: 10.1016/j.coi.2011.05.005
172. Mackall CL. T-cell immunodeficiency following cytotoxic antineoplastic therapy: A review. Stem Cells (2000) 18:10–8. doi: 10.1634/stemcells.18-1-10
173. Mathios D, Kim JE, Mangraviti A, Phallen J, Park C-K, Jackson CM, et al. Anti-PD-1 antitumor immunity is enhanced by local and abrogated by systemic chemotherapy in GBM. Sci Transl Med (2016) 8:370ra180–370ra180. doi: 10.1126/scitranslmed.aag2942
174. Litterman AJ, Dudek AZ, Largaespada DA. Alkylating chemotherapy may exert a uniquely deleterious effect upon neo-antigen-targeting anticancer vaccination. Oncoimmunology (2013) 2:e26294. doi: 10.4161/onci.26294
175. Litterman AJ, Zellmer DM, Grinnen KL, Hunt MA, Dudek AZ, Salazar AM, et al. Profound impairment of adaptive immune responses by alkylating chemotherapy. J Immunol (2013) 190:6259–68. doi: 10.4049/jimmunol.1203539
176. Candolfi M, Yagiz K, Wibowo M, Ahlzadeh GE, Puntel M, Ghiasi H, et al. Temozolomide does not impair gene therapy-mediated antitumor immunity in syngeneic brain tumor models. Clin Cancer Res (2014) 20:1555–65. doi: 10.1158/1078-0432.CCR-13-2140
177. Saha D, Rabkin SD, Martuza RL. Temozolomide antagonizes oncolytic immunovirotherapy in glioblastoma. J Immunother Cancer (2020) 8. doi: 10.1136/jitc-2019-000345
178. Tysome JR, Li X, Wang S, Wang P, Gao D, Du P, et al. A novel therapeutic regimen to eradicate established solid tumors with an effective induction of tumor-specific immunity. Clin Cancer Res (2012) 18:6679–89. doi: 10.1158/1078-0432.CCR-12-0979
179. Wang P, Li X, Wang J, Gao D, Li Y, Li H, et al. Re-designing Interleukin-12 to enhance its safety and potential as an anti-tumor immunotherapeutic agent. Nat Commun (2017) 8:1395. doi: 10.1038/s41467-017-01385-8
180. Kleijn A, van den Bossche W, Haefner ES, Belcaid Z, Burghoorn-Maas C, Kloezeman JJ, et al. The sequence of delta24-RGD and TMZ administration in Malignant glioma affects the role of CD8(+)T cell anti-tumor activity. Mol Ther oncol (2017) 5:11–9. doi: 10.1016/j.omto.2017.02.002
181. Koks CA, Garg AD, Ehrhardt M, Riva M, Vandenberk L, Boon L, et al. Newcastle disease virotherapy induces long-term survival and tumor-specific immune memory in orthotopic glioma through the induction of immunogenic cell death. Int J Cancer (2015) 136:E313–25. doi: 10.1002/ijc.29202
182. Garg AD, Vandenberk L, Koks C, Verschuere T, Boon L, Van Gool SW, et al. Dendritic cell vaccines based on immunogenic cell death elicit danger signals and T cell-driven rejection of high-grade glioma. Sci Transl Med (2016) 8:328ra27–328ra27. doi: 10.1126/scitranslmed.aae0105
183. Saha D, Martuza RL, Rabkin SD. Macrophage polarization contributes to glioblastoma eradication by combination immunovirotherapy and immune checkpoint blockade. Cancer Cell (2017) 32:253–267.e5. doi: 10.1016/j.ccell.2017.07.006
184. Curtin JF, Liu N, Candolfi M, Xiong W, Assi H, Yagiz K, et al. HMGB1 mediates endogenous TLR2 activation and brain tumor regression. PloS Med (2009) 6:e10. doi: 10.1371/journal.pmed.1000010
185. Curtin JF, Candolfi M, Fakhouri TM, Liu C, Alden A, Edwards M, et al. Treg depletion inhibits efficacy of cancer immunotherapy: implications for clinical trials. PloS One (2008) 3:e1983. doi: 10.1371/journal.pone.0001983
186. Li X, Wang P, Li H, Du X, Liu M, Huang Q, et al. The efficacy of oncolytic adenovirus is mediated by T-cell responses against virus and tumor in Syrian hamster model. Clin Cancer Res (2017) 23:239–49. doi: 10.1158/1078-0432.CCR-16-0477
187. Eoli M, Corbetta C, Anghileri E, Di Ianni N, Milani M, Cuccarini V, et al. Expansion of effector and memory T cells is associated with increased survival in recurrent glioblastomas treated with dendritic cell immunotherapy. Neuro-Oncol Adv (2019) 1:vdz022. doi: 10.1093/noajnl/vdz022
188. Keskin DB, Anandappa AJ, Sun J, Tirosh I, Mathewson ND, Li S, et al. Neoantigen vaccine generates intratumoral T cell responses in phase Ib glioblastoma trial. Nature (2019) 565:234–9. doi: 10.1038/s41586-018-0792-9
189. Löhr M, Freitag B, Technau A, Krauss J, Monoranu C-M, Rachor J, et al. High-grade glioma associated immunosuppression does not prevent immune responses induced by therapeutic vaccines in combination with Treg depletion. Cancer Immunol Immunother (2018) 67:1545–58. doi: 10.1007/s00262-018-2214-0
190. Van Gool SW, Makalowski J, Bitar M, Van de Vliet P, Schirrmacher V, Stuecker W. Synergy between TMZ and individualized multimodal immunotherapy to improve overall survival of IDH1 wild-type MGMT promoter-unmethylated GBM patients. Genes Immun (2022) 23:255–9. doi: 10.1038/s41435-022-00162-y
191. Karachi A, Dastmalchi F, Mitchell DA, Rahman M. Temozolomide for immunomodulation in the treatment of glioblastoma. Neuro Oncol (2018) 20:1566–72. doi: 10.1093/neuonc/noy072
192. Kumar N, Kumar R, Sharma SC, Mukherjee A, Khandelwal N, Tripathi M, et al. Impact of volume of irradiation on survival and quality of life in glioblastoma: a prospective, phase 2, randomized comparison of RTOG and MDACC protocols. Neuro-oncol Pract (2020) 7:86–93. doi: 10.1093/nop/npz024
193. Rudra S, Hui C, Rao YJ, Samson P, Lin AJ, Chang X, et al. Effect of radiation treatment volume reduction on lymphopenia in patients receiving chemoradiotherapy for glioblastoma. Int J Radiat Oncol Biol Phys (2018) 101:217–25. doi: 10.1016/j.ijrobp.2018.01.069
194. Kut C, Kleinberg L. Radiotherapy, lymphopenia and improving the outcome for glioblastoma: a narrative review. Chin Clin Oncol (2023) 12:4. doi: 10.21037/cco-22-94
195. Lambin P, Lieverse RIY, Eckert F, Marcus D, Oberije C, van der Wiel AMA, et al. Lymphocyte-sparing radiotherapy: the rationale for protecting lymphocyte-rich organs when combining radiotherapy with immunotherapy. Semin Radiat Oncol (2020) 30:187–93. doi: 10.1016/j.semradonc.2019.12.003
196. Nishioka K, Takahashi S, Mori T, UChinami Y, Yamaguchi S, Kinoshita M, et al. The need of radiotherapy optimization for glioblastomas considering immune responses. Jpn J Radiol (2023) 41(10):1062–71. doi: 10.1007/s11604-023-01434-x
197. Mireştean CC, Iancu RI, Iancu DT. Immunotherapy and radiotherapy as an antitumoral long-range weapon—A partnership with unsolved challenges: dose, fractionation, volumes, therapeutic sequence. Curr Oncol (2022) 29:7388–95. doi: 10.3390/curroncol29100580
198. Kim N, Lim DH, Choi JW, Lee J-I, Kong D-S, Seol HJ, et al. Clinical outcomes of moderately hypofractionated concurrent chemoradiotherapy for newly diagnosed glioblastoma. Yonsei Med J (2023) 64:94–103. doi: 10.3349/ymj.2022.0352
199. Crocenzi T, Cottam B, Newell P, Wolf RF, Hansen PD, Hammill C, et al. A hypofractionated radiation regimen avoids the lymphopenia associated with neoadjuvant chemoradiation therapy of borderline resectable and locally advanced pancreatic adenocarcinoma. J Immunother Cancer (2016) 4:45. doi: 10.1186/s40425-016-0149-6
200. Roa W, Brasher PMA, Bauman G, Anthes M, Bruera E, Chan A, et al. Abbreviated course of radiation therapy in older patients with glioblastoma multiforme: A prospective randomized clinical trial. J Clin Oncol (2004) 22:1583–8. doi: 10.1200/JCO.2004.06.082
201. Trone J-C, Vallard A, Sotton S, Ben Mrad M, Jmour O, Magné N, et al. Survival after hypofractionation in glioblastoma: a systematic review and meta-analysis. Radiat Oncol (2020) 15:145. doi: 10.1186/s13014-020-01584-6
202. Wild AT, Herman JM, Dholakia AS, Moningi S, Lu Y, Rosati LM, et al. Lymphocyte-sparing effect of stereotactic body radiation therapy in patients with unresectable pancreatic cancer. Int J Radiat Oncol (2016) 94:571–9. doi: 10.1016/j.ijrobp.2015.11.026
203. Mohan R, Liu AY, Brown PD, Mahajan A, Dinh J, Chung C, et al. Proton therapy reduces the likelihood of high-grade radiation-induced lymphopenia in glioblastoma patients: phase II randomized study of protons vs photons. Neuro Oncol (2021) 23:284–94. doi: 10.1093/neuonc/noaa182
204. Shiraishi Y, Fang P, Xu C, Song J, Krishnan S, Koay EJ, et al. Severe lymphopenia during neoadjuvant chemoradiation for esophageal cancer: A propensity matched analysis of the relative risk of proton versus photon-based radiation therapy. Radiother Oncol (2018) 128:154–60. doi: 10.1016/j.radonc.2017.11.028
205. Baumann BC, Mitra N, Harton JG, Xiao Y, Wojcieszynski AP, Gabriel PE, et al. Comparative effectiveness of proton vs photon therapy as part of concurrent chemoradiotherapy for locally advanced cancer. JAMA Oncol (2020) 6:237. doi: 10.1001/jamaoncol.2019.4889
206. Choi JI, Simone CB, Lozano A, Frank SJ. Advances and challenges in conducting clinical trials with proton beam therapy. Semin Radiat Oncol (2023) 33:407–15. doi: 10.1016/j.semradonc.2023.06.006
207. Goff KM, Zheng C, Alonso-Basanta M. Proton radiotherapy for glioma and glioblastoma. Chin Clin Oncol (2022) 11:46–6. doi: 10.21037/cco-22-92
208. Galluzzi L, Aryankalayil MJ, Coleman CN, Formenti SC. Emerging evidence for adapting radiotherapy to immunotherapy. Nat Rev Clin Oncol (2023) 20:543–57. doi: 10.1038/s41571-023-00782-x
209. Liu D, Yang T, Ma W, Wang Y. Clinical strategies to manage adult glioblastoma patients without MGMT hypermethylation. J Cancer (2022) 13:354–63. doi: 10.7150/jca.63595
210. Frederico SC, Darling C, Bielanin JP, Dubinsky AC, Zhang X, Hadjipanayis CG, et al. Neoadjuvant immune checkpoint inhibition in the management of glioblastoma: Exploring a new frontier. Front Immunol (2023) 14:1057567. doi: 10.3389/fimmu.2023.1057567
211. Ogino H, Taylor JW, Nejo T, Gibson D, Watchmaker PB, Okada K, et al. Randomized trial of neoadjuvant vaccination with tumor-cell lysate induces T cell response in low-grade gliomas. J Clin Invest (2022) 132. doi: 10.1172/JCI151239
212. Waqar M, Trifiletti DM, McBain C, O’Connor J, Coope DJ, Akkari L, et al. Early therapeutic interventions for newly diagnosed glioblastoma: rationale and review of the literature. Curr Oncol Rep (2022) 24:311–24. doi: 10.1007/s11912-021-01157-0
213. Duerinck J, Tuyaerts S, Movahedi K, Neyns B. Overcoming the immune suppressive nature of glioblastoma by leveraging the surgical intervention - current status and future perspectives. Front Immunol (2023) 14:1183641. doi: 10.3389/fimmu.2023.1183641
214. Díez Valle R, Becerra Castro V, Marigil Sánchez M, Gállego Pérez-Larraya J, Núñez-Córdoba JM, Tejada Solis S. Results of a policy of fast tapering of steroids after resection surgery in glioblastoma. World Neurosurg (2018) 109:e845–52. doi: 10.1016/j.wneu.2017.10.110
215. Jessurun CAC, Hulsbergen AFC, Cho LD, Aglio LS, Nandoe Tewarie RDS, Broekman MLD. Evidence-based dexamethasone dosing in Malignant brain tumors: what do we really know? J Neurooncol (2019) 144:249–64. doi: 10.1007/s11060-019-03238-4
216. Lee EQ, Wen PY. Corticosteroids for peritumoral edema: time to overcome our addiction? Neuro Oncol (2016) 18:1191–2. doi: 10.1093/neuonc/now167
217. Ly KI, Wen PY. Clinical relevance of steroid use in neuro-oncology. Curr Neurol Neurosci Rep (2017) 17:5. doi: 10.1007/s11910-017-0713-6
218. Arvold ND, Armstrong TS, Warren KE, Chang SM, DeAngelis LM, Blakeley J, et al. Corticosteroid use endpoints in neuro-oncology: Response Assessment in Neuro-Oncology Working Group. Neuro Oncol (2018) 20:897–906. doi: 10.1093/neuonc/noy056
219. Bryan AM, Del Poeta M. Sphingosine-1-phosphate receptors and innate immunity. Cell Microbiol (2018) 20:e12836. doi: 10.1111/cmi.12836
220. Mahajan-Thakur S, Bien-Möller S, Marx S, Schroeder H, Rauch BH. Sphingosine 1-phosphate (S1P) signaling in glioblastoma multiforme-A systematic review. Int J Mol Sci (2017) 18:2448. doi: 10.3390/ijms18112448
221. Ghosh S, Huang J, Inkman M, Zhang J, Thotala S, Tikhonova E, et al. Radiation-induced circulating myeloid-derived suppressor cells induce systemic lymphopenia after chemoradiotherapy in patients with glioblastoma. Sci Transl Med (2023) 15. doi: 10.1126/scitranslmed.abn6758
222. Byun HK, Chung SY, Kim K-J, Seong J. Role of interleukin-7 in the development of and recovery from radiation-induced lymphopenia: A post-hoc analysis of a prospective cohort. Cancer Res Treat (2021) 53:962–72. doi: 10.4143/crt.2020.1053
223. Lee SW, Choi D, Heo M, Shin E, Park S, Kim SJ, et al. hIL-7-hyFc, A long-acting IL-7, increased absolute lymphocyte count in healthy subjects. Clin Transl Sci (2020) 13:1161–9. doi: 10.1111/cts.12800
224. Ahn S, Park J-S, Kim H, Heo M, Sung YC, Jeun S-S. Compassionate use of recombinant human IL-7-hyFc as a salvage treatment for restoring lymphopenia in patients with recurrent glioblastoma. Cancer Med (2023) 12:6778–87. doi: 10.1002/cam4.5467
225. Erhart F, Buchroithner J, Reitermaier R, Fischhuber K, Klingenbrunner S, Sloma I, et al. Immunological analysis of phase II glioblastoma dendritic cell vaccine (Audencel) trial: immune system characteristics influence outcome and Audencel up-regulates Th1-related immunovariables. Acta Neuropathol Commun (2018) 6:135. doi: 10.1186/s40478-018-0621-2
226. Bota DA, Chung J, Dandekar M, Carrillo JA, Kong X-T, Fu BD, et al. Phase II study of ERC1671 plus bevacizumab versus bevacizumab plus placebo in recurrent glioblastoma: interim results and correlations with CD4 + T-lymphocyte counts. CNS Oncol (2018) 7(3):CNS22. doi: 10.2217/cns-2018-0009
227. Pellegatta S, Eoli M, Frigerio S, Antozzi C, Bruzzone MG, Cantini G, et al. The natural killer cell response and tumor debulking are associated with prolonged survival in recurrent glioblastoma patients receiving dendritic cells loaded with autologous tumor lysates. Oncoimmunology (2013) 2:e23401. doi: 10.4161/onci.23401
228. Fong B, Jin R, Wang X, Safaee M, Lisiero DN, Yang I, et al. Monitoring of Regulatory T Cell Frequencies and Expression of CTLA-4 on T Cells, before and after DC Vaccination, Can Predict Survival in GBM Patients. PloS One (2012) 7:e32614. doi: 10.1371/journal.pone.0032614
229. Wheeler CJ, Black KL, Liu G, Mazer M, Zhang X-x, Pepkowitz S, et al. Vaccination elicits correlated immune and clinical responses in glioblastoma multiforme patients. Cancer Res (2008) 68:5955–64. doi: 10.1158/0008-5472.CAN-07-5973
230. Everson RG, Jin RM, Wang X, Safaee M, Scharnweber R, Lisiero DN, et al. Cytokine responsiveness of CD8+ T cells is a reproducible biomarker for the clinical efficacy of dendritic cell vaccination in glioblastoma patients. J Immunother Cancer (2014) 2:10. doi: 10.1186/2051-1426-2-10
231. Hsu M, Sedighim S, Wang T, Antonios JP, Everson RG, Tucker AM, et al. TCR sequencing can identify and track glioma-infiltrating T cells after DC vaccination. Cancer Immunol Res (2016) 4:412–8. doi: 10.1158/2326-6066.CIR-15-0240
232. Jan C-I, Tsai W-C, Harn H-J, Shyu W-C, Liu M-C, Lu H-M, et al. Predictors of response to autologous dendritic cell therapy in glioblastoma multiforme. Front Immunol (2018) 9:727. doi: 10.3389/fimmu.2018.00727
233. Yao Y, Luo F, Tang C, Chen D, Qin Z, Hua W, et al. Molecular subgroups and B7-H4 expression levels predict responses to dendritic cell vaccines in glioblastoma: an exploratory randomized phase II clinical trial. Cancer Immunol Immunother (2018) 67(11):1777–88. doi: 10.1007/s00262-018-2232-y
234. Bloch O, Lim M, Sughrue ME, Komotar RJ, Abrahams JM, O’Rourke DM, et al. Autologous heat shock protein peptide vaccination for newly diagnosed glioblastoma: impact of peripheral PD-L1 expression on response to therapy. Clin Cancer Res (2017) 23:3575–84. doi: 10.1158/1078-0432.CCR-16-1369
235. Fadul CE, Fisher JL, Hampton TH, Lallana EC, Li Z, Gui J, et al. Immune response in patients with newly diagnosed glioblastoma multiforme treated with intranodal autologous tumor lysate-dendritic cell vaccination after radiation chemotherapy. J Immunother (2011) 34:382–9. doi: 10.1097/CJI.0b013e318215e300
236. Rühle PF, Goerig N, Wunderlich R, Fietkau R, Gaipl US, Strnad A, et al. Modulations in the peripheral immune system of glioblastoma patient is connected to therapy and tumor progression—A case report from the IMMO-GLIO-01 trial. Front Neurol (2017) 8:296. doi: 10.3389/fneur.2017.00296
237. Desjardins A, Gromeier M, Herndon JE, Beaubier N, Bolognesi DP, Friedman AH, et al. Recurrent glioblastoma treated with recombinant poliovirus. N Engl J Med (2018) 379:150–61. doi: 10.1056/NEJMoa1716435
238. Holl EK, Frazier VN, Landa K, Beasley GM, Hwang ES, Nair SK. Examining peripheral and tumor cellular immunome in patients with cancer. Front Immunol (2019) 10:1767. doi: 10.3389/fimmu.2019.01767
239. Bagley SJ, Kothari S, Rahman R, Lee EQ, Dunn GP, Galanis E, et al. Glioblastoma clinical trials: current landscape and opportunities for improvement. Clin Cancer Res (2022) 28:594–602. doi: 10.1158/1078-0432.CCR-21-2750
240. Mallick S VRA, Giridhar P, Upadhyay R, Kim B-K, Sharma A, Elghazawy H, et al. A systematic review and meta-analysis of the impact of radiation-related lymphopenia on outcomes in high-grade gliomas. South Asian J Cancer (2022) 11:361–9. doi: 10.1055/s-0042-1753504
241. Elbers JBW, Gunsch PA, Debets R, Keereweer S, van Meerten E, Zindler J, et al. HYpofractionated, dose-redistributed RAdiotherapy with protons and photons to combat radiation-induced immunosuppression in head and neck squamous cell carcinoma: study protocol of the phase I HYDRA trial. BMC Cancer (2023) 23:541. doi: 10.1186/s12885-023-11031-w
242. Yang C, Hu B-W, Tang F, Zhang Q, Quan W, Wang J, et al. Prognostic value of systemic immune-inflammation index (SII) in patients with glioblastoma: A comprehensive study based on meta-analysis and retrospective single-center analysis. J Clin Med (2022) 11:7514. doi: 10.3390/jcm11247514
243. Bispo RG, Bastos Siqueira IF, de Oliveira BFS, Moreira Fernandes CE, Figueiredo LA, Cintra LP, et al. Prognostic value of the platelet-lymphocyte ratio for glioblastoma: A systematic review. World Neurosurg (2023) 175:137–141.e1. doi: 10.1016/j.wneu.2023.04.086
244. Wang Y, Xu C, Zhang Z. Prognostic value of pretreatment lymphocyte-to-monocyte ratio in patients with glioma: a meta-analysis. BMC Med (2023) 21:486. doi: 10.1186/s12916-023-03199-6
245. Zhang S, Ni Q. Prognostic role of the pretreatment systemic immune-inflammation index in patients with glioma: A meta-analysis. Front Neurol (2023) 14:1094364. doi: 10.3389/fneur.2023.1094364
246. Topkan E, Kucuk A, Ozdemir Y, Mertsoylu H, Besen AA, Sezen D, et al. Systemic inflammation response index predicts survival outcomes in glioblastoma multiforme patients treated with standard stupp protocol. J Immunol Res (2020) 2020:1–10. doi: 10.1155/2020/8628540
247. He Q, Li L, Ren Q. The prognostic value of preoperative systemic inflammatory response index (SIRI) in patients with high-grade glioma and the establishment of a nomogram. Front Oncol (2021) 11:671811. doi: 10.3389/fonc.2021.671811
248. Wang Z, Li J, Yuan Y, Li T, Zuo M, Liu Y. Prognostic significance of preoperative systemic inflammation response index in newly diagnosed glioblastoma patients underwent gross total resection: a propensity score matching analysis. World J Surg Oncol (2022) 20:137. doi: 10.1186/s12957-022-02588-0
Keywords: glioblastoma, immunotherapy, lymphopenia, oncolytic virotherapy, radiotherapy, steroid dexamethasone, temozolomide chemotherapy, total lymphocyte count
Citation: Stepanenko AA, Sosnovtseva AO, Valikhov MP, Chernysheva AA, Abramova OV, Naumenko VA and Chekhonin VP (2024) The need for paradigm shift: prognostic significance and implications of standard therapy-related systemic immunosuppression in glioblastoma for immunotherapy and oncolytic virotherapy. Front. Immunol. 15:1326757. doi: 10.3389/fimmu.2024.1326757
Received: 23 October 2023; Accepted: 23 January 2024;
Published: 08 February 2024.
Edited by:
Dorota Lubanska, University of Windsor, CanadaReviewed by:
Stuart Grossman, Johns Hopkins University, United StatesLawrence Kleinberg, Johns Hopkins University, United States
Copyright © 2024 Stepanenko, Sosnovtseva, Valikhov, Chernysheva, Abramova, Naumenko and Chekhonin. This is an open-access article distributed under the terms of the Creative Commons Attribution License (CC BY). The use, distribution or reproduction in other forums is permitted, provided the original author(s) and the copyright owner(s) are credited and that the original publication in this journal is cited, in accordance with accepted academic practice. No use, distribution or reproduction is permitted which does not comply with these terms.
*Correspondence: Aleksei A. Stepanenko, a.a.stepanenko@gmail.com