- Allergic Dermatoses Clinical Center, Shanghai Skin Disease Hospital, Tongji University School of Medicine, Shanghai, China
The development of immune checkpoint inhibitors (ICIs) has dramatically altered the landscape of therapy for multiple malignancies, including urothelial carcinoma, non-small cell lung cancer, melanoma and gastric cancer. As part of their anti-tumor properties, ICIs can enhance susceptibility to inflammatory side effects known as immune-related adverse events (irAEs), in which the skin is one of the most commonly and rapidly affected organs. Although numerous questions still remain unanswered, multi-omics technologies have shed light into immunological mechanisms, as well as the correlation between ICI-induced activation of immune systems and the incidence of cirAE (cutaneous irAEs). Therefore, we reviewed integrated biological layers of omics studies combined with clinical data for the prediction biomarkers of cirAEs based on skin pathogenesis. Here, we provide an overview of a spectrum of dermatological irAEs, discuss the pathogenesis of this “off-tumor toxicity” during ICI treatment, and summarize recently investigated biomarkers that may have predictive value for cirAEs via multi-omics approach. Finally, we demonstrate the prognostic significance of cirAEs for immune checkpoint blockades.
1 Introduction
The development of immune checkpoint inhibitors (ICIs), such as monoclonal antibodies targeting programmed death-1 (PD-1)/programmed death-ligand 1 (PD-L1) and cytotoxic T-lymphocyte-associated antigen 4 (CTLA-4), has dramatically changed the landscape of therapy for multiple malignancies. ICIs represent one type of immune therapy for cancer, among other options such as, surgery, chemotherapy, radiotherapy, targeted therapy and immune therapy. In contrast to other therapies that use toxic chemical or physical agents to kill tumors, immunotherapy aims to harness the immune response. Immunotherapy is premised based on the theory that the immune system should be able to eliminate tumors, but the tumors ‘escape’ by some mechanisms, t‘immunoediting’ (1, 2). Accordingly, immunotherapy kills tumors by enhancing the anti-tumor ability of the immune system or by inhibiting tumor immunoediting. It has been established that checkpoints are some regulators of immune response, and tumors could specifically stimulate some negative checkpoints to suppress the immune response, thus escaping (3). Therefore, the immune system may be used to target tumors by inhibiting negative checkpoints, such as CTLA-4 and PD-1/PD-L1.
The intervention of immune homeostasis by ICIs can enhance the anti-tumor function of the immune system, while also leading to some adverse effects resulting from the systemic immune overactivation (Table 1). These unwanted effects are often termed immune-related adverse events (irAEs). Among all the related organs and systems, the skin is one of the most common targets, of which cutaneous irAEs (cirAEs) are often the first to manifest (5). Since the suppressive effects of checkpoints on the immune response are inhibited by ICIs, lymphocytes become over-activated (22, 23), pro-inflammatory cytokines are abundantly released (24, 25), and immune tolerance is destroyed (26, 27), all of which may contribute to the irAEs. These adverse events present a challenge for cancer patients receiving ICIs and can even force them to withdraw from ICI therapy. However, the precise mechanism of irAE remains unknown, and treatment primarily comprises immunosuppressants, such as glucocorticoids (28). Although there is scant evidence showing that the application of immunosuppressors can offset the anti-tumor effect of ICIs (29), the development of new adverse events (e.g., opportunistic infection, hyperglycemia, fluid retention, anxiety, and osteoporosis) should not be ignored over the long term (30, 31).
Since cirAEs occur often and early, influencing the life quality of patients, which reduces patient compliance to ICIs, the treatment of which also gives rise to a series of new problems, there is an urgent need to identify predictive biomarkers of cirAEs. Several risk factors have been identified by epidemiological investigation, and the serum levels of several molecules in patients suffering from irAEs have been found to exhibit significant differences compared to those without irAEs (Table 2). However, none of these biomarkers have shown satisfying prediction efficacy, which may be due to the heterogeneity and complex mechanisms of irAEs. The recent advent of multi-omics, a combined technology including genomics, transcriptomics, proteomics and metabolomics, has been associated with substantial progress for revealing the mechanism and predicting irAEs. Analyzing the genome helps us to find mutations that are responsible for ICI-resistance and irAEs, thus contributing to uncovering the mechanism of irAEs and predicting the risk. Regarding to the heterogeneity, transcriptomics deals with the distinct expression of genes, providing a context-dependent understanding of what actually occur in the anti-tumor immunity, and proteomics provides functional insight into genomics. Moreover, since the metabolic reprogramming is a hallmark of cancers, which is associated with the tumorigenesis, progression, metastasis and drug-resistance, the screening for metabolomics reveals the current condition or status, helping to determine whether the tumors are responsive to ICIs and whether the immune homeostasis is disturbed to elicit adverse events (51–53). From a systemic biology perspective, a macroscopic immune network has gradually been uncovered and additional molecules that were previously unknown have been identified as biomarkers for the prediction of both anti-tumor efficacy and irAEs.
2 Epidemiology and clinical manifestations
Cutaneous irAEs is one of the most common types of irAEs, with regards to morbidity. cirAEs arise in as many as 34% of patients receiving anti-PD-1/PD-L1 therapy and about 43% −45% of those on CTLA-4 inhibitors (54). The incidence of cirAEs varies among the patients suffering from different types of cancers, and even different pathological subtypes and different stages of certain cancers (55). Distinct types of ICIs can also lead to distinct incidence of cirAEs (56). Nevertheless, cirAEs commonly manifest as maculopapular rash, psoriasiform rash, bullous pemphigoid (BP), vitiligo, pruritus, eczema, and Stevens-Johnson syndrome (SJS), toxic epidermal necrolysis (TEN), drug reaction with eosinophilia and systemic symptoms (DRESS) less commonly (13). Cutaneous irAEs occur early, with the time to onset ranging from 3 to 4 weeks (57), compared to 12 weeks in the endocrine gland (58) and 22.2 weeks in the gastrointestinal tract (59). Therefore, for the common and early onset and suffering manifestations, there is an urgent need to investigate cirAEs, uncovering its mechanism, and identifying its predictive biomarkers. Such information will serve to relieve the pain of patients as well as contribute to cancer therapy.
3 Mechanisms
ICIs are agents that block the interaction between checkpoints and the associated ligands and thereby block the subsequent intracellular signaling. The most commonly used ICIs target PD-1/PD-L1, with others targeting of CTLA-4, Tim-3, and LAG-3. Although both CTLA-4 and PD-1/PD-L1 are negative checkpoints, they play different roles in regulating the immune response, thus leading to different adverse events once blocked. CTLA-4 is a competitive inhibitor of CD28, a co-stimulatory signal receptor that is essential for T cell activation (Figure 1A). CTLA-4 is considered to be the most important negative checkpoint, as murine animals lacking CTLA-4 will die at an early age due to severe lymphoproliferation (60). Moreover, regulatory T cells (Treg) also function via CTLA-4 expression, competitively binding to B7 expressed on antigen presenting cells (APCs), which blocks its co-stimulatory effects on naïve T cells (61). PD-1 is one of the inhibitory receptors that contain an immunoreceptor tyrosine-based inhibition motif (ITIM) or the related immunoreceptor tyrosine-based switch motif (ITSM), which could remove the phosphates once activated and thereby inhibit the signaling (Figure 1B). PD-1 is able to bind with PD-L1 and PD-L2, which are constitutively expressed by a variety of cells and inductively expressed on APCs during inflammation, respectively (62, 63). Regulating the expression of PD-1 can control the intensity of the immune response, as pro-inflammatory cytokines have been shown to down-regulate PD-1 expression and murine models lacking PD-1 tend to develop auto-immune diseases (64). Therefore, various checkpoints substantially contribute to the regulation of the immune response and tolerance. Medications that affect checkpoints may lead to a disorder in immune homeostasis.
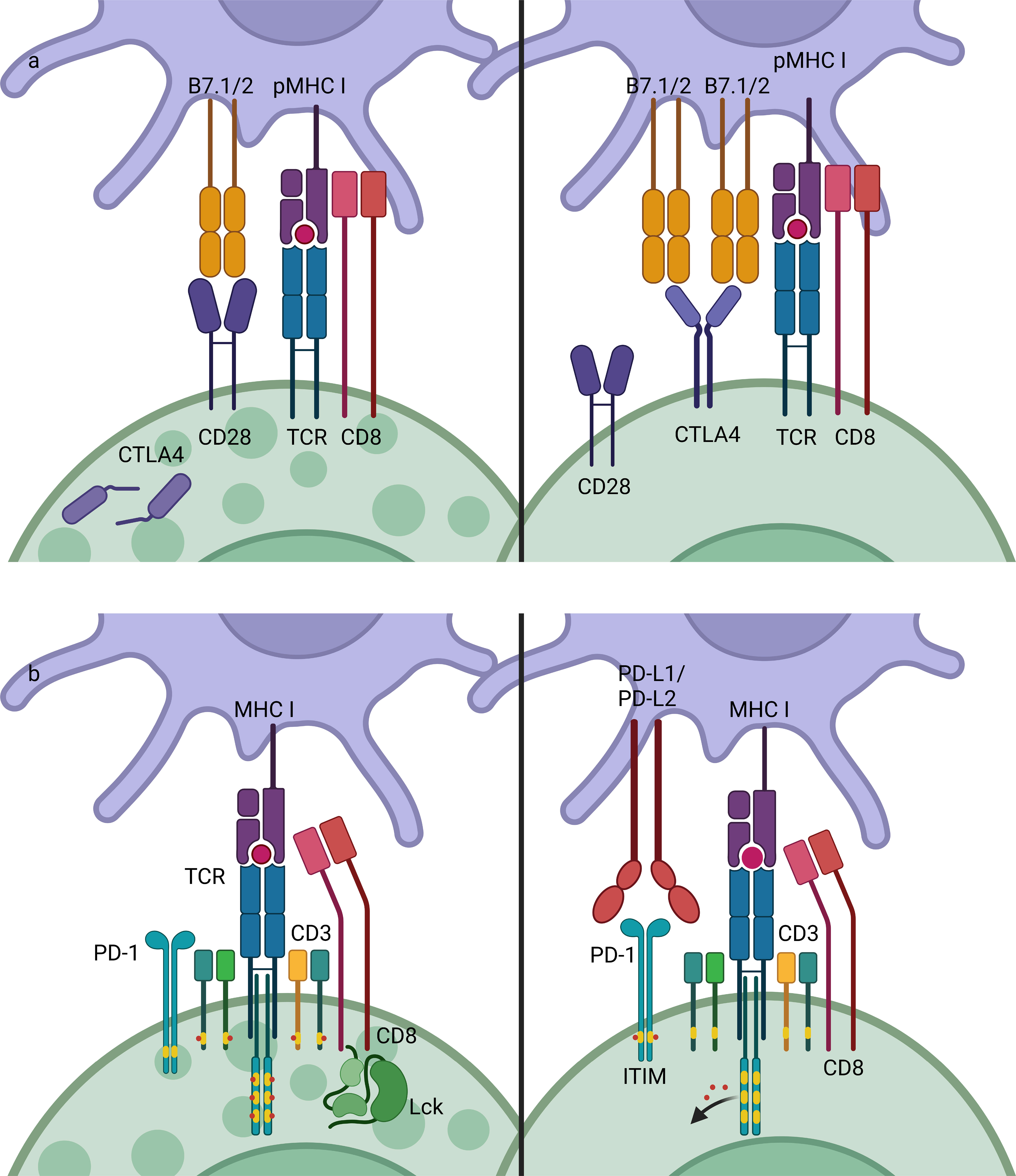
Figure 1 Mechanisms of CTLA-4 and PD-1/PD-L1.CTLA-4 and PD-1 are both negative checkpoints that inhibit the activation of lymphocytes. (A) T cells can be activated by APCs by pMHC-TCR (signal 1) and co-stimulatory molecules such as B7-CD28 (signal 2). CTLA-4 is competitive inhibitor of CD28, which has higher affinity to B7, thus inhibiting the co-stimulatory signaling in T cells activation. (B) PD-1 is an inhibitory receptor that contains an ITIM or ITSM, which mediate dephosphorylation reaction once activated, thus stopping the activation signaling transduction. CTLA-4, cytotoxic T-lymphocyte-associated antigen 4; PD-1, programmed death-1; PD-L1, programmed death-ligand 1; MHC, major histocompatibility complex; TCR, T cell receptor; ITIM, immunoreceptor tyrosine-based inhibition motif; ITSM, immunoreceptor tyrosine-based switch motif.
Having established a macroscopic overview of the action of ICIs in the immune response, we will now discuss the mechanism of irAEs, especially cirAEs. In general, irAEs are primarily induced by the overactivation of the immune response due to a blockades of negative regulators, and auto-immune responses are activated as a result. As previously discussed, cirAEs manifest commonly and early, indicating that cirAEs have some distinct characteristics other than the common mechanism of irAEs. Here, we propose that the commensal microbiota and the distinct characteristics of cutaneous immune system may be the issue. Next, we will first demonstrate the role of auto-immune response in irAEs, followed by a discussion of the commensal microbiota and the characteristics of cutaneous immunity. Finally, we will discuss genetic factors.
3.1 Autoimmunity
An important mechanism of irAEs is the autoimmune response, which is the immune response that targets self-antigens. Many irAEs are considered to be or appear to mimic autoimmune diseases, including myocarditis (65), diabetes mellitus (66), hypothyroidism (67), pneumonitis (68), rheumatoid arthritis (69), vitiligo (70), BP (10) and psoriasis (71). The association between checkpoints and autoimmunity has previously been confirmed by the Genome-Wide Association Study (GWAS), in which mutations in the CTLA-4 and PD-1/PD-L1 genes were identified to be responsible for several autoimmune diseases, such as Grave’s Disease and systemic lupus erythematosus (SLE) (72, 73). Other studies have also demonstrated that the IL-27-mediated priming of naïve T cells could upregulate the expression of PD-L1, which inhibited the differentiation of CD4+ T cells into a Th17 phenotype, thereby exhibiting protection against autoimmune diseases (74). The mechanism of this protection also involved a blockade of the TCR from binding to dendritic cells (DCs) through the interaction of PD-1 and PD-L1 (75). Moreover, PD-L1 were found to be abundantly expressed on pancreatic β-cells to avoid autoimmune attack (76). Therefore, a PD-1/PD-L1 blockade may induce autoimmune diabetes (type 1 diabetes mellitus, T1DM) by destroying this tolerance. Pdcd1-/-Ctla4+/- mice can be used as the model to study ICI-associated myocarditis (ICI-MC) (77). Moreover, the engineered expression of PD-1/PD-L1 have been used for the treatment of several immune diseases, including arthritis, colitis, and T1DM (78, 79). This indispensable role of PD-1/PD-L1 for preventing autoimmunity was also confirmed by a clinical research in a patient with an inherited PD-1 deficiency, diagnosed with T1DM, hypothyroidism, and idiopathic arthritis, who was dying from severe pulmonary autoimmunity (80). In fact, although the mechanism of ‘central tolerance’ eliminates most of the lymphocytes which could be activated by self-antigens, self-reactive lymphocytes always exist in the natural immune repertoire but do not elicit remarkable autoimmune diseases, due partly to the lack of activation signal (also termed as ‘second signal’) and the action of negative checkpoints such as PD-1/PD-L1, termed ‘peripheral tolerance’, thus keeping a balance between preventing infection and preventing autoimmunity. In some contexts, infection is a common trigger of autoimmune diseases because it leads to an abundant release of pro-inflammatory cytokines, which act as activation signals towards self-reactive lymphocytes. The application of ICIs may also activate the autoimmune response by liberating self-reactive lymphocytes from the inhibitory control of negative checkpoints. Therefore, in this manner, irAEs of ICIs often appear to mimic classic autoimmune diseases. Since self-active T cells can induce the autoimmune response via two methods, exhibiting direct cytotoxicity and facilitating B cell-induced immune response, auto-antibodies are also involved in the irAEs, which is in line with previous literature. The hypophysitis and diabetes mellitus induced by anti-CTLA-4 and anti-PD-1/PD-L1, respectively, can serve as examples of cases in which auto-antibodies, while undetectable at baseline, developed significantly following treatment with ICIs (64, 81). Another important autoimmune disease is bullous pemphigoid (BP), which is associated with impaired basement membrane zone (BMZ) caused by auto-antibodies targeting BP180 and BP230. Trauma, burn or radiation may elicit BP by destroying the immune barrier which leads to the exposure of self-antigen to self-active lymphocytes, and application of some drugs also results in BP which may due to the immune-modulatory effects of these drugs. ICBs will also give rise to disturbance of immune responses. The increased risk of BP in patients receiving anti-PD-1/PD-L1 has been confirmed by series of clinic researches (11, 13, 82, 83), while the molecular mechanisms still need further researches to elucidate. A depletion of Tregs, which function depending on immune checkpoints, may play a role in the pathogenesis of BP, according to immunopathological results (84).
The relevance of anti-tumor efficacy and the irAEs of ICIs also implies the autoimmune mechanism of irAEs. While some studies did not confirm the association between the treatment efficacy and irAEs (29), others did find that the occurrence of irAEs was usually associated with a more robust response to ICI therapy and better prognosis (70, 85–88). GWAS studies also identified an IL-7 variant that can lead to increased irAEs incidence and a concomitant increase in overall survival in melanoma patients (89, 90). The failure of the immune system to eliminate tumors is partially due to a lack of a true ‘onco-antigen’, that is, the tumors do not present distinct antigens that can be recognized by the immune system rather than being tolerated. This is because tumors comprise part of our body and the mechanism of immune tolerance can prevent the body’s response to them. The condition may differ with the application of ICIs, which interferes with immune homeostasis. Several studies have found that severe irAEs were associated with a longer overall survival, even while regarding irAEs as an indicator for predicting the prognosis of patients receiving ICIs. This finding may be partly explained by the fact that ICIs can promote the anti-tumor immune response by inhibiting immune tolerance, as PD-1/PD-L1 plays an important role in mediating the immune tolerance. An example is vitiligo, a common irAE in melanoma patients receiving anti-PD-1 therapy that is caused by an autoimmune attack to melanocytes (Figure 2). Research has proposed that this effect is due to the cross-reactivity between T cells directed against tumors and a related antigen expressed in normal tissues (27). Under normal physiological conditions, T cell targeting of antigens expressed on normal melanocytes will not be activated, nor will those that target related antigens expressed on tumors due to the immune tolerance. Therefore, neither the auto-immune response nor the anti-tumor response is intensely elicited. Once tolerance is destroyed by PD-1/PD-L1 blockade, these T cells will be activated by melanoma or by melanocytes, thus attacking melanoma cells as well as normal melanocytes, which may account for the relevance between the efficacy and irAEs of ICIs. Moreover, this theory may help explain why the irAEs vary among the different types of cancers, depending on the cross antigens between tumors and normal cells. Whether the PD-1/PD-L1 blockade could promote anti-tumors immunity via decreasing tolerance to tumors requires validation with further research. However, the role of checkpoints in preventing autoimmune diseases and the relationship between the severity of irAEs and better prognosis have been established by previous studies.
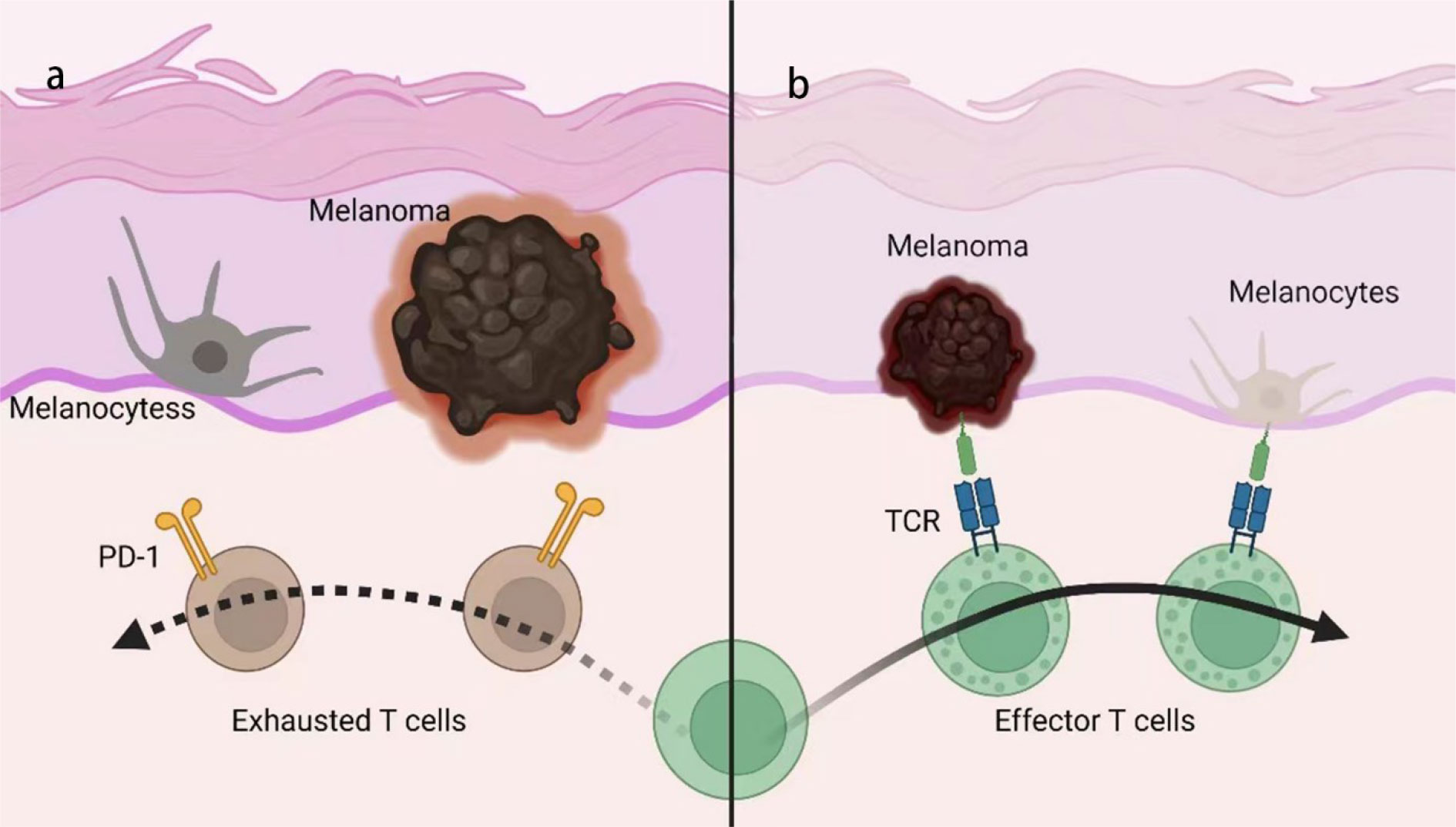
Figure 2 Mechanism of anti-PD-1/PD-L1-induced vitiligo. (A) Melanoma and melanocytes exhibit some shared antigen but are protected from immune attack by peripheral tolerance mechanisms, such as PD-1/PD-L1. (B) With PD-1/PD-L1 blocked, T cells will be activated by melanoma and initiate immune response targeting both tumors and normal tissue, due to the shared antigen on melanoma and melanocytes, termed ‘cross-activation’. TCR, T cell receptor.
3.2 Targeting at commensal microbiota
The link between commensal microbiota and response to ICIs for the treatment of cancer has been revealed by a series of studies that investigated the heterogeneity of the patients’ response to ICIs (91–98). Using 16S ribosome RNA gene sequencing, both preclinical and clinical studies have found distinct commensal microbiota between patients who are ICI-responsive and non-responsive (97, 99). There is increased concern that the commensal microbiota and its metabolites have a substantial influence on host homeostasis. For example, the interaction between the microbiota and host can ‘shape’ the immune system of the host. As a result, there is a heterogeneous response to ICIs due to the heterogeneity of the commensal microbiota to some extent. Although the association between the anti-tumor efficacy and the irAEs of ICIs remains conflicting (29, 85, 100–102), some studies have actually found a positive relationship (100–102), indicating that commensal microbiota may play a role in the pathogenesis of irAEs. It has been well established that the colon is the location in which there is the most abundant commensal microbiota, and the skin is another important residence. Epidemiological investigations have shown that irAEs most commonly involve the skin, GI tract, and endocrine system (5, 103). The microbiota abundance and irAE frequency in the skin and GI tract may indicate the potential relevance of commensal microbiota. One study confirmed an association between irAEs and several Lachnospiraceae spp. and indicated that the abundance of Streptococcus spp. substantially contributes to the distinction of irAEs (91). Additional studies have also supported the association between the commensal microbiota and irAEs (93, 95, 104). Reports researching the impact of PPIs have found that the application of PPIs has also had an impact on irAEs by influencing the microbiome (105, 106). The commensal microbiota profile may be used to predict irAEs (93) and the therapy targeting the commensal microbiota, such as fecal microbiota transplantation (FMT), may be used to cure irAEs (107).
Skin is the first-line barrier to protect the host from microbial invasion while maintaining a peaceful coexistence with resident microbiota (108), along with other barriers, such as the GI tract, respiratory tract and genital tract. A homeostatic state is formed under the complex microbiota-host interaction network and the intensity of immune response is controlled to a ‘set point’, which is suitable for the micro-ecosystem. Immune homeostasis is maintained by many immune regulators, including cytokines, regulatory receptors and regulatory immune cells. The application of ICIs makes great intervention for this control mechanism. Physiologically, within the action of checkpoints, T cells will not become activated to target commensal microbiota due to the lack of activation signals and presence of inhibition signals, plus some regulatory immune cells (e.g., Tregs) and cytokines (e.g., IL-10). Once CTLA-4 or PD-1/PD-L1 is blocked, however, CD4+ and CD8+ T cells may be activated and induce a subsequent immune response, which can lead to tissue damage. A recent study found that Staphylococcus epidermidis could only elicit inflammation in the context of a CTLA-4 blockade, the latter of which resulted in excessive activation of IL-17-producing commensal-specific T cells; thus inducing skin damage (109). Moreover, since the commensal microbiota itself also plays a crucial role in maintaining immunological homeostasis, an inappropriate immune response causes indirect damage by inhibiting commensal microbiota. Research into inflammatory bowel disease (IBD) has revealed that the failure to limit inappropriate inflammation contributes to ulcerative colitis and Crohn’s disease (110–112), and other research about atopic dermatitis (AD) has found that microbiota diversity was decreased in inflamed AD skin (113) and reverted during the treatment and recovery (114). Moreover, epidermal barrier dysfunction represents a key factor associated with the pathogenesis of AD, which can be due to an over-release of pro-inflammatory cytokines and damage-associated molecular patterns (DAMPs) (115), such as that seen in inherited filaggrin deficiency (116). Further research into the influence of ICIs on the cutaneous micro-economy must be conducted. In summary, the cutaneous commensal microbiota is highly involved in the pathogenesis of cirAEs and the immune responses targeting commensal microbiota can lead to tissue damage by both direct and indirect methods, as a result of impaired immune tolerance.
3.3 The cutaneous immunity
In this section, we will aim to explore that why the skin is a common and early target of irAEs. The hallmark of the skin from an immunological perspective is the abundance of immune molecules and cells, as well as higher activity of the immune response. The immune profile is determined by and is suitable for the systemic role of the skin as a barrier for the entire body and its corresponding physiological functions. Our internal environment is separated from the external environment by the skin and mucosa, which covers the surface of body and the internal lumen, respectively, such that the skin is exposed to the most direct effects of various of physical, chemical and biological factors. The role of the skin to protect the internal environment from being affected by these disturbing agents, thereby helping to maintain internal homeostasis, so as to increase the active immunity of the skin. Similar to the colon, which also continuously makes contact with a wide range of foreign antigens and may lead to diarrhea, abdominal distension, and abdominal pain if the local immune response is induced (117–120), the skin also continuously faces a multitude of foreign stimuli. Moreover, since the immune responses to these factors can occasionally be senseless and challenging, immune tolerance is of greater significance in the skin compared to other parts of the body. UV radiation will give rise to DNA damage (121, 122), contacting chemicals will also affect, and the commensal microbiota is an obvious source of foreign antigens. Using the immune response induced by UV radiation as an example, keratinocytes could be activated by UV radiation, initiating the formation of NLRP3 inflammasome and releasing IL-1β, which acts as an important pro-inflammatory cytokine (123, 124). By blocking the mechanisms used to limit the immune response, the persistent stimuli of UV radiation can over-activate the immune response in both intensity and duration, leading to cutaneous disorders. Therefore, the skin is a common target for the destruction of immune tolerance by ICIs. Moreover, the immune response in the skin is more readily initiated due to the abundance of the immune cells residing within the skin. Keratinocytes and melanocytes represent two major types of epidermal cells which generate keratin and melanin and act as crucial components of the skin barrier, respectively. In addition, both of these cell types express TLRs and NLRs and can initiate the immune response by secreting pro-inflammatory cytokines and chemokines to activate and recruit other immune cells (125, 126). Another important cell type that can induce an immune response is Langerhans cells (LCs), which can be regarded as a specific type of dendritic cell for its similar antigen-presenting function as a classic DC, whereas recent studies have shown that LCs are resident macrophages in the epidermis (127). Additionally, tissue-resident memory T cells (TRM) also contribute substantially with their ability to rapidly recall the immune response by releasing cytokines or exhibiting cytotoxicity (128). In particular, CD8+ TRM have been shown to patrol the tissue or function as a local sentinel in both epidermal and dermal layers, providing a rapid and tissue-wide immune response (129). Significantly, there is a constitutive expression of negative checkpoints (e.g., PD-1, LAG-3, and Tim-3) on these TRM in the skin (130, 131). Therefore, the application of ICIs may lead to the over-activation of TRM. Taken together, these characteristics of cutaneous immunity indicate that the skin is more susceptible to the adverse events induced by drugs that affect immune homeostasis and the onset of cirAEs occurs swiftly due to the rapid responses of these immune cells.
In addition to inducing a local immune response, the skin is also responsible for transmitting invading signals to the brain; thus, the skin is largely innervated by sensory nerves. The role of inflammation on inducing sensations such as pain and itching is relatively clear (132). It has been shown that some neuropeptides, such as substance P (SP) and vasoactive intestinal peptide (VIP), can activate immune cells (e.g., mast cells), as confirmed by previous studies (133–135). Additionally, several studies have reported highly complex crosstalk or interactions between the immune response and these sensations. Moreover, the experimental application of imiquimod (IMQ) in murine skin could provoke inflammatory lesions that resemble human psoriasis. This effect was found to be blocked pharmacologically or through genetic ablation of nociceptors and could be restored by exogenous IL-23. A subsequent detailed study confirmed that the sensory neurons expressing the ion channels, TRPV1 and NaV1.8, could regulate the production of IL-23/IL-17 by interacting with dermal dendritic cells to modulate the local immune response (136). Another subset of neurons expressing MrgprD was shown to inhibit the degranulation of mast cells and limit the cutaneous immune response via releasing glutamate. Indeed, the loss of these neurons may lead to immune disorders (137). There is also a subset of macrophages that have been identified to interact with sensory nerves, surveilling and trimming the myelin sheath (138). Due to the complicated interaction between sensory nerves and immune cells, such homeostasis appears to be susceptible to intervention, and some unpleasant sensations (e.g., chronic pain and itchiness) are commonly induced once the immune response is activated, as the manifestation of cirAEs.
3.4 Genetics
We have established that the irAEs are closely related to autoimmunity. Despite the unclear precise mechanism, epidemiological investigations have found that autoimmune diseases usually have a strong genetic component, which means some are easier to suffer from these diseases, whereas others are not. Psoriasis is a common disease that cirAEs appear to mimic (also termed ‘psoriasiform rash’) after using PD-1/PD-L1 (139, 140) and is considered to be an autoimmune disease to some extent (141, 142). Previous research has found that in people with parents suffering from psoriasis are easier to suffer from this disease (143), several psoriasis susceptibility genes have been identified, including HLA-Cw6, IL12B, IL23R, and LCE3B/3C (144). T1DM is another example which is also a classic autoimmune disease and one of the most common irAEs. The primary risk factor for β-cell immunity is confirmed as genetic, which mainly occurs in individuals with either HLA-DR3-DQ2 or HLA-DR4-DQ8 haplotypes, or both (145). Alopecia is the most common hair toxicity associated with ICIs and has a phenotype similar to alopecia areata (AA) (13). The GWAS study identified 139 SNPs associated with AA and demonstrated an autoimmune mechanism (146). Genomics-based and recent multi-omics-based approaches have shed light on the research into autoimmune diseases and irAEs. Some of these biomarkers may potentially be applied for the prediction and precise treatment of diseases in the future.
4 Prediction of cirAEs
Since cutaneous irAEs occur often and early, patients suffer and are forced to withdraw, and there is an urgent and unmet demand for seeking validated biomarkers to predict cirAEs due to its high morbidity and negative influence on cancer immunotherapy. This task can be conducted by traditional epidemiological methods, such as cross-sectional, cohort, or case-control studies (147–149). The probable biomarkers that have currently been identified are either general characteristics (e.g., age (150, 151), gender (152), and BMI (153)) or common serum molecules based on the current understanding of the immunological mechanisms of cirAEs (e.g., CRP (154), IFN-γ (155)). Regarding to the antigenicity, tumor mutation burden (TMB) (156–158) and microsatellite instability (MSI) (159, 160), which stand for the neoantigen or onco-antigen, are used to predict the efficacy of ICIs and the risk of irAEs. As for the strategies by which tumors suppress the immune response, the expression of PD-L1 was also considered as a predictive biomarker for the responsiveness of ICIs (161). However, these biomarkers do not provide appropriate predictions in clinical practice, and the results of studies identifying these biomarkers also remain conflicting. These discrepancies are partially due to the heterogeneity of the cirAEs. As previously discussed, cirAEs are closely related to cutaneous commensal microbiota and autoimmunity, both of which are highly heterogeneous among the population. Therefore, general characteristics alone may not be sufficient to induce cirAEs and those serum factors often play a common role in several pathways in the immune network, thereby lacking precision. Traditional research methods of molecular biology explore the role of a ‘pathway’ rather than dealing with the ‘network’, ignoring the crosstalk among the pathways which appear to be independent. In contrast, the technology of multi-omics screens for the whole genome, transcriptome, proteome and metabolome, analyzing the whole signaling network in different stages from gene to metabolites. It also helps to study commensal microbiota, which is unavoidable in cirAE research, as discussed previously.
Researchers have identified LCP1 and ADPGK as irAE biomarkers by conducting a comprehensive screening across mRNA, miRNA, lncRNA, protein expression and non-silent gene mutations across 26 cancer types, in which lymphocyte cytosolic protein 1 (LCP1), involved in T cell activation, achieved the highest correlation coefficient. The addition of the adenosine diphosphate-dependent glucokinase (ADPGK), which can mediate a metabolic shift during T cell activation, to LCP1, led to a linear-regression model with the best accuracy among all the bivariate models. These two biomarkers were validated by a subsequent cohort study, which involved 28 cancer patients receiving anti-PD-1/PD-L1 therapy and found higher geometric mean and stronger immunohistochemistry staining in the irAEs group. The area under the receiver-operating characteristic curve (AUC) of LCP1 and ADPGK to predict irAE was 0.78 and 0.78, whereas the combination of LCP1 and ADPGK had a better AUC value at 0.80 (162). Another study established a tri-variate model composed of CDC42EP3-206, TMEM138-211, and IRX3-202 to predict irAEs by combining pharmacovigilance data and pan-cancer transcriptomic information (163). RNA and whole exon sequencing of tumors from 13 patients who developed ICI-induced diabetes mellitus (ICI-DM) showed significant overexpression of ORM1, PLG, G6PC and a missense mutation in NLRC5. The researcher proposed that NLRC5 mutation could be used to predict ICI-DM (164). The analysis of protein-protein interactions also helped to identify biomarkers, for which four immunogenetic variants were identified by genotyping 39 variants in 18 genes using a multiplex genotyping assay (165). Single-cells RNA sequencing revealed that patients with distinct T cells populations at baseline were under the risk of distinct types of irAEs and this could serve as biomarker for those irAEs. Fewer CD8+ TCM cells, more Th2 and Th17 cells were observed in patients with irAEs, and were associated with a higher risk of ICIs-induced arthritis, pneumonitis, and thyroiditis (166). Higher resolution human leucocyte antigen (HLA)-I typing on 179 patients with NSCLC treated with anti-PD-1/PD-L1 found that homozygosity at one or more HLA-I loci was associated with a reduced risk of irAE, including pruritus and rash (relative risk (RR) = 0.61, 95% CI 0.33 − 0.95, P = 0.035) and this could also serve as a biomarker (167). A variation in HLA-DRB1 was found to be associated with one or more types of cirAEs, and a more detailed association between HLA-DRB1*11:01 and pruritus was validated (168). This finding was in line with that of a previous study confirming an association between HLA-DRB1*11:01 and atopic dermatitis (AD) (169). The results of 16S rRNA gene amplification and multi-parallel sequencing also indicated that microbiota may serve as a potential biomarker (93, 104). Non-coding RNA (e.g., miR-146a) was found to be associated with irAEs in preclinical studies, and the predictive efficacy was validated by analyzing the effect of a SNP in the MIR164A gene on irAEs in 167 patients treated with ICIs. SNP rs2910164 led to reduced miR-164a expression and was associated with an increased risk of irAEs (170). Taken together, these studies via multi-omics technology have shed light on the discovery of biomarkers that can predict irAEs, although the efficacy should be validated using large number of clinical trials and the testing methods must be improved to be suitable for clinical practice.
There’re also many biomarkers for the responsiveness to ICIs therapy being identified by means of multi-omics, with a potential role in predicting irAEs as well. In a rich resource of scRNA-seq and bulk mRNA-seq analysis, B cells and T follicular helper cells were found mediating the response to ICI in breast cancers, and a new predictive gene signature was identified (171). Based on the antigenicity of tumors, the analysis of self-immunopeptidome also served to predict the response of ICIs. This method calculated the ratio of nonsynonymous to synonymous mutation (dN/dS) to discriminate the ‘escaped tumors’ and ‘edited tumors’, with the former presenting neoantigens but escaping immune attack by immunosuppressive mechanisms such as over-expressing PD-L1, thus responsive to ICIs and under risk of irAEs; and the latter escaping by neoantigen-depletion that prevent tumors from being recognized by immune system, thus non-responsive to ICIs (172). A study of proteomics also identified leukemia inhibitory factor (LIF) as a novel predictive biomarker of resistance of ICIs (173). Moreover, a quantitative functional proteomics analysis[QF-Pro] found that functional engagement of the PD-1/PD-L1 complex but not PD-L1 expression alone is highly predictive to the response to ICIs in non-small-cell lung cancer (174). Another multi-omics study of 108 human papilloma virus (HPV)-negative head and neck squamous cell carcinomas (HNSCCs) identified three subtypes with responsiveness to CDK inhibitors, anti-EGFR antibody and immunotherapy, respectively, and an immune-proteogenomic analysis uncovered the mechanisms of immunosuppression and ICIs-resistance (175). Metabolomics studies found that hypoxanthine and histidine in early on-treatment serum (176), indoleamine-2,3-dioxygenase (IDO) (177) and very long-chain fatty acid-containing lipids (VLCFA-containing lipids) (178) were also predictive biomarkers for the response to ICIs. Still, these biomarkers need further validation for the predictive efficacy of irAEs, in addition to the anti-tumor efficacy.
Another important method is radiomics, which assess tumors based on the abundant images from computed tomography (CT), magnetic resonance imaging (MRI) and positron emission tomography/computed tomography (PET/CT) (173, 174). The analysis of genomics, transcriptomics, proteomics and metabolomics, as previously discussed, need puncture or surgery for biopsy. Due to the spatial and temporal heterogeneity, however, it is not available to get whole information by sampling (175). As a widely used and non-invasive diagnosis method, radiographic examination serves as a repeatable and rapid approach for assessing the tumors. These traditional radiographic imaging, equipped with modern technologies such as high-resolution imaging, data mining algorithms and high-throughput analysis, provides global information about the biology of tumors and contributes to predicting the efficacy and adverse events of immunotherapy. Nowadays, radiomics studies seek for biomarkers mainly by analyzing the characteristics of tumor microenvironment (TME) (176), such as tumor-infiltrating lymphocytes (TIL), microcirculation, various signal molecules and extracellular matrix, tightly associated with immunotherapy. As known, TIL is a significant parameter to predict the response to immunotherapy, CT, PET/CT and MRI-based biomarkers to assess TIL have been identified by radiomics studies (4, 177, 178). There’re also radiomics-based prediction models for assessing the PD-L1 expression being proposed, using the radiomics feature combined with clinical characteristics (5–9). Radiomics features that predict ICI-induced pneumonitis were identified by maximum relevance and minimum redundancy feature selection method, anomaly detection algorithm, and leave-one-out cross-validation, despite the predictive efficacy to other types of irAEs remaining unvalidated. Nevertheless, radiomics analysis, involving in the technology of multi-omics, will play an increasingly crucial role in the research of tumors.
5 Summary
In this review we discussed the application of ICI therapy to cancers and its adverse events in epidemiology, followed by a detailed discussion of its immunological mechanism and prediction. Cutaneous irAEs represents one of the most common types of irAE associated with ICI therapy and can lead to substantial suffering, as well as hinder the normal application of ICI, with a blockade of the normal role of checkpoints in the regulation of immune response being an initiating factor of irAEs. The immune response is a double-edge sword in that an appropriate immune response can serve as a defense against invading pathogenic microbes, eliminating damaged and aging cells and surveilling the oncogenic cells, whereas a disordered immune response will induce harmful effects. Checkpoints, including positive and negative checkpoints, play a crucial role in limiting the immune response and mediating immune tolerance. In this manner, these checkpoints contribute substantially to the maintenance of immune homeostasis, although it might be used by tumors to suppress immunity. From this perspective, ICIs may induce the non-specific enhancement of immune activity, including both increased cytotoxicity and broadened immune targets, thereby giving rise to an over-activated immune response. Such overactivation can manifest as irAE, and cutaneous irAEs commonly occur early for the distinctive immune signature of the skin as discussed above. For the broad use of ICIs in comprehensive cancer therapy, predicting and identifying irAEs is necessary, especially cirAEs. Previous studies have primarily conducted epidemiological investigations or measured the serum levels of some immune molecules, failing to identify satisfying biomarkers due to the heterogeneity of irAEs. Multi-omics analysis has shed light on the precise mechanism of irAEs and identify several genetic variants, non-coding RNAs and enzymes, which can potentially serve as biomarkers for predicting cirAEs; however, further clinical validation is required. Nevertheless, we believe that multi-omics research will continue to contribute more for both uncovering the mechanism and identifying of biomarkers.
Although various of PD-1/PD-L1 blockers have been applicated in clinical practice, the complete mechanisms of checkpoints such as TIM-3 and LAG-3 still remain unclear and the distinct mechanisms of irAEs induced by different types of ICBs need more researches to elucidate. The clinical manifestations of irAEs also provide a novel pathway to uncover the role of these immune checkpoints in regulating immune homeostasis. More precise understanding of cutaneous immunity and cutaneous immune diseases such as psoriasis and atopic dermatitis is also needed for exploring the mechanisms of cirAEs. The predictive biomarkers for cirAEs will be more precise, specific to certain type and severity of cirAEs. Finally, the developing multi-omics analysis technologies, especially single-cell and spatial multi-omics analysis, will provide more and more information which helps not only to find predictive biomarkers but also to uncover the mechanisms of cirAEs.
Author contributions
TC conducted the bulk of the writing and the crafting of the figures; XZ performed the formulation of the overarching research goal and the revision of the draft; XZ and XW retrieved literature; YZ critically revised the manuscript. All authors contributed to the article and approved the submitted version.
Funding
This study was financially funded by the National Natural Science Foundation of China (NO. 81602757) and Technology Commission of Shanghai Municipality (No. 21Y11905200).
Acknowledgments
Figures were created with biorender.com.
Conflict of interest
The authors declare that the research was conducted in the absence of any commercial or financial relationships that could be construed as a potential conflict of interest.
Publisher’s note
All claims expressed in this article are solely those of the authors and do not necessarily represent those of their affiliated organizations, or those of the publisher, the editors and the reviewers. Any product that may be evaluated in this article, or claim that may be made by its manufacturer, is not guaranteed or endorsed by the publisher.
References
1. Meissner TB, Schulze HS, Dale SM. Immune editing: overcoming immune barriers in stem cell transplantation. Curr Stem Cell Rep (2022) 8(4):206–18. doi: 10.1007/s40778-022-00221-0
2. Starling S. Mhc molecules: immune editing shapes the cancer landscape. Nat Rev Immunol (2017) 17(12):729. doi: 10.1038/nri.2017.129
3. Sundar R, Huang KK, Kumar V, Ramnarayanan K, Demircioglu D, Her Z, et al. Epigenetic promoter alterations in gi tumour immune-editing and resistance to immune checkpoint inhibition. Gut (2022) 71(7):1277–88. doi: 10.1136/gutjnl-2021-324420
4. Boutros C, Tarhini A, Routier E, Lambotte O, Ladurie F, Carbonnel F, et al. Safety profiles of anti-Ctla-4 and anti-Pd-1 antibodies alone and in combination. Nat Rev Clin Oncol (2016) 13(8):473–86. doi: 10.1038/nrclinonc.2016.58
5. Sibaud V. Dermatologic reactions to immune checkpoint inhibitors : skin toxicities and immunotherapy. Am J Clin Dermatol (2018) 19(3):345–61. doi: 10.1007/s40257-017-0336-3
6. Quach H, Johnson D, LeBoeuf N, Zwerner J, Dewan A. Cutaneous adverse events caused by immune checkpoint inhibitors. J Am Acad Dermatol (2021) 85(4):956–66. doi: 10.1016/j.jaad.2020.09.054
7. Capdevila J, Wirth L, Ernst T, Ponce Aix S, Lin C, Ramlau R, et al. Pd-1 blockade in anaplastic thyroid carcinoma. J Clin Oncol Off J Am Soc Clin Oncol (2020) 38(23):2620–7. doi: 10.1200/JCO.19.02727
8. Schoenfeld J, Giobbie-Hurder A, Ranasinghe S, Kao K, Lako A, Tsuji J, et al. Durvalumab plus tremelimumab alone or in combination with low-dose or hypofractionated radiotherapy in metastatic non-Small-Cell lung cancer refractory to previous Pd(L)-1 therapy: an open-label, multicentre, randomised, phase 2 trial. Lancet Oncol (2022) 23(2):279–91. doi: 10.1016/s1470-2045(21)00658-6
9. Ueno M, Ikeda M, Morizane C, Kobayashi S, Ohno I, Kondo S, et al. Nivolumab alone or in combination with cisplatin plus gemcitabine in Japanese patients with unresectable or recurrent biliary tract cancer: a non-randomised, multicentre, open-label, phase 1 study. Lancet Gastroenterol Hepatol (2019) 4(8):611–21. doi: 10.1016/s2468-1253(19)30086-x
10. Asdourian MS, Shah N, Jacoby TV, Reynolds KL, Chen ST. Association of bullous pemphigoid with immune checkpoint inhibitor therapy in patients with cancer: a systematic review. JAMA Dermatol (2022) 158(8):933–41. doi: 10.1001/jamadermatol.2022.1624
11. Shalata W, Weissmann S, Itzhaki Gabay S, Sheva K, Abu Saleh O, Jama A, et al. A retrospective, single-institution experience of bullous pemphigoid as an adverse effect of immune checkpoint inhibitors. Cancers (Basel) (2022) 14(21):5451. doi: 10.3390/cancers14215451
12. Kawsar A, Edwards C, Patel P, Heywood RM, Gupta A, Mann J, et al. Checkpoint inhibitor-associated bullous cutaneous immune-related adverse events: a multicentre observational study. Br J Dermatol (2022) 187(6):981–7. doi: 10.1111/bjd.21836
13. Geisler AN, Phillips GS, Barrios DM, Wu J, Leung DYM, Moy AP, et al. Immune checkpoint inhibitor-related dermatologic adverse events. J Am Acad Dermatol (2020) 83(5):1255–68. doi: 10.1016/j.jaad.2020.03.132
14. Khoja L, Day D, Wei-Wu Chen T, Siu L, Hansen A. Tumour- and class-specific patterns of immune-related adverse events of immune checkpoint inhibitors: a systematic review. Ann Oncol Off J Eur Soc Med Oncol (2017) 28(10):2377–85. doi: 10.1093/annonc/mdx286
15. Cutroneo P, Ingrasciotta Y, Isgrò V, Rullo E, Berretta M, Fiorica F, et al. Psoriasis and psoriasiform reactions secondary to immune checkpoint inhibitors. Dermatol Ther (2021) 34(2):e14830. doi: 10.1111/dth.14830
16. Chan L, Hwang S, Byth K, Kyaw M, Carlino M, Chou S, et al. Survival and prognosis of individuals receiving programmed cell death 1 inhibitor with and without immunologic cutaneous adverse events. J Am Acad Dermatol (2020) 82(2):311–6. doi: 10.1016/j.jaad.2019.06.035
17. Lee Y, Kim H, Won C, Chang S, Lee M, Choi J, et al. Characterization and prognostic significance of cutaneous adverse events to anti-programmed cell death-1 therapy. J Korean Med Sci (2019) 34(26):e186. doi: 10.3346/jkms.2019.34.e186
18. Zhang J, Zhang P, Xu Q, Zhu Y, Chen W, Ji C. Pembrolizumab associated stevens-Johnson syndrome with porokeratosis in a patient in the setting of primary hepatocellular carcinoma. Australas J Dermatol (2022) 63(1):e71–e4. doi: 10.1111/ajd.13704
19. Gong Y, Mao J, Liu M, Gao J. A case of toxic epidermal necrolysis associated with lenvatinib and sintilimab therapy for intrahepatic cholangiocarcinoma. J Int Med Res (2023) 51(5):3000605231173556. doi: 10.1177/03000605231173556
20. Yang H, Ma Q, Sun Y, Zhang K, Xing Y, Li H. Case report: toxic epidermal necrolysis associated with sintilimab in a patient with relapsed thymic carcinoma. Front Oncol (2022) 12:1065137. doi: 10.3389/fonc.2022.1065137
21. Criado PR, Avancini J, Santi CG, Medrado AT, Rodrigues CE, de Carvalho JF. Drug reaction with eosinophilia and systemic symptoms (Dress): a complex interaction of drugs, viruses and the immune system. Isr Med Assoc J (2012) 14(9):577–82.
22. Tivol EA, Borriello F, Schweitzer AN, Lynch WP, Bluestone JA, Sharpe AH. Loss of ctla-4 leads to massive lymphoproliferation and fatal multiorgan tissue destruction, revealing a critical negative regulatory role of ctla-4. Immunity (1995) 3(5):541–7. doi: 10.1016/1074-7613(95)90125-6
23. Waterhouse P, Penninger JM, Timms E, Wakeham A, Shahinian A, Lee KP, et al. Lymphoproliferative disorders with early lethality in mice deficient in ctla-4. Science (1995) 270(5238):985–8. doi: 10.1126/science.270.5238.985
24. Esfahani K, Miller WH Jr. Reversal of autoimmune toxicity and loss of tumor response by interleukin-17 blockade. N Engl J Med (2017) 376(20):1989–91. doi: 10.1056/NEJMc1703047
25. Tarhini AA, Zahoor H, Lin Y, Malhotra U, Sander C, Butterfield LH, et al. Baseline circulating il-17 predicts toxicity while tgf-B1 and il-10 are prognostic of relapse in ipilimumab neoadjuvant therapy of melanoma. J Immunother Cancer (2015) 3:39. doi: 10.1186/s40425-015-0081-1
26. Lo JA, Fisher DE, Flaherty KT. Prognostic significance of cutaneous adverse events associated with pembrolizumab therapy. JAMA Oncol (2015) 1(9):1340–1. doi: 10.1001/jamaoncol.2015.2274
27. Byrne EH, Fisher DE. Immune and molecular correlates in melanoma treated with immune checkpoint blockade. Cancer (2017) 123(S11):2143–53. doi: 10.1002/cncr.30444
28. Brahmer JR, Lacchetti C, Schneider BJ, Atkins MB, Brassil KJ, Caterino JM, et al. Management of immune-related adverse events in patients treated with immune checkpoint inhibitor therapy: American society of clinical oncology clinical practice guideline. J Clin Oncol (2018) 36(17):1714–68. doi: 10.1200/jco.2017.77.6385
29. Horvat TZ, Adel NG, Dang TO, Momtaz P, Postow MA, Callahan MK, et al. Immune-related adverse events, need for systemic immunosuppression, and effects on survival and time to treatment failure in patients with melanoma treated with ipilimumab at memorial Sloan Kettering cancer center. J Clin Oncol (2015) 33(28):3193–8. doi: 10.1200/jco.2015.60.8448
30. Carli L, Tani C, Querci F, Della Rossa A, Vagnani S, Baldini C, et al. Analysis of the prevalence of cataracts and glaucoma in systemic lupus erythematosus and evaluation of the rheumatologists' practice for the monitoring of glucocorticoid eye toxicity. Clin Rheumatol (2013) 32(7):1071–3. doi: 10.1007/s10067-013-2214-6
31. Huscher D, Thiele K, Gromnica-Ihle E, Hein G, Demary W, Dreher R, et al. Dose-related patterns of glucocorticoid-induced side effects. Ann Rheum Dis (2009) 68(7):1119–24. doi: 10.1136/ard.2008.092163
32. Wang Y, Zou J, Li Y, Jiao X, Wang Y, Zhuo N, et al. Serological biomarkers predict immune-related adverse events and clinical benefit in patients with advanced gastrointestinal cancers. Front Immunol (2022) 13:987568. doi: 10.3389/fimmu.2022.987568
33. Shi Y, Liu X, Liu J, Zhang D, Liu X, Yue Y, et al. Correlations between peripheral blood biomarkers and clinical outcomes in advanced non-small cell lung cancer patients who received immunotherapy-based treatments. Trans Lung Cancer Res (2021) 10(12):4477–93. doi: 10.21037/tlcr-21-710
34. Muir C, Wood C, Clifton-Bligh R, Long G, Scolyer R, Carlino M, et al. Association of antithyroid antibodies in checkpoint inhibitor-associated thyroid immune-related adverse events. J Clin Endocrinol Metab (2022) 107(5):e1843–e9. doi: 10.1210/clinem/dgac059
35. Johnson D, Patel AB, Uemura MI, Trinh VA, Jackson N, Zobniw CM, et al. Il17a blockade successfully treated psoriasiform dermatologic toxicity from immunotherapy. Cancer Immunol Res (2019) 7(6):860–5. doi: 10.1158/2326-6066.Cir-18-0682
36. Koguchi Y, Iwamoto N, Shimada T, Chang S, Cha J, Curti B, et al. Trough levels of ipilimumab in serum as a potential biomarker of clinical outcomes for patients with advanced melanoma after treatment with ipilimumab. J Immunother Cancer (2021) 9(10):e002663. doi: 10.1136/jitc-2021-002663
37. Kankkunen E, Penttilä P, Peltola K, Bono P. C-reactive protein and immune-related adverse events as prognostic biomarkers in immune checkpoint inhibitor treated metastatic renal cell carcinoma patients. Acta Oncol (Stockholm Sweden) (2022) 61(10):1240–7. doi: 10.1080/0284186X.2022.2104132
38. Zhou J, Wong A, Wang H, Tan F, Chen X, Jin S, et al. Elucidation of the application of blood test biomarkers to predict immune-related adverse events in atezolizumab-treated nsclc patients using machine learning methods. Front Immunol (2022) 13:862752. doi: 10.3389/fimmu.2022.862752
39. Tahir SA, Gao J, Miura Y, Blando J, Tidwell RSS, Zhao H, et al. Autoimmune antibodies correlate with immune checkpoint therapy-induced toxicities. Proc Natl Acad Sci U.S.A. (2019) 116(44):22246–51. doi: 10.1073/pnas.1908079116
40. Toi Y, Sugawara S, Sugisaka J, Ono H, Kawashima Y, Aiba T, et al. Profiling preexisting antibodies in patients treated with anti-Pd-1 therapy for advanced non-small cell lung cancer. JAMA Oncol (2019) 5(3):376–83. doi: 10.1001/jamaoncol.2018.5860
41. Gay S, Rossi G, Corica G, Graziani G, Genova C, Rijavec E, et al. Retracted article: can baseline endocrinological examination and thyroid ultrasound predict the development of thyroid disease in immunotherapy-treated patients? results from a prospective, single-center, open-label study. Endocrine (2020) 69(1):233. doi: 10.1007/s12020-019-01854-8
42. Möhn N, Mahjoub S, Duzzi L, Narten E, Grote-Levi L, Körner G, et al. Monocyte chemoattractant protein 1 as a potential biomarker for immune checkpoint inhibitor-associated neurotoxicity. Cancer Med (2023) 12(8):9373–83. doi: 10.1002/cam4.5695
43. Eun Y, Kim IY, Sun JM, Lee J, Cha HS, Koh EM, et al. Risk factors for immune-related adverse events associated with anti-Pd-1 pembrolizumab. Sci Rep (2019) 9(1):14039. doi: 10.1038/s41598-019-50574-6
44. Pavan A, Calvetti L, Dal Maso A, Attili I, Del Bianco P, Pasello G, et al. Peripheral blood markers identify risk of immune-related toxicity in advanced non-small cell lung cancer treated with immune-checkpoint inhibitors. Oncologist (2019) 24(8):1128–36. doi: 10.1634/theoncologist.2018-0563
45. Gazzano M, Parizot C, Psimaras D, Vozy A, Baron M, Abbar B, et al. Anti-Pd-1 immune-related adverse events are associated with high therapeutic antibody fixation on T cells. Front Immunol (2022) 13:1082084. doi: 10.3389/fimmu.2022.1082084
46. Stephens M, Asdourian M, Jacoby T, Shah N, Thompson L, Otto T, et al. Tumor-infiltrating lymphocytes as a predictive biomarker of cutaneous immune-related adverse events after immune checkpoint blockade in patients with advanced melanoma. J Am Acad Dermatol (2023) S0190-9622(23):00193–7. doi: 10.1016/j.jaad.2023.01.040
47. Sholl LM, Hirsch FR, Hwang D, Botling J, Lopez-Rios F, Bubendorf L, et al. The promises and challenges of tumor mutation burden as an immunotherapy biomarker: a perspective from the international association for the study of lung cancer pathology committee. J Thorac Oncol (2020) 15(9):1409–24. doi: 10.1016/j.jtho.2020.05.019
48. Ren S, Chen J, Xu X, Jiang T, Cheng Y, Chen G, et al. Camrelizumab plus carboplatin and paclitaxel as first-line treatment for advanced squamous nsclc (Camel-sq): a phase 3 trial. J Thorac Oncol Off Publ Int Assoc Study Lung Cancer (2022) 17(4):544–57. doi: 10.1016/j.jtho.2021.11.018
49. Jin Y, Chen D, Wang F, Yang C, Chen X, You J, et al. The predicting role of circulating tumor DNA landscape in gastric cancer patients treated with immune checkpoint inhibitors. Mol Cancer (2020) 19(1):154. doi: 10.1186/s12943-020-01274-7
50. Yoshimura K, Tamano Y, Nguyen Canh H, Zihan L, Le Thanh D, Sato Y, et al. A novel pathologic marker, indoleamine 2,3-dioxygenase 1, for the cholangiopathy of immune checkpoint inhibitors-induced immune mediated hepatotoxicity as adverse events and the prediction of additional ursodeoxycholic acid treatment. Med Mol Morphol (2023) 56(2):106–15. doi: 10.1007/s00795-022-00344-7
51. DePeaux K, Delgoffe GM. Metabolic barriers to cancer immunotherapy. Nat Rev Immunol (2021) 21(12):785–97. doi: 10.1038/s41577-021-00541-y
52. Pavlova NN, Thompson CB. The emerging hallmarks of cancer metabolism. Cell Metab (2016) 23(1):27–47. doi: 10.1016/j.cmet.2015.12.006
53. Hanahan D, Weinberg RA. Hallmarks of cancer: the next generation. Cell (2011) 144(5):646–74. doi: 10.1016/j.cell.2011.02.013
54. Muntyanu A, Netchiporouk E, Gerstein W, Gniadecki R, Litvinov I. Cutaneous immune-related adverse events (Iraes) to immune checkpoint inhibitors: a dermatology perspective on management [Formula: see text]. J cutaneous Med Surg (2021) 25(1):59–76. doi: 10.1177/1203475420943260
55. Nguyen N, Wan G, Ugwu-Dike P, Alexander N, Raval N, Zhang S, et al. Influence of melanoma type on incidence and downstream implications of cutaneous immune-related adverse events in the setting of immune checkpoint inhibitor therapy. J Am Acad Dermatol (2023) 88(6):1308–16. doi: 10.1016/j.jaad.2023.02.014
56. Puzanov I, Diab A, Abdallah K, Bingham CO 3rd, Brogdon C, Dadu R, et al. Managing toxicities associated with immune checkpoint inhibitors: consensus recommendations from the society for immunotherapy of cancer (Sitc) toxicity management working group. J Immunother Cancer (2017) 5(1):95. doi: 10.1186/s40425-017-0300-z
57. Lacouture ME, Wolchok JD, Yosipovitch G, Kähler KC, Busam KJ, Hauschild A. Ipilimumab in patients with cancer and the management of dermatologic adverse events. J Am Acad Dermatol (2014) 71(1):161–9. doi: 10.1016/j.jaad.2014.02.035
58. Chang LS, Barroso-Sousa R, Tolaney SM, Hodi FS, Kaiser UB, Min L. Endocrine toxicity of cancer immunotherapy targeting immune checkpoints. Endocr Rev (2019) 40(1):17–65. doi: 10.1210/er.2018-00006
59. Miyahara K, Noda T, Ito Y, Hidaka H, Fujimoto S, Takedomi H, et al. An investigation of nine patients with gastrointestinal immune-related adverse events caused by immune checkpoint inhibitors. Digestion (2020) 101(1):60–5. doi: 10.1159/000504647
60. Chambers C, Sullivan T, Allison J. Lymphoproliferation in ctla-4-Deficient mice is mediated by costimulation-dependent activation of Cd4+ T cells. Immunity (1997) 7(6):885–95. doi: 10.1016/s1074-7613(00)80406-9
61. Marangoni F, Zhakyp A, Corsini M, Geels S, Carrizosa E, Thelen M, et al. Expansion of tumor-associated treg cells upon disruption of a ctla-4-Dependent feedback loop. Cell (2021) 184(15):3998–4015.e19. doi: 10.1016/j.cell.2021.05.027
62. Sun C, Mezzadra R, Schumacher TN. Regulation and function of the pd-L1 checkpoint. Immunity (2018) 48(3):434–52. doi: 10.1016/j.immuni.2018.03.014
63. Latchman Y, Wood C, Chernova T, Chaudhary D, Borde M, Chernova I, et al. Pd-L2 is a second ligand for pd-1 and inhibits T cell activation. Nat Immunol (2001) 2(3):261–8. doi: 10.1038/85330
64. Chen L, Flies DB. Molecular mechanisms of T cell Co-stimulation and Co-inhibition. Nat Rev Immunol (2013) 13(4):227–42. doi: 10.1038/nri3405
65. Johnson DB, Balko JM, Compton ML, Chalkias S, Gorham J, Xu Y, et al. Fulminant myocarditis with combination immune checkpoint blockade. N Engl J Med (2016) 375(18):1749–55. doi: 10.1056/NEJMoa1609214
66. Rui J, Deng S, Arazi A, Perdigoto AL, Liu Z, Herold KC. B cells that resist immunological attack develop during progression of autoimmune diabetes in nod mice. Cell Metab (2017) 25(3):727–38. doi: 10.1016/j.cmet.2017.01.005
67. Osorio JC, Ni A, Chaft JE, Pollina R, Kasler MK, Stephens D, et al. Antibody-mediated thyroid dysfunction during T-cell checkpoint blockade in patients with non-Small-Cell lung cancer. Ann Oncol (2017) 28(3):583–9. doi: 10.1093/annonc/mdw640
68. Sears C, Peikert T, Possick J, Naidoo J, Nishino M, Patel S, et al. Knowledge gaps and research priorities in immune checkpoint inhibitor-related pneumonitis. an official American thoracic society research statement. Am J Respir Crit Care Med (2019) 200(6):e31–43. doi: 10.1164/rccm.201906-1202ST
69. Cappelli LC, Thomas MA, Bingham CO 3rd, Shah AA, Darrah E. Immune checkpoint inhibitor-induced inflammatory arthritis as a model of autoimmune arthritis. Immunol Rev (2020) 294(1):106–23. doi: 10.1111/imr.12832
70. Teulings HE, Limpens J, Jansen SN, Zwinderman AH, Reitsma JB, Spuls PI, et al. Vitiligo-like depigmentation in patients with stage iii-iv melanoma receiving immunotherapy and its association with survival: a systematic review and meta-analysis. J Clin Oncol (2015) 33(7):773–81. doi: 10.1200/jco.2014.57.4756
71. Belzer A, Mortlock RD, Pach J, Cohen JM, Leventhal JS. The effect of baseline eczema or psoriasis on the morphology of cutaneous immune-related adverse events due to immune checkpoint inhibitor therapy. J Am Acad Dermatol (2023) 88(5):1198–200. doi: 10.1016/j.jaad.2023.01.002
72. Farh KK, Marson A, Zhu J, Kleinewietfeld M, Housley WJ, Beik S, et al. Genetic and epigenetic fine mapping of causal autoimmune disease variants. Nature (2015) 518(7539):337–43. doi: 10.1038/nature13835
73. Parkes M, Cortes A, van Heel DA, Brown MA. Genetic insights into common pathways and complex relationships among immune-mediated diseases. Nat Rev Genet (2013) 14(9):661–73. doi: 10.1038/nrg3502
74. Hirahara K, Ghoreschi K, Yang XP, Takahashi H, Laurence A, Vahedi G, et al. Interleukin-27 priming of T cells controls il-17 production in trans Via induction of the ligand pd-L1. Immunity (2012) 36(6):1017–30. doi: 10.1016/j.immuni.2012.03.024
75. Fife B, Pauken K, Eagar T, Obu T, Wu J, Tang Q, et al. Interactions between pd-1 and pd-L1 promote tolerance by blocking the tcr-induced stop signal. Nat Immunol (2009) 10(11):1185–92. doi: 10.1038/ni.1790
76. Osum KC, Burrack AL, Martinov T, Sahli NL, Mitchell JS, Tucker CG, et al. Interferon-gamma drives programmed death-ligand 1 expression on islet B cells to limit T cell function during autoimmune diabetes. Sci Rep (2018) 8(1):8295. doi: 10.1038/s41598-018-26471-9
77. Axelrod ML, Meijers WC, Screever EM, Qin J, Carroll MG, Sun X, et al. T Cells specific for A-myosin drive immunotherapy-related myocarditis. Nature (2022) 611(7937):818–26. doi: 10.1038/s41586-022-05432-3
78. Hou M, Wei Y, Zhao Z, Han W, Zhou R, Zhou Y, et al. Immuno-engineered nanodecoys for the multi-target anti-inflammatory treatment of autoimmune diseases. Adv Mater (Deerfield Beach Fla) (2022) 34(12):e2108817. doi: 10.1002/adma.202108817
79. Zhang X, Kang Y, Wang J, Yan J, Chen Q, Cheng H, et al. Engineered pd-L1-Expressing platelets reverse new-onset type 1 diabetes. Adv Mater (Deerfield Beach Fla) (2020) 32(26):e1907692. doi: 10.1002/adma.201907692
80. Ogishi M, Yang R, Aytekin C, Langlais D, Bourgey M, Khan T, et al. Inherited pd-1 deficiency underlies tuberculosis and autoimmunity in a child. Nat Med (2021) 27(9):1646–54. doi: 10.1038/s41591-021-01388-5
81. Gauci ML, Laly P, Vidal-Trecan T, Baroudjian B, Gottlieb J, Madjlessi-Ezra N, et al. Autoimmune diabetes induced by pd-1 inhibitor-retrospective analysis and pathogenesis: a case report and literature review. Cancer Immunol Immunother (2017) 66(11):1399–410. doi: 10.1007/s00262-017-2033-8
82. Guan S, Zhang L, Zhang J, Song W, Zhong D. A case report of steroid-refractory bullous pemphigoid induced by immune checkpoint inhibitor therapy. Front Immunol (2022) 13:1068978. doi: 10.3389/fimmu.2022.1068978
83. Wang T, Shao Q, Xiao C, Liu L. Case report: bullous pemphigoid associated with sintilimab therapy for Pmmr/Mss colorectal cancer. Front Oncol (2023) 13:1124730. doi: 10.3389/fonc.2023.1124730
84. Bowman C, Delrieu O. Immunogenetics of drug-induced skin blistering disorders. part I: perspective. Pharmacogenomics (2009) 10(4):601–21. doi: 10.2217/pgs.09.11
85. Hua C, Boussemart L, Mateus C, Routier E, Boutros C, Cazenave H, et al. Association of vitiligo with tumor response in patients with metastatic melanoma treated with pembrolizumab. JAMA Dermatol (2016) 152(1):45–51. doi: 10.1001/jamadermatol.2015.2707
86. Downey SG, Klapper JA, Smith FO, Yang JC, Sherry RM, Royal RE, et al. Prognostic factors related to clinical response in patients with metastatic melanoma treated by ctl-associated antigen-4 blockade. Clin Cancer Res (2007) 13(22 Pt 1):6681–8. doi: 10.1158/1078-0432.Ccr-07-0187
87. Haratani K, Hayashi H, Chiba Y, Kudo K, Yonesaka K, Kato R, et al. Association of immune-related adverse events with nivolumab efficacy in non-Small-Cell lung cancer. JAMA Oncol (2018) 4(3):374–8. doi: 10.1001/jamaoncol.2017.2925
88. Sanlorenzo M, Vujic I, Daud A, Algazi A, Gubens M, Luna SA, et al. Pembrolizumab cutaneous adverse events and their association with disease progression. JAMA Dermatol (2015) 151(11):1206–12. doi: 10.1001/jamadermatol.2015.1916
89. Groha S, Alaiwi SA, Xu W, Naranbhai V, Nassar AH, Bakouny Z, et al. Germline variants associated with toxicity to immune checkpoint blockade. Nat Med (2022) 28(12):2584–91. doi: 10.1038/s41591-022-02094-6
90. Taylor CA, Watson RA, Tong O, Ye W, Nassiri I, Gilchrist JJ, et al. Il7 genetic variation and toxicity to immune checkpoint blockade in patients with melanoma. Nat Med (2022) 28(12):2592–600. doi: 10.1038/s41591-022-02095-5
91. McCulloch J, Davar D, Rodrigues R, Badger J, Fang J, Cole A, et al. Intestinal microbiota signatures of clinical response and immune-related adverse events in melanoma patients treated with anti-Pd-1. Nat Med (2022) 28(3):545–56. doi: 10.1038/s41591-022-01698-2
92. Simpson R, Shanahan E, Batten M, Reijers I, Read M, Silva I, et al. Diet-driven microbial ecology underpins associations between cancer immunotherapy outcomes and the gut microbiome. Nat Med (2022) 28(11):2344–52. doi: 10.1038/s41591-022-01965-2
93. Chaput N, Lepage P, Coutzac C, Soularue E, Le Roux K, Monot C, et al. Baseline gut microbiota predicts clinical response and colitis in metastatic melanoma patients treated with ipilimumab. Ann Oncol Off J Eur Soc Med Oncol (2017) 28(6):1368–79. doi: 10.1093/annonc/mdx108
94. Han K, Nam J, Xu J, Sun X, Huang X, Animasahun O, et al. Generation of systemic antitumour immunity Via the in situ modulation of the gut microbiome by an orally administered inulin gel. Nat Biomed Eng (2021) 5(11):1377–88. doi: 10.1038/s41551-021-00749-2
95. Andrews M, Duong C, Gopalakrishnan V, Iebba V, Chen W, Derosa L, et al. Gut microbiota signatures are associated with toxicity to combined ctla-4 and pd-1 blockade. Nat Med (2021) 27(8):1432–41. doi: 10.1038/s41591-021-01406-6
96. Routy B, Le Chatelier E, Derosa L, Duong CPM, Alou MT, Daillère R, et al. Gut microbiome influences efficacy of pd-1-Based immunotherapy against epithelial tumors. Science (2018) 359(6371):91–7. doi: 10.1126/science.aan3706
97. Matson V, Fessler J, Bao R, Chongsuwat T, Zha Y, Alegre ML, et al. The commensal microbiome is associated with anti-Pd-1 efficacy in metastatic melanoma patients. Science (2018) 359(6371):104–8. doi: 10.1126/science.aao3290
98. Gopalakrishnan V, Spencer CN, Nezi L, Reuben A, Andrews MC, Karpinets TV, et al. Gut microbiome modulates response to anti-Pd-1 immunotherapy in melanoma patients. Science (2018) 359(6371):97–103. doi: 10.1126/science.aan4236
99. Sivan A, Corrales L, Hubert N, Williams JB, Aquino-Michaels K, Earley ZM, et al. Commensal bifidobacterium promotes antitumor immunity and facilitates anti-Pd-L1 efficacy. Science (2015) 350(6264):1084–9. doi: 10.1126/science.aac4255
100. Das S, Johnson DB. Immune-related adverse events and anti-tumor efficacy of immune checkpoint inhibitors. J Immunother Cancer (2019) 7(1):306. doi: 10.1186/s40425-019-0805-8
101. Grangeon M, Tomasini P, Chaleat S, Jeanson A, Souquet-Bressand M, Khobta N, et al. Association between immune-related adverse events and efficacy of immune checkpoint inhibitors in non-Small-Cell lung cancer. Clin Lung Cancer (2019) 20(3):201–7. doi: 10.1016/j.cllc.2018.10.002
102. Judd J, Zibelman M, Handorf E, O'Neill J, Ramamurthy C, Bentota S, et al. Immune-related adverse events as a biomarker in non-melanoma patients treated with programmed cell death 1 inhibitors. Oncologist (2017) 22(10):1232–7. doi: 10.1634/theoncologist.2017-0133
103. Weber JS, Hodi FS, Wolchok JD, Topalian SL, Schadendorf D, Larkin J, et al. Safety profile of nivolumab monotherapy: a pooled analysis of patients with advanced melanoma. J Clin Oncol (2017) 35(7):785–92. doi: 10.1200/jco.2015.66.1389
104. Dubin K, Callahan MK, Ren B, Khanin R, Viale A, Ling L, et al. Intestinal microbiome analyses identify melanoma patients at risk for checkpoint-Blockade-Induced colitis. Nat Commun (2016) 7:10391. doi: 10.1038/ncomms10391
105. Horvath A, Rainer F, Bashir M, Leber B, Schmerboeck B, Klymiuk I, et al. Biomarkers for oralization during long-term proton pump inhibitor therapy predict survival in cirrhosis. Sci Rep (2019) 9(1):12000. doi: 10.1038/s41598-019-48352-5
106. Cortellini A, Tucci M, Adamo V, Stucci LS, Russo A, Tanda ET, et al. Integrated analysis of concomitant medications and oncological outcomes from pd-1/Pd-L1 checkpoint inhibitors in clinical practice. J Immunother Cancer (2020) 8(2):e001361. doi: 10.1136/jitc-2020-001361
107. Wang Y, Wiesnoski DH, Helmink BA, Gopalakrishnan V, Choi K, DuPont HL, et al. Fecal microbiota transplantation for refractory immune checkpoint inhibitor-associated colitis. Nat Med (2018) 24(12):1804–8. doi: 10.1038/s41591-018-0238-9
108. Belkaid Y, Tamoutounour S. The influence of skin microorganisms on cutaneous immunity. Nat Rev Immunol (2016) 16(6):353–66. doi: 10.1038/nri.2016.48
109. Ma B, Anandasabapathy N. Immune checkpoint blockade and skin toxicity pathogenesis. J Invest Dermatol (2022) 142(3 Pt B):951–9. doi: 10.1016/j.jid.2021.06.040
110. Kühn R, Löhler J, Rennick D, Rajewsky K, Müller W. Interleukin-10-Deficient mice develop chronic enterocolitis. Cell (1993) 75(2):263–74. doi: 10.1016/0092-8674(93)80068-p
111. Travis MA, Reizis B, Melton AC, Masteller E, Tang Q, Proctor JM, et al. Loss of integrin Alpha(V)Beta8 on dendritic cells causes autoimmunity and colitis in mice. Nature (2007) 449(7160):361–5. doi: 10.1038/nature06110
112. Sellon RK, Tonkonogy S, Schultz M, Dieleman LA, Grenther W, Balish E, et al. Resident enteric bacteria are necessary for development of spontaneous colitis and immune system activation in interleukin-10-Deficient mice. Infect Immun (1998) 66(11):5224–31. doi: 10.1128/iai.66.11.5224-5231.1998
113. Byrd AL, Deming C, Cassidy SKB, Harrison OJ, Ng WI, Conlan S, et al. Staphylococcus aureus and staphylococcus epidermidis strain diversity underlying pediatric atopic dermatitis. Sci Transl Med (2017) 9(397):eaal4651. doi: 10.1126/scitranslmed.aal4651
114. Kong HH, Oh J, Deming C, Conlan S, Grice EA, Beatson MA, et al. Temporal shifts in the skin microbiome associated with disease flares and treatment in children with atopic dermatitis. Genome Res (2012) 22(5):850–9. doi: 10.1101/gr.131029.111
115. Elias MS, Long HA, Newman CF, Wilson PA, West A, McGill PJ, et al. Proteomic analysis of filaggrin deficiency identifies molecular signatures characteristic of atopic eczema. J Allergy Clin Immunol (2017) 140(5):1299–309. doi: 10.1016/j.jaci.2017.01.039
116. Kezic S, O'Regan GM, Lutter R, Jakasa I, Koster ES, Saunders S, et al. Filaggrin loss-of-Function mutations are associated with enhanced expression of il-1 cytokines in the stratum corneum of patients with atopic dermatitis and in a murine model of filaggrin deficiency. J Allergy Clin Immunol (2012) 129(4):1031–9.e1. doi: 10.1016/j.jaci.2011.12.989
117. Shale M, Schiering C, Powrie F. Cd4(+) T-cell subsets in intestinal inflammation. Immunol Rev (2013) 252(1):164–82. doi: 10.1111/imr.12039
118. Peterson LW, Artis D. Intestinal epithelial cells: regulators of barrier function and immune homeostasis. Nat Rev Immunol (2014) 14(3):141–53. doi: 10.1038/nri3608
119. Zigmond E, Bernshtein B, Friedlander G, Walker CR, Yona S, Kim KW, et al. Macrophage-restricted interleukin-10 receptor deficiency, but not il-10 deficiency, causes severe spontaneous colitis. Immunity (2014) 40(5):720–33. doi: 10.1016/j.immuni.2014.03.012
120. Round JL, Mazmanian SK. Inducible Foxp3+ regulatory T-cell development by a commensal bacterium of the intestinal microbiota. Proc Natl Acad Sci U.S.A. (2010) 107(27):12204–9. doi: 10.1073/pnas.0909122107
121. Prinz JC. Melanocytes: target cells of an hla-C*06:02-Restricted autoimmune response in psoriasis. J Invest Dermatol (2017) 137(10):2053–8. doi: 10.1016/j.jid.2017.05.023
122. Boniface K, Taïeb A, Seneschal J. New insights into immune mechanisms of vitiligo. G Ital Dermatol Venereol (2016) 151(1):44–54.
123. Robinson ES, Werth VP. The role of cytokines in the pathogenesis of cutaneous lupus erythematosus. Cytokine (2015) 73(2):326–34. doi: 10.1016/j.cyto.2015.01.031
124. Tanaka K, Asamitsu K, Uranishi H, Iddamalgoda A, Ito K, Kojima H, et al. Protecting skin photoaging by nf-kappab inhibitor. Curr Drug Metab (2010) 11(5):431–5. doi: 10.2174/138920010791526051
125. Tremante E, Ginebri A, Lo Monaco E, Benassi B, Frascione P, Grammatico P, et al. A melanoma immune response signature including human leukocyte antigen-e. Pigment Cell Melanoma Res (2014) 27(1):103–12. doi: 10.1111/pcmr.12164
126. Lai Y, Gallo RL. Toll-like receptors in skin infections and inflammatory diseases. Infect Disord Drug Targets (2008) 8(3):144–55. doi: 10.2174/1871526510808030144
127. Doebel T, Voisin B, Nagao K. Langerhans cells - the macrophage in dendritic cell clothing. Trends Immunol (2017) 38(11):817–28. doi: 10.1016/j.it.2017.06.008
128. Gebhardt T, Palendira U, Tscharke DC, Bedoui S. Tissue-resident memory T cells in tissue homeostasis, persistent infection, and cancer surveillance. Immunol Rev (2018) 283(1):54–76. doi: 10.1111/imr.12650
129. Dijkgraaf FE, Matos TR, Hoogenboezem M, Toebes M, Vredevoogd DW, Mertz M, et al. Tissue patrol by resident memory Cd8(+) T cells in human skin. Nat Immunol (2019) 20(6):756–64. doi: 10.1038/s41590-019-0404-3
130. Ma JZ, Russell TA, Spelman T, Carbone FR, Tscharke DC. Lytic gene expression is frequent in hsv-1 latent infection and correlates with the engagement of a cell-intrinsic transcriptional response. PloS Pathog (2014) 10(7):e1004237. doi: 10.1371/journal.ppat.1004237
131. Mark KE, Wald A, Magaret AS, Selke S, Olin L, Huang ML, et al. Rapidly cleared episodes of herpes simplex virus reactivation in immunocompetent adults. J Infect Dis (2008) 198(8):1141–9. doi: 10.1086/591913
132. Baral P, Udit S, Chiu IM. Pain and immunity: implications for host defence. Nat Rev Immunol (2019) 19(7):433–47. doi: 10.1038/s41577-019-0147-2
133. Meixiong J, Anderson M, Limjunyawong N, Sabbagh MF, Hu E, Mack MR, et al. Activation of mast-Cell-Expressed mas-related G-Protein-Coupled receptors drives non-histaminergic itch. Immunity (2019) 50(5):1163–71. doi: 10.1016/j.immuni.2019.03.013
134. Talbot J, Hahn P, Kroehling L, Nguyen H, Li D, Littman D. Feeding-dependent vip neuron-Ilc3 circuit regulates the intestinal barrier. Nature (2020) 579(7800):575–80. doi: 10.1038/s41586-020-2039-9
135. Seillet C, Luong K, Tellier J, Jacquelot N, Shen R, Hickey P, et al. The neuropeptide vip confers anticipatory mucosal immunity by regulating Ilc3 activity. Nat Immunol (2020) 21(2):168–77. doi: 10.1038/s41590-019-0567-y
136. Riol-Blanco L, Ordovas-Montanes J, Perro M, Naval E, Thiriot A, Alvarez D, et al. Nociceptive sensory neurons drive interleukin-23-Mediated psoriasiform skin inflammation. Nature (2014) 510(7503):157–61. doi: 10.1038/nature13199
137. Zhang S, Edwards T, Chaudhri V, Wu J, Cohen J, Hirai T, et al. Nonpeptidergic neurons suppress mast cells Via glutamate to maintain skin homeostasis. Cell (2021) 184(8):2151–66. doi: 10.1016/j.cell.2021.03.002
138. Kolter J, Feuerstein R, Zeis P, Hagemeyer N, Paterson N, d'Errico P, et al. A subset of skin macrophages contributes to the surveillance and regeneration of local nerves. Immunity (2019) 50(6):1482–97. doi: 10.1016/j.immuni.2019.05.009
139. Ohtsuka M, Miura T, Mori T, Ishikawa M, Yamamoto T. Occurrence of psoriasiform eruption during nivolumab therapy for primary oral mucosal melanoma. JAMA Dermatol (2015) 151(7):797–9. doi: 10.1001/jamadermatol.2015.0249
140. Bonigen J, Raynaud-Donzel C, Hureaux J, Kramkimel N, Blom A, Jeudy G, et al. Anti-Pd1-Induced psoriasis: a study of 21 patients. J Eur Acad Dermatol Venereol (2017) 31(5):e254–e7. doi: 10.1111/jdv.14011
141. Ayala-Fontánez N, Soler DC, McCormick TS. Current knowledge on psoriasis and autoimmune diseases. Psoriasis (Auckl) (2016) 6:7–32. doi: 10.2147/ptt.S64950
142. Bowcock A, Krueger J. Getting under the skin: the immunogenetics of psoriasis. Nat Rev Immunol (2005) 5(9):699–711. doi: 10.1038/nri1689
143. Parisi R, Iskandar I, Kontopantelis E, Augustin M, Griffiths C, Ashcroft D. National, regional, and worldwide epidemiology of psoriasis: systematic analysis and modelling study. BMJ (Clinical Res ed) (2020) 369:m1590. doi: 10.1136/bmj.m1590
144. Ran D, Cai M, Zhang X. Genetics of psoriasis: a basis for precision medicine. Precis Clin Med (2019) 2(2):120–30. doi: 10.1093/pcmedi/pbz011
145. Pociot F, Lernmark Å. Genetic risk factors for type 1 diabetes. Lancet (2016) 387(10035):2331–9. doi: 10.1016/s0140-6736(16)30582-7
146. Rozin A, Schapira D, Bergman R. Alopecia areata and relapsing polychondritis or mosaic autoimmunity? the first experience of Co-trimoxazole treatment. Ann Rheum Dis (2003) 62(8):778–80. doi: 10.1136/ard.62.8.778
147. Head L, Gorden N, Gulick RV, Amato CM, Frazer-Abel A, Robinson W, et al. Biomarkers to predict immune-related adverse events with checkpoint inhibitors. J Clin Oncol (2019) 37(8_suppl):131–. doi: 10.1200/JCO.2019.37.8_suppl.131
148. Fujisawa Y, Yoshino K, Otsuka A, Funakoshi T, Fujimura T, Yamamoto Y, et al. Fluctuations in routine blood count might signal severe immune-related adverse events in melanoma patients treated with nivolumab. J Dermatol Sci (2017) 88(2):225–31. doi: 10.1016/j.jdermsci.2017.07.007
149. Diehl A, Yarchoan M, Hopkins A, Jaffee E, Grossman SA. Relationships between lymphocyte counts and treatment-related toxicities and clinical responses in patients with solid tumors treated with pd-1 checkpoint inhibitors. Oncotarget (2017) 8(69):114268–80. doi: 10.18632/oncotarget.23217
150. Isono T, Kagiyama N, Takano K, Hosoda C, Nishida T, Kawate E, et al. Outcome and risk factor of immune-related adverse events and pneumonitis in patients with advanced or postoperative recurrent non-small cell lung cancer treated with immune checkpoint inhibitors. Thorac Cancer (2021) 12(2):153–64. doi: 10.1111/1759-7714.13736
151. Marur S, Singh H, Mishra-Kalyani P, Larkins E, Keegan P, Sridhara R, et al. Fda analyses of survival in older adults with metastatic non-small cell lung cancer in controlled trials of pd-1/Pd-L1 blocking antibodies. Semin Oncol (2018) 45(4):220–5. doi: 10.1053/j.seminoncol.2018.08.007
152. Kartolo A, Sattar J, Sahai V, Baetz T, Lakoff JM. Predictors of immunotherapy-induced immune-related adverse events. Curr Oncol (2018) 25(5):e403–e10. doi: 10.3747/co.25.4047
153. Cortellini A, Bersanelli M, Santini D, Buti S, Tiseo M, Cannita K, et al. Another side of the association between body mass index (Bmi) and clinical outcomes of cancer patients receiving programmed cell death protein-1 (Pd-1)/ programmed cell death-ligand 1 (Pd-L1) checkpoint inhibitors: a multicentre analysis of immune-related adverse events. Eur J Cancer (2020) 128:17–26. doi: 10.1016/j.ejca.2019.12.031
154. Abolhassani AR, Schuler G, Kirchberger MC, Heinzerling L. C-reactive protein as an early marker of immune-related adverse events. J Cancer Res Clin Oncol (2019) 145(10):2625–31. doi: 10.1007/s00432-019-03002-1
155. Hirashima T, Kanai T, Suzuki H, Yoshida H, Matsusita A, Kawasumi H, et al. Significance of pre-treatment interferon-gamma release in patients with non-Small-Cell lung cancer receiving immune checkpoint inhibitors. Anticancer Res (2020) 40(12):6971–8. doi: 10.21873/anticanres.14721
156. Devarakonda S, Rotolo F, Tsao MS, Lanc I, Brambilla E, Masood A, et al. Tumor mutation burden as a biomarker in resected non-Small-Cell lung cancer. J Clin Oncol (2018) 36(30):2995–3006. doi: 10.1200/JCO.2018.78.1963
157. Bomze D, Hasan Ali O, Bate A, Flatz L. Association between immune-related adverse events during anti-Pd-1 therapy and tumor mutational burden. JAMA Oncol (2019) 5(11):1633–5. doi: 10.1001/jamaoncol.2019.3221
158. Nassar A, Adib E, Abou Alaiwi S, El Zarif T, Groha S, Akl E, et al. Ancestry-driven recalibration of tumor mutational burden and disparate clinical outcomes in response to immune checkpoint inhibitors. Cancer Cell (2022) 40(10):1161–72. doi: 10.1016/j.ccell.2022.08.022
159. Vikas P, Messersmith H, Compton C, Sholl L, Broaddus R, Davis A, et al. Mismatch repair and microsatellite instability testing for immune checkpoint inhibitor therapy: asco endorsement of college of American pathologists guideline. J Clin Oncol Off J Am Soc Clin Oncol (2023) 41(10):1943–8. doi: 10.1200/JCO.22.02462
160. Ciombor K, Eng C. Immunotherapy in localized microsatellite instability-High/Mismatch repair deficient solid tumors: are we ready for a new standard of care? J Clin Oncol (2023) 41(12):2138–40. doi: 10.1200/jco.22.02564
161. Patel SP, Kurzrock R. Pd-L1 expression as a predictive biomarker in cancer immunotherapy. Mol Cancer Ther (2015) 14(4):847–56. doi: 10.1158/1535-7163.Mct-14-0983
162. Petukhova L, Duvic M, Hordinsky M, Norris D, Price V, Shimomura Y, et al. Genome-wide association study in alopecia areata implicates both innate and adaptive immunity. Nature (2010) 466(7302):113–7. doi: 10.1038/nature09114
163. He X, Yu J, Shi H. Pan-cancer analysis reveals alternative splicing characteristics associated with immune-related adverse events elicited by checkpoint immunotherapy. Front Pharmacol (2021) 12:797852. doi: 10.3389/fphar.2021.797852
164. Caulfield J, Aizenbud L, Perdigoto A, Meffre E, Jilaveanu L, Michalek D, et al. Germline genetic variants are associated with development of insulin-dependent diabetes in cancer patients treated with immune checkpoint inhibitors. J Immunother Cancer (2023) 11(3):e006570. doi: 10.1136/jitc-2022-006570
165. Xin Z, You L, Na F, Li J, Chen M, Song J, et al. Immunogenetic variations predict immune-related adverse events for pd-1/Pd-L1 inhibitors. Eur J Cancer (Oxford Engl 1990) (2023) 184:124–36. doi: 10.1016/j.ejca.2023.01.034
166. Bukhari S, Henick BS, Winchester RJ, Lerrer S, Adam K, Gartshteyn Y, et al. Single-cell rna sequencing reveals distinct T cell populations in immune-related adverse events of checkpoint inhibitors. Cell Rep Med (2023) 4(1):100868. doi: 10.1016/j.xcrm.2022.100868
167. Abed A, Law N, Calapre L, Lo J, Bhat V, Bowyer S, et al. Human leucocyte antigen genotype association with the development of immune-related adverse events in patients with non-small cell lung cancer treated with single agent immunotherapy. Eur J Cancer (2022) 172:98–106. doi: 10.1016/j.ejca.2022.05.021
168. Hasan Ali O, Berner F, Bomze D, Fässler M, Diem S, Cozzio A, et al. Human leukocyte antigen variation is associated with adverse events of checkpoint inhibitors. Eur J Cancer (2019) 107:8–14. doi: 10.1016/j.ejca.2018.11.009
169. Horton R, Gibson R, Coggill P, Miretti M, Allcock RJ, Almeida J, et al. Variation analysis and gene annotation of eight mhc haplotypes: the mhc haplotype project. Immunogenetics (2008) 60(1):1–18. doi: 10.1007/s00251-007-0262-2
170. Marschner D, Falk M, Javorniczky NR, Hanke-Müller K, Rawluk J, Schmitt-Graeff A, et al. Microrna-146a regulates immune-related adverse events caused by immune checkpoint inhibitors. JCI Insight (2020) 5(6):e132334. doi: 10.1172/jci.insight.132334
171. Hollern D, Xu N, Thennavan A, Glodowski C, Garcia-Recio S, Mott K, et al. B cells and T follicular helper cells mediate response to checkpoint inhibitors in high mutation burden mouse models of breast cancer. Cell (2019) 179(5):1191–206. doi: 10.1016/j.cell.2019.10.028
172. Zapata L, Caravagna G, Williams MJ, Lakatos E, AbdulJabbar K, Werner B, et al. Immune selection determines tumor antigenicity and influences response to checkpoint inhibitors. Nat Genet (2023) 55(3):451–60. doi: 10.1038/s41588-023-01313-1
173. Loriot Y, Marabelle A, Guégan J, Danlos F, Besse B, Chaput N, et al. Plasma proteomics identifies leukemia inhibitory factor (Lif) as a novel predictive biomarker of immune-checkpoint blockade resistance. Ann Oncol Off J Eur Soc Med Oncol (2021) 32(11):1381–90. doi: 10.1016/j.annonc.2021.08.1748
174. Sánchez-Magraner L, Gumuzio J, Miles J, Quimi N, Martínez Del Prado P, Abad-Villar M, et al. Functional engagement of the pd-1/Pd-L1 complex but not pd-L1 expression is highly predictive of patient response to immunotherapy in non-Small-Cell lung cancer. J Clin Oncol (2023) 41(14):2561–70. doi: 10.1200/jco.22.01748
175. Huang C, Chen L, Savage S, Eguez R, Dou Y, Li Y, et al. Proteogenomic insights into the biology and treatment of hpv-negative head and neck squamous cell carcinoma. Cancer Cell (2021) 39(3):361–79. doi: 10.1016/j.ccell.2020.12.007
176. Nie X, Xia L, Gao F, Liu L, Yang Y, Chen Y, et al. Serum metabolite biomarkers predictive of response to pd-1 blockade therapy in non-small cell lung cancer. Front Mol Biosci (2021) 8:678753. doi: 10.3389/fmolb.2021.678753
177. Kocher F, Amann A, Zimmer K, Geisler S, Fuchs D, Pichler R, et al. High indoleamine-2,3-Dioxygenase 1 (Ido) activity is linked to primary resistance to immunotherapy in non-small cell lung cancer (Nsclc). Trans Lung Cancer Res (2021) 10(1):304–13. doi: 10.21037/tlcr-20-380
Keywords: immune checkpoint inhibitors, cutaneous immune-related adverse events, multi-omics, biomarkers, cutaneous
Citation: Cao T, Zhou X, Wu X and Zou Y (2023) Cutaneous immune-related adverse events to immune checkpoint inhibitors: from underlying immunological mechanisms to multi-omics prediction. Front. Immunol. 14:1207544. doi: 10.3389/fimmu.2023.1207544
Received: 17 April 2023; Accepted: 05 June 2023;
Published: 22 June 2023.
Edited by:
Maria-Ioanna (Marianna) Christodoulou, European University Cyprus, CyprusReviewed by:
Zhen Zong, Nanchang University, ChinaJiatong Ding, Nanchang University, China
in collaboration with reviewer ZZ
Philippe Lefrançois, McGill University, Canada
Copyright © 2023 Cao, Zhou, Wu and Zou. This is an open-access article distributed under the terms of the Creative Commons Attribution License (CC BY). The use, distribution or reproduction in other forums is permitted, provided the original author(s) and the copyright owner(s) are credited and that the original publication in this journal is cited, in accordance with accepted academic practice. No use, distribution or reproduction is permitted which does not comply with these terms.
*Correspondence: Ying Zou, zouyingsh@163.com