- 1Department of Chemical Engineering, University of Puerto Rico-Mayagüez, Mayagüez, Puerto Rico
- 2Cancer Research Clinic, Carolina BioOncology Institute (CBOI), Huntersville, NC, United States
- 3Human Applications Lab (HAL) - BioCytics, Huntersville, NC, United States
Cellular immunotherapy has revolutionized the oncology field, yielding improved results against hematological and solid malignancies. NK cells have become an attractive alternative due to their capacity to activate upon recognition of “stress” or “danger” signals independently of Major Histocompatibility Complex (MHC) engagement, thus making tumor cells a perfect target for NK cell-mediated cancer immunotherapy even as an allogeneic solution. While this allogeneic use is currently favored, the existence of a characterized memory function for NK cells (“memory-like” NK cells) advocates for an autologous approach, that would benefit from the allogeneic setting discoveries, but with added persistence and specificity. Still, both approaches struggle to exert a sustained and high anticancer effect in-vivo due to the immunosuppressive tumor micro-environment and the logistical challenges of cGMP production or clinical deployment. Novel approaches focused on the quality enhancement and the consistent large-scale production of highly activated therapeutic memory-like NK cells have yielded encouraging but still unconclusive results. This review provides an overview of NK biology as it relates to cancer immunotherapy and the challenge presented by solid tumors for therapeutic NKs. After contrasting the autologous and allogeneic NK approaches for solid cancer immunotherapy, this work will present the current scientific focus for the production of highly persistent and cytotoxic memory-like NK cells as well as the current issues with production methods as they apply to stress-sensitive immune cells. In conclusion, autologous NK cells for cancer immunotherapy appears to be a prime alternative for front line therapeutics but to be successful, it will be critical to establish comprehensives infrastructures allowing the production of extremely potent NK cells while constraining costs of production.
1 Introduction
Immunotherapy is a therapeutic approach that harnesses the immune system against cancer. It has revolutionized the oncology field in terms of effectiveness and offers personalized, targeted treatment options that ultimately yield improved results when compared to surgery, chemotherapy, and/or radiation treatments. The key attributes of cancer immunotherapy are detecting, surveilling, and destroying neoplasm cells using immune cells. To date, six cellular therapy products, all autologous CAR-T cells against hematological malignancies, have been approved by the U.S. Food and Drug Administration (FDA) (https://www.cancer.gov). These treatments require a tailored product for each individual patient. As a consequence of the successful results obtained with CAR-T cells, the use and engineering potentiation to fight cancer of different immune cell types (1), such as dendritic cells, macrophages, and natural killer (NK) cells, has seen increased interest (2).
NK cells, monocytes, macrophages, and to some degree, dendritic cells constitute the innate lymphoid cell family, which is the first line of defense against invasive pathogens and transformed cells in the human body (3, 4). The discovery and characterization of NK cells dates back to 1975 when Herberman et al. and Kiessling et al. found a natural cytotoxic activity of a subpopulation of lymphoid cells against cancer cells in mouse studies (5, 6). The use of innate lymphocytes NK cells is attractive because of their unique ability to recognize cancer cells and exert antitumor cell cytotoxicity (7). Innate NK cells offer a very attractive approach to cancer therapy. Since NK cells base their initial recognition on particular “stress” or “danger” signals, tumor cells become a perfect target for NK cells.
NK cell cytotoxicity is exerted through a delicate balance mechanism of activating and inhibiting surface receptors, without an antibody or MHCI-strict dependence, as detailed in Figure 1 (1, 8, 11). The lack of expression of CD3 characterizes the human NK cell population, but it expresses the CD56 and CD16 cell surface markers and is classified into two main subsets (3). CD56brightCD16lo/- NK cells are poorly cytotoxic and mainly produce pro-inflammatory cytokines after cytokine stimulation. CD56dimCD16+ NK cells, which represent about 90% of peripheral blood NK cells, are highly cytotoxic, but expand poorly and do not show a significant de-novo cytokine production (3, 12). Unsurprisingly CD16, also known as FcγRIIIA, was characterized early as critical to the proper activation of the ADCC (Antibody-Dependent Cell Cytotoxicity) response (13). These NK cell subsets also possess metabolic differences; for example, under cytokine stimulation, the CD56bright subset is more susceptible to upregulating expression of nutrients receptors, amino acid transporters, and transferring receptors than the CD56dim subset (12, 14, 15). However, several studies agree that both subsets, CD56bright and CD56dim, can be cytotoxic or produce cytokines after suitable in vitro stimulation (3).
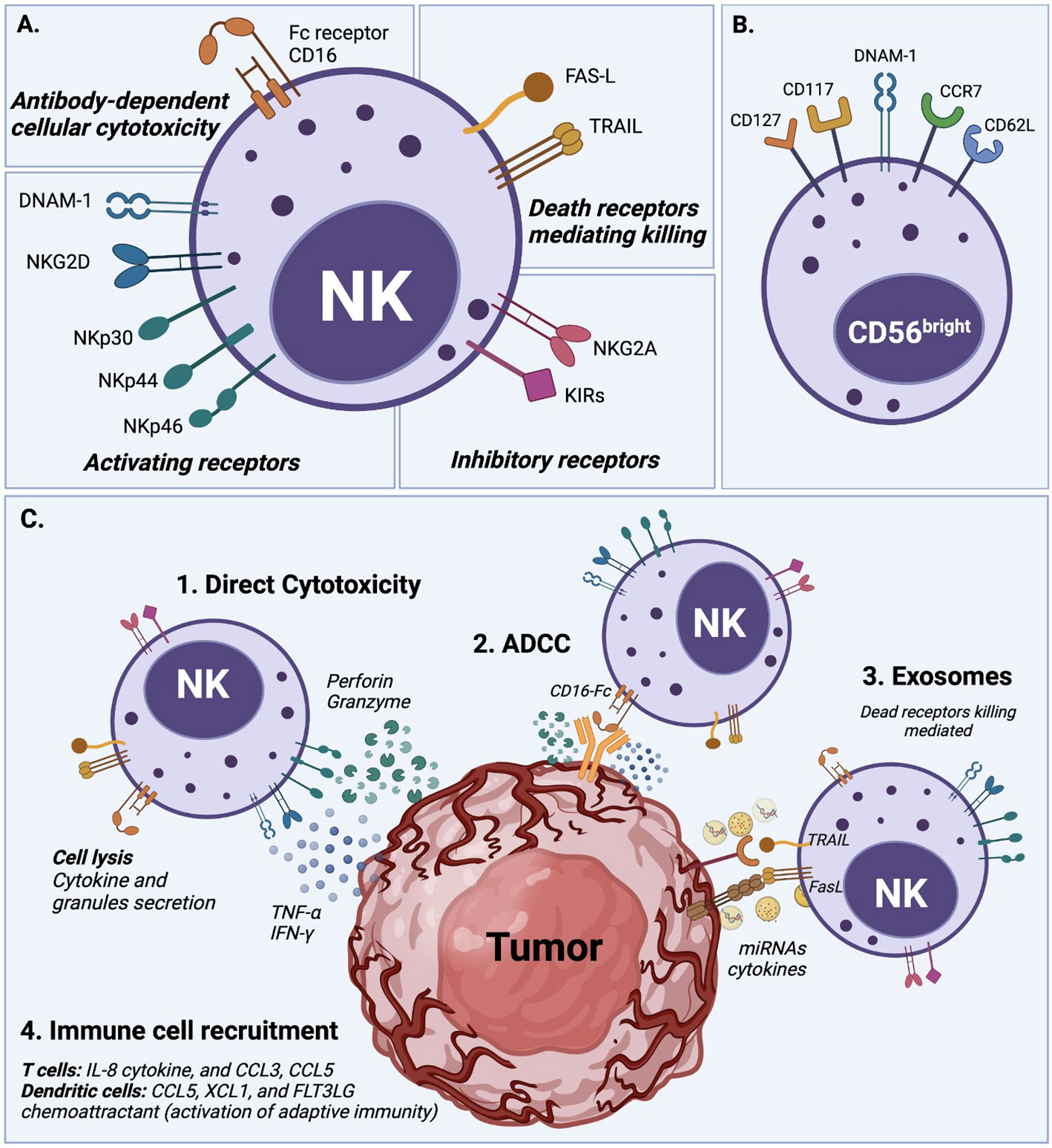
Figure 1 NK cell activation and natural cytotoxicity. (A) NK cells have a natural ability to recognize abnormal cells and exert cell cytotoxicity through different mechanisms. (1) NK cells exert direct cytotoxicity against compromised cells mainly by the secretion of IFN-γ and TNF-α cytokines, and the release of perforin and granzyme cytolytic granules. (2) Antibody-dependent cellular cytotoxicity (ADCC) is another mechanism of NK cell cytotoxicity, which is mediated by the CD16 (FcRIII) receptor after recognizing and bound target cells. (3) NK cells display dead receptors, FAS-L and TRAIL, and exert their killing capability against compromised cells through the release of exosome that contains small non-coding RNAs (miRNAs) and cytokines (8–10). (4) The immune cell recruitment of T cells and dendritic cells also plays an essential role in NK cell cytotoxicity to enhance their action through soluble cytokine IL-2 and chemokine (CCL3, CCL5, XCL1, and FLT3LG) secretion. (B) Overview of NK cell receptors. All described cytotoxicity mechanisms are in response to an orchestrated balance of activating receptors (e.g., DNAM-1, NKG2D, NKp30, NKp44, NKp46) and inhibitory receptors (e.g., NKG2A, KIRs family) that determine the NK cell activation state. (C) Phenotype of circulating CD56bright NK cells which typically express DNAM-1, CD62L (L-selectin), and CCR7, but also in some cases CD117 (c-kit), and CD127 (IL-7Rα).
Target cell recognition and NK cell activation occur through different mechanisms (see Figure 1). For example, target cells can be recognized through the overexpression of ligands for the NK cell activating receptors such as natural killer group 2D (NKG2D), costimulatory receptors (DNAX accessory molecule-1 DNAM-1), and natural cytotoxicity receptors (NKp46). The ligands for these receptors are MHC class I chain-related MIC or UL16-Binding Proteins ULBPs (16), Nectin-2 or Poliovirus Receptor PVR (17), and B7H6 (18), respectively. Another target cell recognition mechanism is through a low-level expression of ligands for NK cell inhibitory receptors (e.g., KIRs family) such as MHC-I or the non-classical HLA-E for NKG2A (8, 19, 20). NK cells will kill cells that express low levels of MHC-I or HLA-E. Therefore, cancer cells that downregulate MHC-I molecules to evade T cell cytotoxicity can be targets for NK cells (21). Once target cells are recognized, NK cells exert their cytotoxicity by secreting cytokines and chemokines. They release perforin and granzyme cytolytic granules upon direct contact with the target malignant cell to cause cell apoptosis. NK cells also generate interferon-gamma (IFN-γ) and tumor necrosis factor-alpha (TNF-α), which exert antitumor effects and upregulate other immune responses (11, 19, 22).
All these features make NK cells particularly useful for autologous and allogeneic cancer immunotherapy, since they were recognized early on for their potential use in the allogeneic setting as an “off-the-shelf” product (23). Still, some challenges are standing before a successful anticancer treatment can be implemented, including short-term persistence, sensitivity, and clinical-grade ex vivo expansion (24). Despite the encouraging results of NK cells against hematological malignancies and solid tumors, the tumor microenvironment presents a steep challenge to the success of cancer immunotherapies. In the past decade, it was found that NK cells can exhibit memory-like functions under certain circumstances. This memory function can provide superior host protection compared to naive NK cells, due to their faster recognition and higher cytotoxic responses against tumor cells (25, 26). It could be a powerful property if successfully leveraged against solid tumors.
In this review, we will provide the traditional overview of the use of NK cells in cancer immunotherapy along with their autologous and allogeneic approaches. Allogeneic NK cell therapy dominates the adoptive NK cell-mediated cancer immunotherapy. Despite this fact, ongoing research and some clinical trials with autologous settings suggest its use as an alternative when the allogeneic setting is not applicable or unavailable (27). Then, the characteristics of NK cells memory function and the memory-like NK cell production for NK cell-mediated cancer immunotherapy will be described. Finally, the enhancement of non-genetically modified autologous NK cells will be shown along with their cancer therapeutic advantages when compared to genetically engineered or allogeneic cells.
2 NK cells in cancer immunotherapy
Since the identification of the NK cell population in the 1970s (5, 6), this immune cell lineage has been used as an anticancer treatment because of its capability to identify and kill malignant cells without priming, in an MHC-I non-restricted manner (28–30). In contrast to T cells, NK cells do not need prior antigen priming by MHC-I molecules to mediate their killing capability (28, 30), instead relying on a state of equilibrium that can be influenced by recognition of multiple markers of “missing self” (missing classical of non-classical MHC markers) or “induced self-recognition” (induced markers of abnormal-self, for example generated upon virus infection). The use of NK cells to fight hematological malignancies, including leukemia, lymphoma, and multiple myeloma, yielded encouraging results in pre-clinical and clinical studies (31). These favorable outcomes stem from different mechanisms that NK cells exert against tumor cells. In normal conditions, healthy cells of the human body have either absent or low expression of ligands for activating receptors. When compromised, stressed, or modified, cells upregulate these ligands, becoming more sensitive to NK cells (32). This mechanism, along with the tolerance of a KIR-HLA mismatch between recipient and donors, makes NK cells suitable for an “off-the-shelf” allogeneic cancer immunotherapy product. This allogeneic approach is based on the NK cells’ ability to recognize, direct, and rapidly lyse cancerous, stressed, and viral-infected cells without MHC-dependent priming (33–35). It avoids the rejection of self-MHC recognition and has a low risk of cytokine release syndrome (“cytokine storm”), graft-versus-host disease (GvHD), and other immune-associated off-target events observed with CAR-T cells (2, 24). Additionally, it was corroborated that autologous and allogeneic NK cells are a fantastic alternative therapy when cancer cells become resistant to chemotherapy, T cells, or checkpoint immunotherapy (29, 30, 36).
Allogeneic NK cell immunotherapy has been investigated in several clinical trials. It has been used not only against hematologic malignancies, but also against solid tumors, yielding encouraging results even with the immunocompromising tumor microenvironment. Melanoma, neuroblastoma, breast cancer, hepatocellular cancer, ovarian cancer, renal cell carcinoma, and colorectal cancer are examples of treated solid tumors (1, 4, 37). To date, more than 150 clinical trials of NK cells against cancer have been developed. Table 1 illustrates 15 out of 150 trials of NK cells against cancer. These 15 trials explicitly focused on fighting solid tumors using allogeneic cells; most of them were completed, but two were terminated due to toxicity (NCT00582816) or the death of 2 patients (NCT01337544).
3 Autologous NK therapy against cancer
The principle of allogeneic transplant has been explored since the early 1950s (39), and allogeneic therapies are defined by the transplant of biological material from a donor to a host in order to cure a disease. This approach has been intensively sought by researcher as a tool against cancer since it would allow for an “off-the-shelve”, universal solution (40). NK cells are a privileged tool for allogeneic immunotherapies mainly because, unlike cytotoxic T-Cells, their MHC independence does not trigger Graft vs. Host Disease (GvHD) (41). Because of this obvious advantage, the consideration to use the own patient NK cells (autologous setting) has received little attention. Ongoing research with autologous settings suggests it is a promising strategy when allogeneic cells are limited or unavailable (27). Autologous products are simpler to obtain and avoid some challenges of human leukocyte antigen (HLA) mismatched cellular therapy, such as the failure to persist for more than a few weeks in an immune incompatible environment (42–44). Another example is the off-target activation of allogeneic cell products due to the abundance of targets in the non-immune compatible recipient that can result in NK cell misdirection, exhaustion, or anergy (44). Additionally, the use of chemotherapy is needed before allogeneic therapy injection to avoid immunological rejection of the recipient (42, 45). Table 2 summarizes the advantages and limitations offered by both types of NK cell therapy, autologous and allogeneic settings.
The initial clinical autologous NK cell therapy was conducted in 2003 and used IL-2 ex vivo cytokine-activated NK cells. The results of this trial did not produce significant improvements in patient disease outcomes of lymphoma and breast cancer (46). More recently, Nahi et al. performed multiple infusion doses of ex vivo activated and expanded autologous NK cells in multiple myeloma (MM) patients. This treatment resulted in a reduction or minimal residual disease with increased NK cell circulation and granzyme B levels, persisting for several weeks after the last infusion (27). Another clinical trial used autologous NK cells combined with Bortezomib against different solid tumors such as Chronic Myeloid Leukemia (CML), MM, carcinoma, pancreatic, colon/rectal, and non-small-cell lung cancer (NCT00720785). Also, 12 young patients with recurrent or refractory brain tumors were the target in a phase I clinical trial (NCT02271711) that evaluated autologous ex vivo expanded NK cells. These two trials yielded no conclusive data.
In another study, patients with metastatic digestive cancer, whose standard therapy failed, were enrolled in a phase I clinical trial to be treated with ex vivo expanded NK cells (UMIN000007527). Expanded and γ-irradiated PBMCs were used as feeder cells to stimulate NK-cell expansion. Injected NK cells were demonstrated to have high in vitro lytic action and strong expression of NKG2D and CD16. Even when no clinical responses were observed, the therapy did not show severe adverse events, and the cytotoxicity of peripheral NK cells was elevated up to 4 weeks following the last transfusion (47). Bae et al. performed a phase I clinical trial of autologous expanded and activated NK cell therapy. Cells were administered for 5 consecutive days in a dose-escalating manner combined with chemotherapy in 11 hepatocellular carcinoma patients. They observed a disease control rate of 81.8% with no decompensation or adverse events (48). Similarly, pediatric medulloblastoma and ependymoma were treated with intraventricular infusions of ex vivo expanded autologous NK cells. NK cells were expanded from PBMCs by co-culturing with irradiated K562 feeder cells genetically modified to express costimulatory molecules. In this case, 8 of the 9 patients still showed progressing disease. Nevertheless, NK cells increased in the cerebrospinal fluid when higher dose levels with repetitive infusions were used. The authors suggested that these findings support additional studies of NK cell infusions against brain malignancies in children (49).
Although many strategies efficiently generate large quantities of expanded NK cells ex vivo, it is still unclear whether the expanded NK cells can persist/proliferate in vivo without exogenous human cytokines. Vahedi et al., in a pre-clinical study with humanized mice, demonstrated that autologous human immune cells support the in vivo survival of ex vivo expanded human NK cells. They administrated ex vivo expanded human cord blood (HCB) derived NK cells into humanized mice reconstituted with autologous HCB immune cells. In contrast to the control mice lacking human immune cells, NK cells could survive and possibly proliferate in vivo without exogenous cytokine administration (50). This study underlines the benefits of the autologous setting to maintain the correct immune homeostasis and feedback mechanisms.
Despite these efforts, the success of autologous NK cell therapies has been affected by the aggressiveness of malignant cells, tumor escape, and manufacturing letdowns due to the low number and compromised function of patient-derived NK cells. For that reason, several approaches emerged to enhance their antibody-dependent cellular cytotoxicity (ADCC). Some examples include the addition of checkpoint receptor blockers, antitumor monoclonal antibodies, bi-/tri-specific killer engagers (BiKEs and TriKEs), and the use of memory-type NK cells, which showed beneficial effects (51). Additionally, therapeutic applications recently explored the targeting of NK cells with memory functions, acquired during a previous activation (52, 53).
4 Memory-type NK cells
Under certain conditions, NK cells display adaptive and memory-like features against different pathogens, such as viruses, including HCMV (54), HIV (55), chikungunya (56), and influenza (57). These findings primed the interest of researchers to explore the NK cell memory potential for cancer treatment of hematologic malignancies and solid tumors. The ability of the immune system to remember and rapidly respond against prior-faced pathogens is defined as immunological memory, which is mainly considered a characteristic of adaptive immune cells. Recent studies indicate that innate immune cells can also exert memory function against a previously encountered pathogen (52, 53). Memory-like (ML) NK cells can ignore some inhibitory receptors, such as KIR, and exhibit higher expression of the activating receptors, such as NKG2D, NKp46, and DNAM-1, suggesting their potential effectiveness in recognizing additional tumor types (26). ML NK cells show various features, such as longer persistence, higher proliferation, and extended effector function (58), that provide superior host protection, higher expansion, and higher IFN-γ production than conventional NK cells (59). In humans, the increased output of IFN-γ by ML NKs was correlated to the expression of NKp46, CD94, NKG2A, and CD69 receptors, as well as the lack of CD57 and KIR receptors (56). Additionally, it was found that the inhibitory receptor NKG2A is a dominant checkpoint for the ML NK-cell phenotype, and that CD8α+ donor NK cells correlate to treatment failure against AML acute myeloid leukemia (AML) (60). We summarized the specific features of ML NK cells in comparison to classical NK features in Table 3.
The use of ML NK cells has been considered reliable and sufficient to stimulate remissions in patients with hematological malignancies such as AML and multiple myeloma (MM) patients (60, 70). Activated NK cells effectively targeted and killed compromised cells in pediatric and adult patients with acute lymphoblastic leukemia (ALL). Boieri et al. demonstrated that Roser leukemia was highly resistant to autologous NK cells. That resistance could be overcome using autologous NK cells pre-activated with IL-12, IL-15, and IL-18 cytokines in vitro and in vivo (71). Romee et al. demonstrated that ML NK cells induced with IL-12, IL-15, and IL-18 cytokines had highly functional responses, enhanced INF-γ production, and higher cytotoxicity when stimulated in vitro with primary human AML blasts and against several myeloid leukemia cell lines. Also, good clinical responses were observed in five of nine patients, including four complete remissions (72). The in vitro activation of ML NK cells was also shown to have a greater and longer capacity to contain the in vivo growth of multiple myeloma cell lines compared to IL-2 activated NK cells (73).
ML NK cells also exhibited promising in vitro results for treating solid tumors, encouraging their use in the early phases of clinical studies (see Table 1). For example, human ML NK cells were injected in NSG (NOD scid gamma) mouse models, demonstrating higher control of melanoma xenografts compared to conventional NK cells maintained with IL-15 (26). Uppendahl et al. studied the interaction of ML NK cells from healthy donors and melanoma patients against melanoma targets and established the superior cytokine production (IFN-γ) and cytotoxicity of ML NKs. They correlated that these ML NK-cell responses against autologous targets were related to the activatory receptors NKG2D and NKp46. ML NK cells also showed enhanced in vitro production of TNF-α and IFN-γ against different ovarian cancer cell lines, including SKOV-3, MA148, OVCAR5, and A1847, but also controlled the MA148 tumor growth of an in vivo xenogeneic mouse model (74). Moreover, ML NK cells isolated from hepatocellular carcinoma (HCC) patients and healthy donors were identified as potential effectors against HCC by trafficking to the liver to exert a cytolytic activity responsible for lower levels of spontaneous HCC formation (75). Tanzi et al. studied ML NK cells against solid tumors and demonstrated that these cells were able to lyse autologous tumors, including lung, liver, and peritoneum tumors. Of note, in this study, the antitumor activity of IL-12, IL-15, and IL-18 cytokine-activated NK cells was superior to that obtained with IL-2 stimulated NK cells (76). Another study demonstrated that ML NK cells cultured in the presence of IL-2 and IL-21 showed enhanced expansion and cytotoxicity against K562 cells. This enhanced bioactivity was correlated to the upregulation of the markers CD69 and CD25, along with phosphorylation of signal transducer and activator of transcription (STAT) 1 and 3 (68).
In summary, the use of memory-like NK cells has shown to be advantageous and a promising alternative to improve the current results obtained with NK cell immunotherapy. However, to translate these advantages into a clinical setting, it is imperative that efficient methods can be deployed for the large, stable production of these cells.
5 Targeting the immunosuppressive tumor microenvironment
One of the significant challenges in using NK cell-mediated immunotherapy is the cells’ low activity when deployed against solid tumors, due to the several immunosuppressive mechanisms that the tumor microenvironment (TME) uses, as illustrated by Figure 2. The TME establishes immune evasion mechanisms that include acidification (low pH), hypoxia, decreased expression of tumor-associated antigens, and low nutrients. Also, the TME upregulates the expression of inhibitory ligands and downregulates transcriptional ligands complementary to NK cell-activating receptors (4, 77, 78).
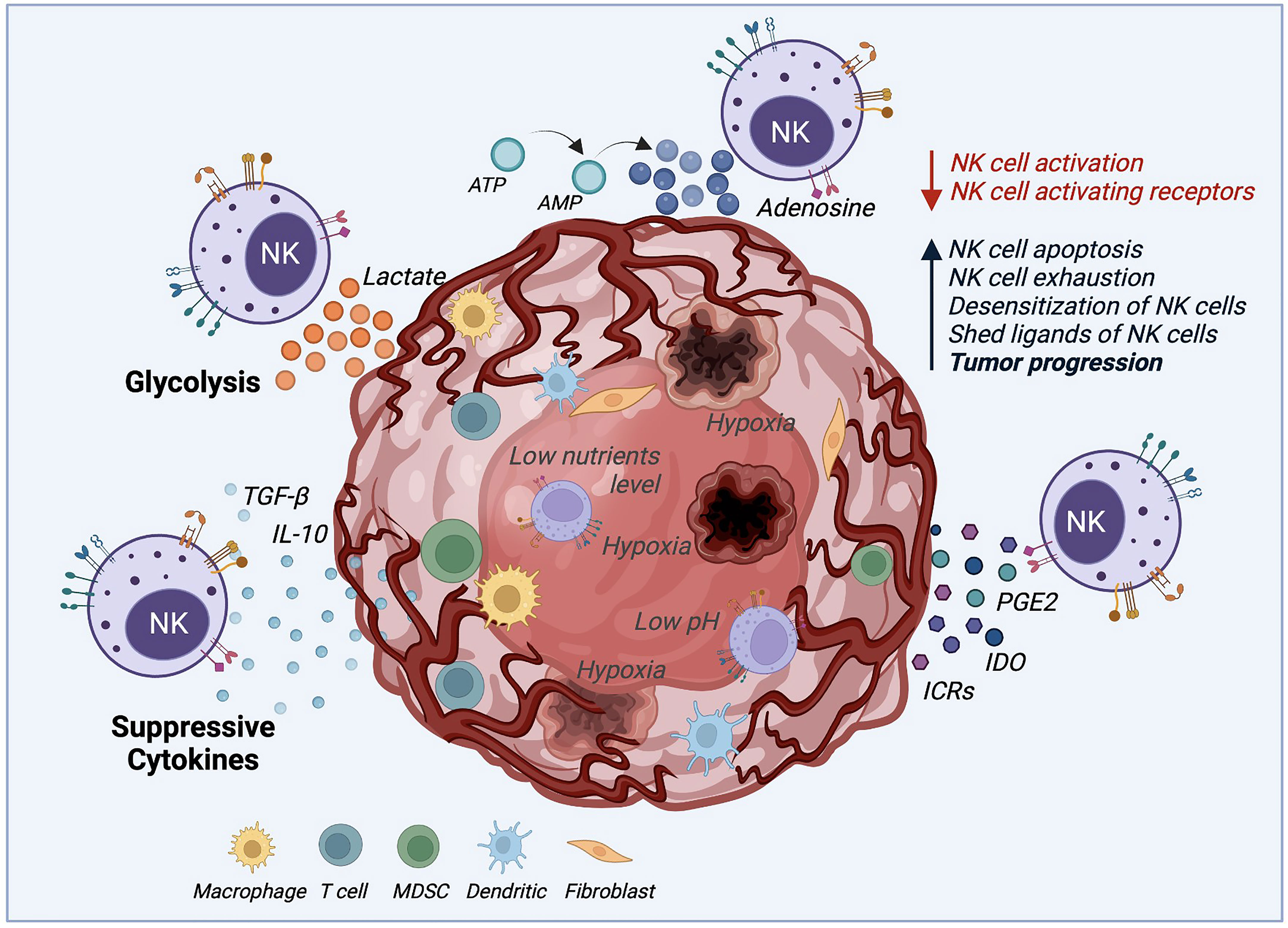
Figure 2 Tumor microenvironment (TME) mechanisms inhibiting NK cell cytotoxicity. The TME deploys several mechanisms to survive and increase the tumor progression rate by desensitizing NK cells and decreasing NK cell activation. These mechanisms negatively impact NK cell cytotoxicity by boosting NK cell apoptosis, exhaustion, and proliferation. Suppressive cytokines such as TGF-β and IL-10, immune-checkpoint receptors (ICRs), indoleamine 2,3-dioxygenase (IDO), prostaglandin E2 (PGE2) are secreted by the TME to desensitize NK cells. Also, the production of lactate and adenosine, along with hypoxia, and low levels of nutrients and pH, inhibits the NK cell function against solid tumors.
For example, the activating natural killer group 2D (NKG2D) receptor is highly involved in NK cell-mediated tumor surveillance. Specific tumor cells developed a mechanism to evade NKG2D recognition by shedding and saturating the NKG2D receptor with NKG2D soluble ligand (79). This action, in addition to allowing cancer cells to hide from the effect of NK cells, also leads to the desensitization of NKG2D-mediated NK cell activation since high levels of NKG2D ligand shedding cause the downregulation of NKG2D signaling (80). Another immunosuppressive mechanism example is the increase of associated factors, including tumor-secreted factors (TSF) and tumor-secreted exosomes (TSE) (2). Some examples of TSE or tumor cytokine expression are the transforming growth factor (TGF)-β, tumor necrosis factor (TNF), vascular endothelial growth factor (VEGF), and IL-10 that induce the upregulation of key molecules for tumor cell proliferation and suppress the adaptive antitumor immune response (2, 4, 81). It was demonstrated that TGF-β leads to NK cell differentiation and reduces the expression of NK cell activating receptors NKG2D, NKp30, and DNAM-1, which is related to their lack of efficient activation (81, 82). Another factor that represents a challenge for NK cells, when facing cancer cells, is their ability to maintain normal MHC-I expression. This results in the inhibition of NK cell activation by binding to NKG2A and KIR (Killer cell Immunoglobulin-like Receptors). These receptors act as immune checkpoints, preventing NK-mediated cancer cell destruction (83).
Moreover, tumor metabolic activity, mitophagy, and oxygen availability also affect the NK cell responses (24). Tumor glycolysis can drive lactic acid accumulation in the TME. NK cells ex vivo culture with 15 mM lactic acid results in a totally blocked IFN-γ production, inhibiting NK cell proliferation and tumor surveillance in melanomas (84). Similarly, the mitochondrial fragmentation caused by hypoxia may be an essential factor to consider for NK cell therapy success (24). Zheng et al. found that mitochondrial fragmentation induced a loss of NK cell cytotoxicity, helping tumor evasion from NK cell-mediated surveillance (85). Hypoxia, along with nutrient shortage, acidic environment, abnormal vasculature, and high pressure, also plays an essential role in TME. Hypoxia not only stimulates the TSE release and attracts bone marrow-derived cells (BMDCs), but it may also increase neoplastic growth, slow down the tumor cell death rate, and promote cancer cells genomic variability leading to increased tumorigenesis (2). Similar to the TGF-β mechanism, hypoxia affects NK cell cytotoxicity by decreasing the expression of activating receptors NKp46 and CD16. Hypoxia can also induce overexpression of the adenosine nucleotidase CD73, upregulate checkpoint molecules such as PD-L1, and down-regulate the activating ligand MICA on tumor cells (51).
All of these survival TME mechanisms are challenges that autologous and allogeneic NK cells must overcome to be successful as an immunotherapy option. Lately, one alternative explored for therapeutic purposes is to target and boost NK cells that were previously activated to awaken their memory functions (52, 53).
6 Memory-like NK cell production
The consensus is that innate memory forms in three stages (1). The cell expansion phase upon an activation event (encounter of the antigen/activator by naïve cells) (2); the contraction phase, when cells that return to baseline function undergo apoptosis; and survival cells enter (3) the memory phase, which produces enhanced response of remembering a prior-faced stimulation. However, NK cell memory differs from a classic adaptive memory, varying accordingly to the employed activation stimulus with unique functional and molecular characteristics (25, 86). Current strategies to produce a large number of NK cells are in development, with the hope of allowing the potential treatment of many patients from a single source, as observed in Figure 3 (24). Several studies demonstrated that the generation of ML NK and memory-like responses could be achieved under specific circumstances such as hapten-specific exposure, viral infection, feeder cell exposure, and cytokine activation (25, 53).
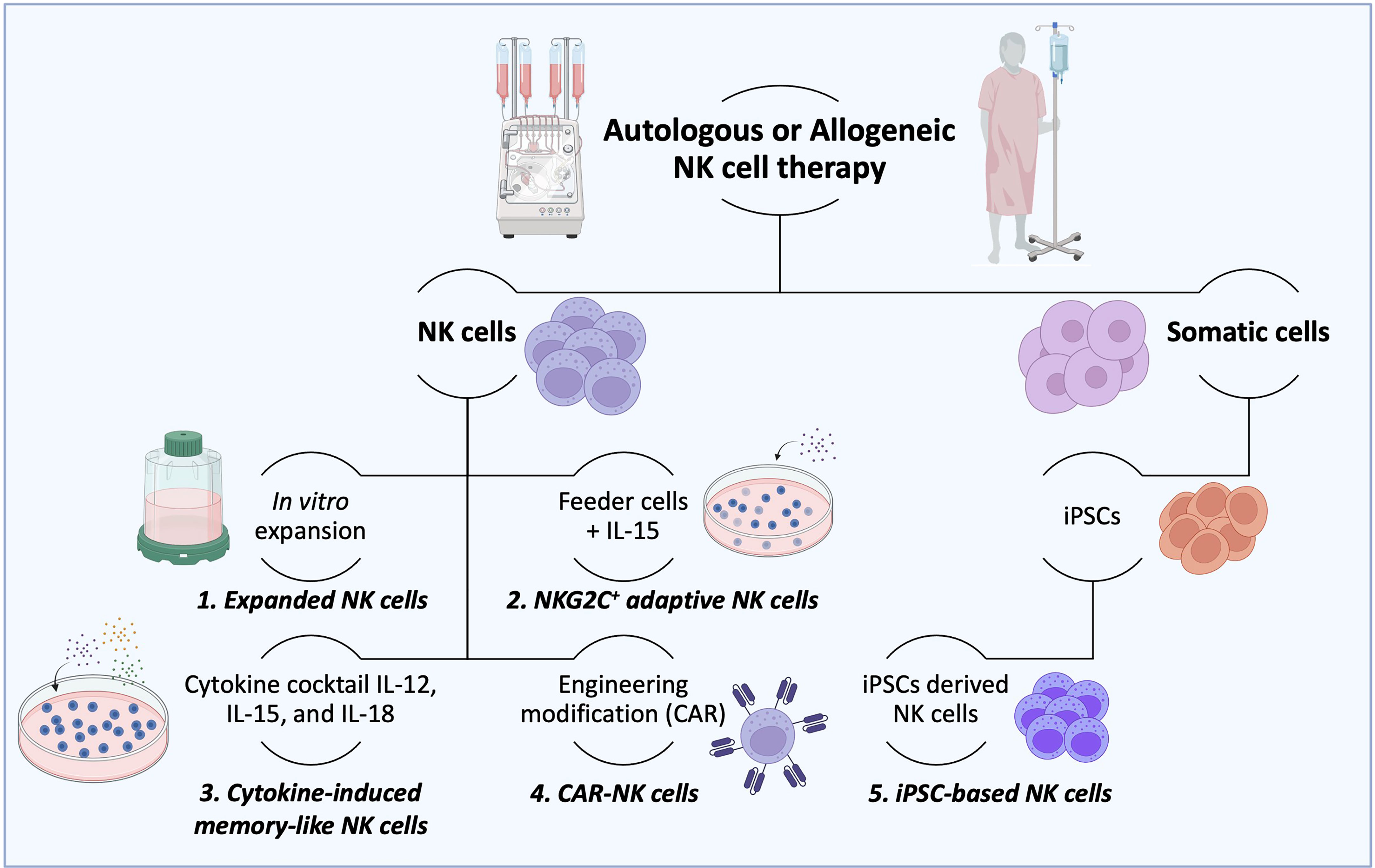
Figure 3 Production of therapeutic NK cells. For autologous or allogeneic clinical purposes, a considerable number of therapeutic NK cells are needed to destroy cancer cells effectively. To this end, several ways to obtain the desired number of therapeutic NK cells have been established. (1) After isolation, NK cells could be mass expanded in vitro in culture flasks, reactors, and bags until the needed cell number is achieved. (2) Irradiated feeder cells (to suppress their expansion) in the presence of IL-15 are employed to stimulate the NK cell activation and expansion. (3) The combination of cytokines such as IL-12, IL-15, and IL-18 is used to expand NK cells and generate memory functions (memory-like NK cells). (4) NK cells can also be potentiated by engineering modifications with CAR constructs to overcome their current limitations. (5) More recently, induced pluripotent stem cells (iPSCs) have been considered an advantageous source of NK and CAR-NK cells over peripheral and cord blood due to their usage in both autologous and allogeneic settings. (6) The IL-2-dependent immortalized NK-92 cell line is another type of NK cell source that possesses features of immune cell types and has been used in clinical trials.
ML NK cells obtained by antigen-specific exposure, were first described by O’Leary et al. when studying hapten-induced contact hypersensitivity (CHS) response in mice lacking T cells and B cells (87). Hapten-induced CHS is a typical example of adaptive immunity, where the epithelial surface is exposed to molecules that chemically modify proteins. Those “haptenated molecules” becomes foreign antigens triggering the formation of hapten-specific and long-lived immune memory cells. In this study, it was found that the Ly49C-I+ NK subpopulation localized in the donor’s liver exhibited responses specifically to DNFB (2,4-dinitro-1- fluorobenzene) and OXA (oxazolone) haptens that persisted for at least 4 weeks (87). After that, it was demonstrated that the CXCR6 (C-X-C chemokine receptor type 6) chemokine receptor plays a critical role in NK memory response to haptens and viruses. CXCR6 was present on hepatic NK cells and was responsible for their persistence and homeostasis, but not for antigen recognition. Although the CXCR6 receptor expression is not limited to the liver, only hepatic NK cells mediated the memory response (62). Another study of classical CHS protocols with immunocompromised mice that lacked T and B cells, but not NK cells, demonstrated that liver NK cells induced specific memory responses to haptens. This behavior was confirmed upon transfer in CD3-deficient mice, confirming the existence of “memory-like” NK cells (88). Consequently, it was established that DNFB-induced liver NK cells were potent effector cells that appeared within 1 hour after immunization. Authors suggested that hapten-specific effector NK cells are pre-existing in naive hosts, ready to exert inflammatory responses immediately after antigen contact (89). Antigen-specific NK cell memory was also found in primate species (rhesus macaques) after SIVmac251- and SHIV162P3 infections and AD26 vaccination, suggesting a potential use in vaccines against HIV-1 and pathogens (55). Likewise, it was found that specific subpopulations infected with human cytomegalovirus (HCMV) possess an NKG2C+ NK cell subset that exhibits clonal-like expansion and partially mimics an anti-viral adaptive response (64). Another study showed that NK cells exposed to therapeutic antibodies such as AFM13 (CD30/CD16A) produced more IFN-γ and showed increased cytotoxicity upon restimulation with lymphoma tumor cells, indicating memory-like functionality (65).
The use of feeder cells such as PBMCs, Jurkat T cells, K562 leukemia cells, and Wilms tumor HFWT have also been explored to produce ML NK cells. This method shows promising survival and growth results even for large-scale NK cell expansion. Feeder cells can promote cell growth by triggering the extracellular secretions of growth factors, detoxification of the culture media, synthesis of extracellular matrix proteins, or improved cell attachment (90). For example, NK cells expanded in a co-culture setting with irradiated (40 Gy) 721.221-AEH cells showed a high killing capability against acute lymphoblastic leukemia cells, that could be easily scaled up into a GMP-compliant process. Interestingly, even when the cytotoxicity response was optimal, less than 30% of the cell population showed CD107a marker upon degranulation, which was explained by the different killing mechanisms that natural killer cells could perform in response to the target (91). Jurkat T cells sub-line KL-1 induced a 100-fold NK cell expansion with almost 90% purity of NK cells from PBMCs, with potent antitumor capability. The in vitro and in vivo tumor-lytic capacity was associated with the expression of natural cytotoxicity receptors (NKp30, NKp44), activation receptors (NKG2D, DNAM-1), and adhesion molecules (CD11a, ICAM-1) (92). Authors also suggested that the positive results using feeder cells could be enhanced using genetically modified cells. Indeed K562-mb15-4-1BBL cells (K562 cells modified to express membrane-bound IL-15 and 4-1BBL) are already used in several clinical trials of allogeneic NK cells (93). Membrane-bound cytokines IL-2, IL-15, or IL-21 in K562 feeder cells, lacking HLA, but expressing costimulatory molecules, can be used for effective NK cell ex vivo expansion. Concurrently, Min et al. combined the use of irradiated autologous PBMCs and the monoclonal antibody OKT3 in the presence of IL-2 to large-scale NK cell expansion for 3 weeks and evaluated the effect of a freeze/thaw cycle. NK cells reached approximately 15,000-fold expansion, and while their viability was slightly reduced after thawing, their cytotoxicity and cytokine secretion against hepatocellular carcinoma cells were not affected (94). Rezaeifard et al., as part of an autologous phase I clinical trial, executed a pre-clinical NK cell enrichment employing anti-CD56 and anti-CD3 in PBMCs with the addition of IL-2. After two weeks, these expanded NK cells (CD3-CD16+/-CD56+) presented highly lytic properties against K562 cells, reaching a 510-fold average with a strong expression of NKG2D and CD16 (95).
Another method to support the activation, expansion, and survival of NK cells is the addition of cytokines to the culture medium, traditionally using IL-2 and, most recently, IL-15 (72, 96). The rationale behind using cytokines to stimulate NK cells is to increase cell sensitivity by targeting different costimulatory molecules and activating receptors (97). Various combinations and permutations of the cytokines IL-12, IL-15, IL-18, and IL-21 were shown to produce ML NK cells for pre-clinical and clinical studies. Terren et al. studied different combinations of IL-12, IL-15, and IL-18 cytokines to generate human cytokine-induced memory-like (CIML) NK cells. They found that IL-15 significantly contributes to NK cell cytotoxicity, but combining the three cytokines induced a synergistic effect that granted the cells the best polyfunctional profile. That profile includes proper degranulation and production of multiple cytokines and chemokines such as INF-γ, TNF-α, and CCL3 (C-C motif chemokine ligand 3) (98). Romee et al. developed the first-in-human phase I clinical trial of CIML NK cells with low dose IL-2, demonstrating high functional responses enhancing INF-γ production and cytotoxicity. This study showed promising results, with good clinical response in five of nine treated myeloid leukemia patients (72). Shapiro et al. performed a phase I clinical trial infusing allogeneic CIML NK cells in acute AML patients after lymphodepletion and subcutaneous IL-2 dosage. NK cells stimulated for 12-16 hours with the cytokine cocktail (IL-12, IL-15, and IL-18) and infused at a range of 5 to 10 million cells/kg demonstrated a rapid in vivo expansion and long-term persistence after infusion (59). Other research focused on the large-scale production of ML NK cells through cytokine stimulation. Liu et al. established a strategy to develop a high-efficient large-scale generation of NK cells from peripheral blood. In that study, NK cells stimulated with IL-2, IL-15, and IL-18 showed prolonged persistence, high cytotoxicity against K562 cells, and high levels of activating molecules such as CD16 and NKG2D (99). While feeder cells have previously been employed for large-scale expansion (100), the use of cytokine-induced stimulation could avoid the problems that come along with the use of feeder cells. Some examples of those problems include irradiation for inactivation, product contamination by feeder cells, and overall safety risk that can limit the large-scale production of NK cells for clinical purposes (99).
7 Enhancing NK cells potency against cancer
Current NK cells-mediated cancer immunotherapy produced encouraging results against hematological malignancies and solid tumors (1, 4). Still, these results can likely be potentiated in combination with other cell lines (101), nanoparticles (102–104), as well as chemotherapy and radiotherapy (105).
The combination of NK cell therapy with cytotoxic T cells was demonstrated to kill more cells than the sum of individual treatments. Notably, these 2 cell types are the most widely used lymphocytes in cancer immunotherapy, which aims to target cancer cell populations through opposites mechanisms, suggesting the existence of cytotoxic synergies (37, 101). Additionally, IFN-γ production of NK cells in the TME upregulates MHC-I expression by tumor cells, increasing activatory targets for cytotoxic T cells (106, 107). Therefore, combining NK cells with T cells is a potential mechanism to achieve tumor regression. For example, Zhang et al. studied this combined human lymphocyte treatment against primary tumor tissue of lung cancer in vitro and in vivo. They found that allogeneic NK cells were more cytolytic against cancer cells than autologous NK cells; activated T cells primarily impacted MHC class I-positive cancer cells, while NK cells focused on MHC class I-negative cancer cells (101). This result could be correlated to the fact that CD8+ T cell response and expansion increased with the presence of NK cells in the B16F10 melanoma (2). Friedmann et al. used melanoma cells as a cancer model to study the combinatorial cytotoxicity of NK cells and cytotoxic T lymphocytes (CTL). They found that after 24 hours of co-culture using high effector-to-target (E: T) ratios, melanoma cells were fully lysed, while at lower E: T ratios (simulating human cancer conditions), a significant number of melanoma cells survived and became more resistant to further NK cell exposure. Interestingly, this detrimental effect could be reversed if the initial NK exposure is followed by CTL exposure, which the authors suggest could be due to the release of exogenous antigens (108). The engineering modification of NK cells in combination with CAR-T cells has also been explored to enhance the potency of cell therapy treatments, showing that NK cells support the anticancer CAR-T cell response (109). In combination with CD19 CAR-T cells, cord blood-derived NK cells induced an enhanced antitumor efficacy of CAR-T cells against multiple myeloma, by facilitating their earlier activation and improving their migration to the tumor cells (109). It was also observed that in malignant mesothelioma patients, the physiological levels of CD56Bright and CD56Dim NK cell subset populations were abnormal. However, the addition of an anti-cytotoxic T lymphocyte-associated antigen 4 (CTLA4) checkpoint restored those NK-subset levels, activity, and killing capability (110).
NK cells have also been associated with other immune cells than T cell lymphocytes to enhance the cytotoxic response against cancer. In human melanoma, stimulatory dendritic cell abundance was found to be related to the intra-tumoral expression of the gene encoding the cytokine FLT3LG, which is principally produced by NK cells. Also, Böttcher et al. found that the interplay between NK cells and conventional type 1 dendritic cells (cDC1) has numerous therapeutic implications within the TME (111). For instance, intra-tumoral transcripts CCL5, XCL1, and XCL2 closely correlate with NK cells and cDC1 gene signatures that were also associated with patient survival (111). This cell interaction suggested an excellent tool for the success of cell immunotherapy since NK cells are required to promote the optimal cytotoxicity response of T cells through the recruitment of dendritic cells in the tumor site (21, 107, 111, 112).
Nanoparticles have also been explored to enhance NK cell therapy in multiple research groups (103). Magnetic (113), chitosan (114), lipid nanoparticles (115), liposomal polymeric gel (116), and PLGA microspheres (117) are examples of nanoparticle substrates employed in previous studies to augment NK cell immunotherapy against several types of cancers. Namely, Adjei et al. used manganese dioxide nanoparticles as a vehicle to deliver siRNA (small interfering RNA) for the NK cell TGF‐β receptor 2, which resulted in the restoration of NK cell activity against lung cancer cells (102). Most recently, Biber et al. developed a non-viral lipid nanoparticle to encapsulate and deliver small interfering RNAs (siRNAs) to overcome current obstacles in NK cell-based immunotherapies. They showed that their nanoparticles targeted NK cells in vivo, silencing their intrinsic inhibitory checkpoints and triggering NK cell activity to kill tumors (118).
Currently, the combination of chemotherapy with NK cell therapy is tested in multiple cancer clinical trials (see Table 1). This combination can be a synergistic strategy to improve outcomes in order to facilitate effector cell trafficking, cell infiltration, NK cell-mediated cytotoxicity, lysis, and cancer antigen release (105, 119). Several chemo-drugs also promote the expression of NK cell activating receptors and their ligands expression on tumor cells, and enhance the secretion of selected chemokines, making cancer cells more vulnerable to NK cell cytotoxicity (37, 105). A vast list of available drugs can be considered to potentiate the NK cell therapy results. Antitumor antibiotics, antimetabolic and alkylating agents, plant alkaloids, proteasome, histone deacetylase, and tyrosine kinase inhibitors are types of drugs commonly used in chemotherapy (105). That potentiation has been demonstrated with different types of cancer. For example, Bae et al. demonstrated the safety of a high-dose autologous NK cell therapy combined with chemotherapy in a phase I clinical trial. They employed 4 cycles of hepatic arterial infusion chemotherapy (HAIC) of 5- fluorouracil (750 mg/m2) and cisplatin (25 mg/m2) (48). Additionally, it was confirmed that combining NK cells with alkylating agents sensitizes melanoma cells, allowing the control of tumor growth. Using dacarbazine in melanoma patients showed an increased expression of the NKp46 receptor (120), and a higher NK cell activation and cytolytic activity (121). Another study revealed that using lymphodepletion chemotherapy before injecting NK cells in patients with AML combined with a low dose of IL-2, increased IL-15 production and promoted NK cell persistence and expansion (42). The chemotherapy regimen included cyclophosphamide and methylprednisolone, and fludarabine (42). Other types of cancer, including ovarian cancer (122) and advanced colon carcinoma (123), have also been treated with NK cells and chemotherapy combinations. Moreover, NK cells combined with chemoradiotherapy induced long-term tumor control against metastatic nasopharyngeal carcinoma (124). An intriguing avenue to enhance the cytotoxicity of NK cells in the TME is to use artificial engagers that both support NK function and lower the TME capacity for immune inhibition while lowering the potentials for side-effects. For example, a fusion complex combining an IL-15 agonist coupled with a TGF-β molecular sink showed increased infiltration of both NK and CD8+ cytotoxic cells and lower tumor burden in a syngeneic mouse B16F10 melanoma model (125).
Other combinatorial studies have also demonstrated additional avenues for potentiating the anti-cancer effect of NK-based therapies. For instance, it was reported that the combined treatment of NK cells with oncolytic viruses (OV, therapeutically beneficial viruses that have the ability to replicate and kill cancer cells) enhanced the cytotoxicity of OV and induced NK cell infiltration (107, 126–128). Another study found that using a checkpoint antibody blocking TIGIT, an inhibitory cell surface receptor, reversed the NK cell exhaustion and enhanced NK cell cytotoxicity, inhibiting in vivo colon, breast, and melanoma tumor growth (129).
In addition to the potentiated NK cell therapy combinations already mentioned, engineered modifications of NK cells is a fast-growing field. Further details are mentioned in the next section.
8 Engineered modification of NK cells
The genetic modification of NK cells to express CAR (Chimeric Antigen Receptor) constructs has recently gained significant attention, due to the success met using this technology with T cells. CAR were first described in 1987 (31) and are bioengineered cell receptors coded by “chimeric genes composed of immunoglobulin (Ig)-derived variable (V) regions and T-cell receptor (TCR)-derived constant (C) regions”, allowing for the specific of cancer cells while activating the engaged immune cell. Since NK possess several activation receptors, this concept was ideal to be used for the design of CAR-NK cells (130). These modifications intend to overcome the limitations of non-modified NK cells when facing tumor cells with genetic or epigenetic variations that bypass the NK immunological surveillance (51, 58). CAR-NK cells can be produced from multiple sources (such as peripheral blood, cord blood, iPSCs, and NK-92 cell line), adopting the basic methods borrowed from CAR-T cell generation (131). The CAR molecule, a synthetic hybrid antigen receptor (132), can redirect the NK cell-killing capability to a specific antigen target. The main characteristics of CAR-NK cells versus traditional NK cells have been summarized in Table 4.
To date, NK cells have been engineered and modified with CARs in order to overcome challenges such as the limited persistence after infusion without cytokine support, slow trafficking to tumor beds, and overcoming the immunosuppressive TME (131). Similar to CAR-T cells, CAR-NK cell functionality was initially tested against hematological malignancies. In a pre-clinical study, the combination of CIML NK cells expressing an anti-CD19 specific CAR was tested to enhance the response rate in NK-resistant cancers. Results demonstrated an increased functional response (degranulation, IFN-γ production, and specific killing) against B cell lymphomas by CD19-CIML NK cells compared with CD19-NK, CD33-NK, and ML NK cells (133). These results underline the great potential that CAR-NK cell therapy could offer compared with non-modified NK cells. In addition to CD19, there are almost 50 other surface target antigens, including CD5 (134), EGFR or EGFRvIII (135–138), HER-2 (139–142), NKG2D (143–146), and GD2 (147, 148) that have been considered to enhance CAR-NK cell responses (149).
Additionally, it is known that NK cells are characterized by a short-lived persistence in the body after infusion in the absence of cytokine support, approximately 14 days, which limits the efficacy of NK cell immunotherapy. That limitation was intended to be overcome by the genetic modification of NK cells with transgenes encoding cytokines IL-2 or IL-15 either for secretion or membrane expression. This strategy yielded the suppression of inhibitory signals of the TME as well as enhancing the NK cell killing capability in several studies (131, 150, 151). Also, to modulate the CAR-NK cell functionality, the expression of cytotoxic ligands and chemokine receptors such as CXCR4, EGFRvIII, and the combined expression of FRα and DR4/5 were included in the engineering modification design (137, 152, 153). The NK cell metabolism, cell homing, and tumor infiltration were also enhanced with engineering modification (154). For example, to improve the metabolism within the TME, maintaining the levels of cMyc signaling with kinase glycogen synthase kinase-3 (GSK3) inhibitors (155, 156), as well as the deletion of hypoxia-inducible factor 1a (HIF1a) (157) were a target of modifications. Cell homing is another critical factor considered in the NK cell therapy success, which was suggested to be targeted with different mechanisms (154). For example, lymphodepletion complemented with cytokine support (158), expression of the CXCR4 gene (152), pharmacologic combination (139, 159), and others were employed, having encouraging results in cell homing that also impacted the cell trafficking and tumor infiltration.
The first clinical trial of CAR-NK cell treatment focused on CD19 as a target (see Table 5), which showed a 73% positive response rate without evidence of GvHD, development of cytokine release syndrome, or neurotoxicity. Infused CD19-CAR-NK cells also demonstrated expansion and persistence for at least 12 months at low levels (160). After that trial, other phase I and II clinical studies were performed in USA and China. Some of these studies focused on hematological malignancies such as lymphomas (13 trials) and multiple myeloma (NCT05182073, NCT03940833). Others focused on solid tumors (131), including colorectal (NCT05213195, NCT05248048), glioblastoma and neuroblastoma (NCT03383978, NCT02439788), prostate (NCT03692663), ovarian (NCT03692637), metastatic gastric or head and neck cancer (NCT04847466), and pancreatic (NCT03941457) cancer. Some of these studies were withdrawn because of funding issues (NCT03579927) and methodology improvement (NCT02439788), while others have unknown statuses (NCT03940833, NCT03941457, NCT03692637, NCT03415100, NCT03940820).
Nevertheless, before translating the use of CAR-NK cells in clinical trials, some challenges must be addressed. Their limited persistence/expansion and their difficulty in being genetically modified must be addressed (152, 154, 161, 162). Some examples of successful genetic modification include the work of Tang et al., which reached over 90% transduction efficiency of CD33-CAR construct in the NK-92 cell line (163). But, NK cells are generally heterogeneous and have proven to be more challenging to expand and engineer in vitro by transduction, transfection, or nucleofection than T cells. During and after the engineering process, they show high sensitivity to apoptosis and low gene expression levels, which implies instability of the product and that the cost of obtaining therapeutic CAR-NK cells could be significantly higher than other alternatives (164).
The CAR transduction techniques employed for NK are primarily based on viral transduction and non-viral mediated transfection. The latter is the preferred method as it will induce fewer toxicities (149). The most successful current techniques yield a rapid, high, but transient expression (electroporation) or a sustained but low gene expression (viral vectors) (165). Despite the limitations, the most successful non-viral gene delivery technique in NK cells is the rapid transient expression by electroporation (166). Electroporation yields highly efficient T-cell transfection, but that result was not observed at a similar level with NK cells (154). For example, in terms of engineered modification of blood-derived NK cells, lipid-based and electroporation methods typically result in less than 5% transfection; retrovirus vector-based yield a 27-50% efficiency, and lentivirus-based transfection only yields a 20-40% efficiency (167). Nevertheless, even when viral transduction produces better results, they are associated with decreased cell viability, NK cell apoptosis, and reduced efficacy (154). To date, some in vitro and in vivo attempts at genome editing techniques aim to design suitable CAR-NK cell products (168). For example, the NK cells modification with a chimeric receptor consisting of activating receptor NKG2D (143), DNAX-activation protein 12 (DAP12) with CD3ζ (169), and NKG2D-ζ (170) were used to improve NK cell cytotoxic activity. But even when CAR-NK cells are designed to enhance further the results obtained with normal NK cells, the use of engineering NK cells does not always appear to produce optimal results. For example, Bachiller et al. studied the therapeutic effect of CAR-T cells with CAR-NK cells and NK cells combinations against non-Hodgkin’s lymphoma (NHL) and multiple myeloma (MM) using cord blood as an NK cell source. The effectiveness of CAR-NK cells was observed only when using higher doses of cells, with a rapid loss of activity over time compared with CAR-T cells, while the control NK cells produced a synergistic anticancer CAR-T cell response (109).
9 Challenges and future perspectives
The principal feature of NK cells is their natural ability to protect the host by recognizing, directing, and executing the rapid lysis of cancerous, stressed, and viral-infected cells without dependent priming (33–35). However, several challenges overshadow the effective NK cell-mediated cancer immunotherapy. Those challenges, including short-term persistence, sensitivity, immunosuppression of the TME, and clinical-grade ex vivo expansion, should be addressed for a successful cell therapy treatment (24). The potential for NK therapies, demonstrated in pre-clinical studies, has clearly supported sustained research but outside of the field of hematological cancers, very little to no progress have been reported in the clinic for any type of NK therapies against solid tumors with mostly due to a lack of results or early discontinuation of the trial. These discontinuations are in majority dues to either off-target toxicities or a lack of response. While it seems that NK-based immunotherapies are of very little benefit to the patient, it is worth looking at the potential for optimizations that might be responsible for some of these early lackluster results.
NK cells have a highly sensitive response to external stresses, such as cryopreservation. The wellness, stability, and proper therapeutic responses of NK cells throughout the entire manufacturing process are highly dependent on maintaining stable, stress-free physiological settings (171). NK cells are very susceptible to stress stimuli, particularly cryopreservation, which is reflected in their loss of viability and cytotoxic function after thawing (131, 167): the average rate of cryopreserved NK cell survival is less than 50%. Even when the cytotoxicity of viable cells can be recovered overnight in the presence of IL-2, recovering the pre-freezing cell numbers could take several days of in vitro expansion. Similarly, exposure to low temperatures, such as overnight refrigeration, affects cytokine-activated NK cells, as observed by a reduction of their cytotoxicity, even when their viability is not affected (167). In a recent study, cryopreserved NK cells demonstrated a 5.6-fold reduced killing activity against K562 tumor cells embedded in a 3D collagen matrix compared with fresh NK cells. This behavior may be explained by two mechanisms affected by cryopreservation: (1) loss of NK cell activation through cleavage of CD16, and (2) reduction of NK cell motility, preventing contact with target cells (172). Other factors affected by cryo-injury in NK cells are cell metabolic activity, cytokine activation, cell culture density, and persistence (131). Since this particular issue negatively impacts (through the use of cryopreservation, long-term storage, and shipping process) general immunotherapies before infusion, it is essential to intensify efforts and optimize procedures to reduce the NK cell dysfunction after thawing. Preserving the viability and cytotoxic function post-thaw is critical in a clinical setting to ensure a high consistency and quality of the products. Due to these issues observed after thawing during the in vitro culture, the direct infusion of freshly expanded NK cells “warm chain” may be advantageous. Another advantage to such “warm chain” would be the capability to add other immune fractions that could benefit from minimal handling and that can work in synergy with NK cells. γδT cells or CD8+ cytotoxic cells would be prime candidates for such combinations and could be obtained for the same leukapheresis product, for example. However, the realization of such personalized, combinatorial immune cell therapy would require the creation of a structure allowing the harvesting, isolation, expansion, re-invigoration and formulation of the immune cells at the point of care.
Regarding engineering modification, challenges such as the difficulty of transferring genetic material into NK cells in pre-clinical studies, selecting the most suitable and effective technique to accomplish the GMP grades should be a priority before scaling up the CAR-NK cell production. One of the primary issues with CAR-NK stem from either their lack of efficacy, or too much off-target cytotoxicity when applied in the clinic setting. These issues have been intensively scrutinized and researchers have been considering many variables to optimize therapies (149, 173, 174): (i) different cell sources such as immortalized cell lines (NK-92 or YT cell lines, that require irradiation before injection), peripheral NKs, stem-cell, umbilical or placenta-derived NKs, and ML-NKs, (ii) CAR design and use of the correct promoter, (iii) transduction methods such as retroviruses or electroporation. While these approaches have yielded encouraging pre-clinical results, it is still unclear as to how this can translate into the clinical setting. As underlined in Table 5, the lack of positive progress report or the discontinuation for current clinical trial are a testament of the difficulties to port these therapies to the clinic. Additionally, engineering of NK cell carries an additional production cost compared with non-engineered NK cells, and in some cases, the therapeutic response does not have the best cost-effectiveness ratio. Nevertheless, using NK cells over T cells as a vehicle to carry CAR expression has several advantages, such as the different NK cell sources and the donor–patient compatibility, which could reduce the average therapy manufacturing cost. Using iPSCs to obtain NK cells is a promising alternative to producing a significant number of therapeutic NK cells and then genetically modifying them. They are considered an advantageous source of NK and CAR-NK cells over peripheral and cord blood due to their versatility, homogeneity, high proliferation capacity, and usage in both autologous and allogeneic settings (4). This alternative is currently addressed in several research groups. For example, in 2019, Fate Therapeutics Company published clinical data about an iPSC-derived CAR-NK cell therapy against B Cell malignancies. In combination with rituximab, this therapy demonstrated prolonged in vivo cell survival and enhanced tumor-killing capacity compared with rituximab therapy alone (4, 175). Moreover, against solid tumors (e.g., ovarian cancer), iPSC-derived CAR-NK also showed enhanced anti-tumor activity, inhibition of tumor growth, and prolonged survival compared with iPSC-NK cells, T-CAR-iPSC-NK cells, or PB-NK cells (176). These results underline the great potential that iPSCs-derived CAR-NK cells could offer for cancer immunotherapy. One intriguing possibility would be to combine the high cytotoxic and survival of ML-NK cells with the increased specificity and targeting conferred by CAR modifications. However, while this combination might yield significant clinical effect, it is unclear how the downside of CAR modifications would ultimately negatively affect the outcome.
While significant technical progress has been made to enhance the properties of NK cells, most of the progress has sought to correct issues that stem from the allogeneic and bioengineered nature of current NK cell therapies: short persistence of the product and off-target cytotoxicity are instances described in this review. Additionally, we reviewed evidence here that at least some cell anergies could be linked to a decentralized process, introducing detrimental inefficiencies such as product lead time, shipping, and freezing/thawing. Typically, a cell therapy designed by a biopharmaceutical entity would have to be produced and shipped to a clinical site specialized in infusion, where the processes specific to the product needs to be executed. These processes are extremely specific, from shipping/receiving to handling and dosing, and in return require extreme expertise from the staff involved. Meanwhile, the possible complications linked to novel NK cell therapies will always require the availability of specialized post-care monitoring and care by a hospital system or a primary oncologist. However, both the medical and regulatory fields have been slow to recognize NK cells and their unique abilities (and cell therapy as a whole) as a new class of therapies, so it follows that only highly trained and specialized staff should be monitoring and managing patients for these early therapies and their specific needs. The fragmentation of the process, and lack of specialization of the involved structures (especially the hospital system), are clear hurdle to the obtention of stable and interpretable results. The slow progress of NK cell immunotherapy, and the de-facto focus on allogeneic use of the cells that can only exacerbate possible cytotoxicities, may be linked to the inefficiencies listed above. These problems could be addressed with a new clinical paradigm, where the patient’s cells could be directly harvested, expanded, and administered at the point of care, removing inefficiencies and lowering the cost of therapy (177), which remains one of the highest obstacles to the universal adoption of cellular immunotherapies. Patient’s safety and deviations would consequently be minimized and improve trials success and patient care. Even if this model could counteract to some of the downsides of CAR-NK production, it would still not answer the lackluster clinical observations from CAR-NK therapies in the solid tumor space. Autologous therapies and patients would be the main beneficiaries of this model, since the need for an “off-the-shelf” product would become obsolete as safe and longer-lasting therapies can be created on-site directly from the patient and become a prime solution for cancer immune cell therapies.
Author contributions
RW contributed to the conception, design, revision and approval of this manuscript. GL-V contributed to the writing of the sections and the design of figures in the manuscript. MT-L, RD and JP contributed to the manuscript revisions and approved the submitted version. All authors contributed to the article and approved the submitted version.
Funding
This research was funded by the NSF Non-Academic Research Internships for Graduate Students (INTERN) Supplemental Funding Opportunity through the Center for Cell Manufacturing Technologies (CMaT) with award number EEC-1648035 and by Carolina BioOncology Institute (CBOI).
Conflict of interest
JP is the founder/owner of CBOI and BioCytics. RW and RD are employees of CBOI and BioCytics.
This study received funding from CBOI/BioCytics. The funder had the following involvement with the study: manuscript revisions and approval.
The remaining authors declare that the research was conducted in the absence of any commercial or financial relationships that could be construed as a potential conflict of interest.
Publisher’s note
All claims expressed in this article are solely those of the authors and do not necessarily represent those of their affiliated organizations, or those of the publisher, the editors and the reviewers. Any product that may be evaluated in this article, or claim that may be made by its manufacturer, is not guaranteed or endorsed by the publisher.
References
1. Shimasaki N, Jain A, Campana D. NK cells for cancer immunotherapy. Nat Rev Drug Discovery (2020) 19:200–18. doi: 10.1038/s41573-019-0052-1
2. Raskov H, Orhan A, Salanti A, Gaggar S, Gögenur I. Natural killer cells in cancer and cancer immunotherapy. Cancer Lett (2021) 520:233–42. doi: 10.1016/j.canlet.2021.07.032
3. Marcenaro E, Notarangelo LD, Orange JS, Vivier E. Editorial: NK cell subsets in health and disease: new developments. Front Immunol (2017) 8:1363. doi: 10.3389/fimmu.2017.01363
4. Chu J, Gao F, Yan M, Zhao S, Yan Z, Shi B, et al. Natural killer cells: a promising immunotherapy for cancer. J Transl Med (2022) 20:240. doi: 10.1186/s12967-022-03437-0
5. Herberman RB, Nunn ME, Lavrin DH. Natural cytotoxic reactivity of mouse lymphoid cells against syngeneic and allogeneic tumors. i. distribution of reactivity and specificity. Int J Cancer (1975) 16:216–29. doi: 10.1002/ijc.2910160204
6. Kiessling R, Klein E, Wigzell H. "Natural" killer cells in the mouse. i. cytotoxic cells with specificity for mouse moloney leukemia cells. specificity and distribution according to genotype. Eur J Immunol (1975) 5:112–7. doi: 10.1002/eji.1830050208
7. Guillerey C, Huntington ND, Smyth MJ. Targeting natural killer cells in cancer immunotherapy. Nat Immunol (2016) 17:1025–36. doi: 10.1038/ni.3518
8. Shaver KA, Croom-Perez TJ, Copik AJ. Natural killer cells: the linchpin for successful cancer immunotherapy. Front Immunol (2021) 12:679117. doi: 10.3389/fimmu.2021.679117
9. Fabbri M. Natural killer cell–derived vesicular miRNAs: a new anticancer approach? Cancer Res (2020) 80:17–22. doi: 10.1158/0008-5472.CAN-19-1450
10. Jong AY, Wu C-H, Li J, Sun J, Fabbri M, Wayne AS, et al. Large-Scale isolation and cytotoxicity of extracellular vesicles derived from activated human natural killer cells. J Extracell Vesicles (2017) 6:1294368. doi: 10.1080/20013078.2017.1294368
11. Chiossone L, Dumas PY, Vienne M, Vivier E. Natural killer cells and other innate lymphoid cells in cancer. Nat Rev Immunol (2018) 18:671–88. doi: 10.1038/s41577-018-0061-z
12. Brien KLO, Finlay DK. Immunometabolism and natural killer cell responses. Nat Rev Immunol (2019) 19:282–290. doi: 10.1038/s41577-019-0139-2
13. Wu J, Edberg JC, Redecha PB, Bansal V, Guyre PM, Coleman K, et al. Novel polymorphism of fc RIIIa (CD16) and autoimmune disease a novel polymorphism of fc RIIIa (CD16) alters receptor function and predisposes to autoimmune disease. J Clin Invest (1997) 100:1059–70. doi: 10.1172/JCI119616
14. Jensen H, Potempa M, Gotthardt D, Lanier L. Cutting edge: IL-2 – induced expression of the amino acid transporters SLC1A5 and CD98 is a prerequisite for NKG2D-mediated activation of human NK cells. J Immunol (2017) 199:1967–72. doi: 10.4049/jimmunol.1700497
15. Keating SE, Zaiatz-bittencourt V, Roisín M, Keane C, Brennan K, Finlay DK, et al. Metabolic reprogramming supports IFN- γ production by CD56 bright NK cells. J Immunol (2016) 196:2552–2560. doi: 10.4049/jimmunol.1501783
16. Guerra N, Dulphy N, Watzl C, Zingoni A, Molfetta R, Fionda C, et al. NKG2D and its ligands: “One for all, all for one”. Artic 476 1 Immunol (2018) 9:476. doi: 10.3389/fimmu.2018.00476
17. Cifaldi L, Doria M, Cotugno N, Zicari S, Cancrini C, Palma P, et al. Molecular sciences DNAM-1 activating receptor and its ligands: how do viruses affect the NK cell-mediated immune surveillance during the various phases of infection? Int J Mol Sci (2019) 20: doi: 10.3390/ijms20153715
18. Brandt CS, Baratin M, Yi EC, Kennedy J, Gao Z, Fox B, et al. The B7 family member B7-H6 is a tumor cell ligand for the activating natural killer cell receptor NKp30 in humans. J Exp Med (2009) 206:1495–503. doi: 10.1084/JEM.20090681
19. Pedroza-Pacheco I, Madrigal A, Saudemont A. Interaction between natural killer cells and regulatory T cells: perspectives for immunotherapy. Cell Mol Immunol (2013) 10:222–9. doi: 10.1038/cmi.2013.2
20. Jung D, Baek YS, Lee IJ, Kim KY, Jang H, Hwang S, et al. Ex vivo expanded allogeneic natural killer cells have potent cytolytic activity against cancer cells through different receptor-ligand interactions. J Exp Clin Cancer Res (2021) 40:333. doi: 10.1186/s13046-021-02089-0
21. Huntington ND, Cursons J, Rautela J. The cancer–natural killer cell immunity cycle. Nat Rev Cancer (2020) 20:437–54. doi: 10.1038/s41568-020-0272-z
22. Kumar S. Natural killer cell cytotoxicity and its regulation by inhibitory receptors. Immunology (2018) 154:383–93. doi: 10.1111/imm.12921
23. Miller JS, Geller MA. Use of allogeneic NK cells for cancer immunotherapy. Immunotherapy (2011) 3:1445–59. doi: 10.2217/imt.11.131
24. Kennedy PR, Felices M, Miller JS. Challenges to the broad application of allogeneic natural killer cell immunotherapy of cancer. Stem Cell Res Ther (2022) 13:165. doi: 10.1186/s13287-022-02769-4
25. Gang M, Wong P, Berrien-Elliott MM, Fehniger TA. Memory-like natural killer cells for cancer immunotherapy. Semin Hematol (2020) 57:185. doi: 10.1053/J.SEMINHEMATOL.2020.11.003
26. Marin ND, Krasnick BA, Becker-Hapak M, Conant L, Goedegebuure SP, Berrien-Elliott MM, et al. Memory-like differentiation enhances NK cell responses to melanoma. Clin Cancer Res (2021) 27:4859–69. doi: 10.1158/1078-0432.CCR-21-0851
27. Nahi H, Chrobok M, Meinke S, Gran C, Marquardt N, Afram G, et al. Autologous NK cells as consolidation therapy following stem cell transplantation in multiple myeloma. Cell Rep Med (2022) 3:100508. doi: 10.1016/j.xcrm.2022.100508
28. Judge SJ, Murphy WJ, Canter RJ. Characterizing the dysfunctional NK cell: assessing the clinical relevance of exhaustion, anergy, and senescence. Front Cell Infect Microbiol (2020) 10:49. doi: 10.3389/fcimb.2020.00049
29. Abel AM, Yang C, Thakar MS, Malarkannan S. Natural killer cells: development, maturation, and clinical utilization. Front Immunol (2018) 9:1869. doi: 10.3389/fimmu.2018.01869
30. Basar R, Daher M, Rezvani K. Next-generation cell therapies: the emerging role of CAR-NK cells. Blood Adv (2020) 4:5868–76. doi: 10.1182/bloodadvances.2020002547
31. Lamb MG, Rangarajan HG, Tullius BP, Lee DA. Natural killer cell therapy for hematologic malignancies: successes, challenges, and the future. Stem Cell Res Ther (2021) 12:1–19. doi: 10.1186/S13287-021-02277-X
32. Koch J, Steinle A, Watzl C, Mandelboim O. Activating natural cytotoxicity receptors of natural killer cells in cancer and infection. Trends Immunol (2013) 34:182–91. doi: 10.1016/j.it.2013.01.003
33. Vacca P, Munari E, Tumino N, Moretta F, Pietra G, Vitale M, et al. Human natural killer cells and other innate lymphoid cells in cancer: friends or foes? Immunol Lett (2018) 201:14–9. doi: 10.1016/j.imlet.2018.11.004
34. Wilk AJ, Blish CA. Diversification of human NK cells: lessons from deep profiling. J Leukoc Biol (2018) 103:629–41. doi: 10.1002/JLB.6RI0917-390R
35. Terrén I, Orrantia A, Astarloa-Pando G, Amarilla-Irusta A, Zenarruzabeitia O, Borrego F. Cytokine-induced memory-like NK cells: from the basics to clinical applications. Front Immunol (2022) 13:884648. doi: 10.3389/fimmu.2022.884648
36. Buddingh EP, Schilham MW, Ruslan SEN, Berghuis D, Szuhai K, Suurmond J, et al. Chemotherapy-resistant osteosarcoma is highly susceptible to IL-15-activated allogeneic and autologous NK cells. Cancer Immunol Immunother (2011) 60:575–86. doi: 10.1007/s00262-010-0965-3
37. Hu W, Wang G, Huang D, Sui M, Xu Y. Cancer immunotherapy based on natural killer cells: current progress and new opportunities. Front Immunol (2019) 10:1205. doi: 10.3389/fimmu.2019.01205
38. Shah NN, Baird K, Delbrook CP, Fleisher TA, Kohler ME, Rampertaap S, et al. Acute GVHD in patients receiving IL-15/4-1BBL activated NK cells following T-cell-depleted stem cell transplantation. Blood (2015) 125:784–92. doi: 10.1182/blood-2014-07-592881
39. Lorenz E, Congdon C, Uphoff D. Modification of Acute Irradiation Injury in Mice and Guinea-Pigs by Bone Marrow Injections. Radiology (1952) 58:863–77. doi: 10.1148/58.6.863
40. Furukawa Y, Hamano Y, Shirane S, Kinoshita S, Azusawa Y, Ando J, et al. Advances in allogeneic cancer cell therapy and future perspectives on “Off-the-Shelf” T cell therapy using iPSC technology and gene editing. Cells (2022) 11:269. doi: 10.3390/CELLS11020269
41. Beilhack A, Schulz S, Baker J, Beilhack GF, Wieland CB, Herman EI, et al. In vivo Analyses of early events in acute graft-versus-host disease reveal sequential infiltration of T-cell subsets. Blood (2005) 106:1113–22. doi: 10.1182/blood-2005-02-0509
42. Miller JS, Soignier Y, Panoskaltsis-Mortari A, McNearney SA, Yun GH, Fautsch SK, et al. Successful adoptive transfer and in vivo expansion of human haploidentical NK cells in patients with cancer. Blood (2005) 105:3051–7. doi: 10.1182/blood-2004-07-2974
43. Bachanova V, Cooley S, Defor TE, Verneris MR, Zhang B, McKenna DH, et al. Clearance of acute myeloid leukemia by haploidentical natural killer cells is improved using IL-2 diphtheria toxin fusion protein. Blood (2014) 123:3855–63. doi: 10.1182/blood-2013-10-532531
44. Shapiro RM, Romee R. Autologous cellular therapy for myeloma: giving ex vivo expanded NK cells their due. Cell Rep Med (2022) 3:100537. doi: 10.1016/j.xcrm.2022.100537
45. Du N, Guo F, Wang Y, Cui J. Nk cell therapy: a rising star in cancer treatment. Cancers (Basel) (2021) 13:4129. doi: 10.3390/cancers13164129
46. Burns LJ, Weisdorf DJ, DeFor TE, Vesole DH, Repka TL, Blazar BR, et al. IL-2-based immunotherapy after authologous transplantation for lymphoma and breast cancer induces immune activation and cytokine release: a phase I/II trial. Bone Marrow Transplant (2003) 32:177–86. doi: 10.1038/sj.bmt.1704086
47. Sakamoto N, Ishikawa T, Kokura S, Okayama T, Oka K, Ideno M, et al. Phase I clinical trial of autologous NK cell therapy using novel expansion method in patients with advanced digestive cancer. J Transl Med (2015) 13:1–13. doi: 10.1186/s12967-015-0632-8
48. Bae WK, Lee BC, Kim HJ, Lee JJ, Chung IJ, Cho SB, et al. A phase I study of locoregional high-dose autologous natural killer cell therapy with hepatic arterial infusion chemotherapy in patients with locally advanced hepatocellular carcinoma. Front Immunol (2022) 13:879452. doi: 10.3389/fimmu.2022.879452
49. Khatua S, Cooper LJN, Sandberg DI, Ketonen L, Johnson JM, Rytting ME, et al. Phase I study of intraventricular infusions of autologous ex vivo expanded NK cells in children with recurrent medulloblastoma and ependymoma. Neuro Oncol (2020) 22:1214–25. doi: 10.1093/neuonc/noaa047
50. Vahedi F, Nham T, Poznanski SM, Chew MV, Shenouda MM, Lee D, et al. Ex vivo expanded human NK cells survive and proliferate in humanized mice with autologous human immune cells. Sci Rep (2017) 7:1–6. doi: 10.1038/s41598-017-12223-8
51. Gunduz M, Atilla PA, Atilla E. New orders to an old soldier: optimizing NK cells for adoptive immunotherapy in hematology. Biomedicines (2021) 9:1201. doi: 10.3390/biomedicines9091201
52. O’Sullivan TE, Sun JC, Lanier LL. Natural killer cell memory. Immunity (2015) 43:634–45. doi: 10.1016/j.immuni.2015.09.013
53. Cerwenka A, Lanier LL. Natural killer cell memory in infection, inflammation and cancer. Nat Rev Immunol (2016) 16:112–23. doi: 10.1038/nri.2015.9
54. Foley B, Cooley S, Verneris MR, Curtsinger J, Luo X, Waller EK, et al. Human cytomegalovirus (CMV)-induced memory-like NKG2C + NK cells are transplantable and expand In Vivo in response to recipient CMV antigen. J Immunol (2012) 189:5082–8. doi: 10.4049/jimmunol.1201964
55. Reeves RK, Li H, Jost S, Blass E, Li H, Schafer JL, et al. Antigen-specific NK cell memory in rhesus macaques. Nat Immunol (2015) 16:927–32. doi: 10.1038/ni.3227
56. Romee R, Schneider SE, Leong JW, Chase JM, Keppel CR, Sullivan RP, et al. Cytokine activation induces human memory-like NK cells. Blood (2012) 120:4751–4760. doi: 10.1182/blood-2012-04-419283
57. van Helden MJG, Zaiss DMW, Sijts AJAM. CCR2 defines a distinct population of NK cells and mediates their migration during influenza virus infection in mice. PLoS One (2012) 7:e52027. doi: 10.1371/journal.pone.0052027
58. Zhang L, Liu M, Yang S, Wang J, Feng X, Han Z. Natural killer cells: of-the-shelf cytotherapy for cancer immunosurveillance. Am J Cancer Res (2021) 11:1770–91.
59. Shapiro RM, Birch GC, Hu G, Vergara Cadavid J, Nikiforow S, Baginska J, et al. Expansion, persistence, and efficacy of donor memory-like NK cells infused for posttransplant relapse. J Clin Invest (2022) 132. doi: 10.1172/jci154334
60. Berrien-Elliott MM, Cashen AF, Cubitt CC, Neal CC, Wong P, Wagner JA, et al. Multidimensional analyses of donor memory-like NK cells reveal new associations with response after adoptive immunotherapy for leukemia. Cancer Discovery (2020) 10:1854–72. doi: 10.1158/2159-8290.CD-20-0312
61. Wang D, Malarkannan S. Transcriptional regulation of natural killer cell development and functions. Cancers (Basel) (2020) 12:1–33. doi: 10.3390/cancers12061591
62. Paust S, Gill HS, Wang BZ, Flynn MP, Moseman EA, Senman B, et al. Critical role for the chemokine receptor CXCR6 in NK cell-mediated antigen-specific memory of haptens and viruses. Nat Immunol (2010) 11:1127–35. doi: 10.1038/ni.1953
63. Zhang LH, Shin JH, Haggadone MD, Sunwoo JB. The aryl hydrocarbon receptor is required for the maintenance of liver-resident natural killer cells. J Exp Med (2016) 213:2249–57. doi: 10.1084/jem.20151998
64. Hammer Q, Rückert T, Borst EM, Dunst J, Haubner A, Durek P, et al. Peptide-specific recognition of human cytomegalovirus strains controls adaptive natural killer cells article. Nat Immunol (2018) 19:453–63. doi: 10.1038/s41590-018-0082-6
65. Pahl JHW, Koch J, Gotz JJ, Arnold A, Reusch U, Gantke T, et al. Cd16a activation of nk cells promotes nk cell proliferation and memory-like cytotoxicity against cancer cells. Cancer Immunol Res (2018) 6:517–27. doi: 10.1158/2326-6066.CIR-17-0550
66. Beaulieu AM, Zawislak CL, Nakayama T, Sun JC. The transcription factor Zbtb32 controls the proliferative burst of virus-specific natural killer cells responding to infection. Nat Immunol (2014) 15:546–53. doi: 10.1038/ni.2876
67. Adams NM, Lau CM, Fan X, Rapp M, Geary CD, Weizman OE, et al. Transcription factor IRF8 orchestrates the adaptive natural killer cell response. Immunity (2018) 48:1172–1182.e6. doi: 10.1016/j.immuni.2018.04.018
68. Wendt K, Wilk E, Buyny S, Schmidt RE, Jacobs R. Interleukin-21 differentially affects human natural killer cell subsets. Immunology (2007) 122:486–95. doi: 10.1111/j.1365-2567.2007.02675.x
69. Beaulieu AM. Transcriptional and epigenetic regulation of memory NK cell responses. Immunol Rev (2021) 300:125–33. doi: 10.1111/imr.12947
70. Reina-Ortiz C, Giraldos D, Azaceta G, Palomera L, Marzo I, Naval J, et al. Harnessing the potential of NK cell-based immunotherapies against multiple myeloma. Cells (2022) 11:392. doi: 10.3390/cells11030392
71. Boieri M, Ulvmoen A, Sudworth A, Lendrem C, Collin M, Dickinson AM, et al. IL-12, IL-15, and IL-18 pre-activated NK cells target resistant T cell acute lymphoblastic leukemia and delay leukemia development in vivo. Oncoimmunology (2017) 6:e1274478. doi: 10.1080/2162402X.2016.1274478
72. Romee R, Rosario M, Berrien-Elliott MM, Wagner JA, Jewell BA, Schappe T, et al. Cytokine-induced memory-like natural killer cells exhibit enhanced responses against myeloid leukemia. Sci Transl Med (2016) 8:357ra123. doi: 10.1126/scitranslmed.aaf2341
73. Bonanni V, Antonangeli F, Santoni A, Bernardini G. Targeting of CXCR3 improves anti-myeloma efficacy of adoptively transferred activated natural killer cells. J Immunother Cancer (2019) 7:1–16. doi: 10.1186/s40425-019-0751-5
74. Uppendahl LD, Felices M, Bendzick L, Ryan C, Kodal B, Hinderlie P, et al. Cytokine-induced memory-like natural killer cells have enhanced function, proliferation, and in vivo expansion against ovarian cancer cells. Gynecol Oncol (2019) 153:149–57. doi: 10.1016/j.ygyno.2019.01.006
75. Zhuang L, Fulton RJ, Rettman P, Sayan AE, Coad J, Al-Shamkhani A, et al. Activity of IL-12/15/18 primed natural killer cells against hepatocellular carcinoma. Hepatol Int (2019) 13:75–83. doi: 10.1007/s12072-018-9909-3
76. Tanzi M, Consonni M, Falco M, Ferulli F, Montini E, Pasi A, et al. Cytokine-induced memory-like nk cells with high reactivity against acute leukemia blasts and solid tumor cells suitable for adoptive immunotherapy approaches. Cancers (Basel) (2021) 13. doi: 10.3390/cancers13071577
77. Stoiber D, Witalisz-siepracka A, Klein K, Zda B. The multifaceted role of STAT3 in NK-cell tumor surveillance. Front Immunol (2022) 13:1–12. doi: 10.3389/fimmu.2022.947568
78. Melaiu O, Lucarini V, Cifaldi L, Fruci D. Influence of the tumor microenvironment on NK cell function in solid tumors. Front Immunol (2020) 10:3038. doi: 10.3389/fimmu.2019.03038
79. Liu H, Wang S, Xin J, Wang J, Yao C, Zhang Z. Role of NKG2D and its ligands in cancer immunotherapy. Am J Cancer Res (2019) 9:2064–78.
80. Raulet DH, Guerra N. Oncogenic stress sensed by the immune system: role of natural killer cell receptors. Nat Rev Immunol (2009) 9:568–80. doi: 10.1038/nri2604
81. Close HJ, Stead LF, Nsengimana J, Reilly KA, Droop A, Wurdak H, et al. Expression profiling of single cells and patient cohorts identifies multiple immunosuppressive pathways and an altered NK cell phenotype in glioblastoma. Clin Exp Immunol (2020) 200:33–44. doi: 10.1111/cei.13403
82. Viel S, Marçais A, Souza-Fonseca Guimaraes F, Loftus R, Rabilloud J, Grau M, et al. TGF-b inhibits the activation and functions of NK cells by repressing the mTOR pathway. Sci Signal (2016) 9:ra19. doi: 10.1126/scisignal.aad1884
83. St-Pierre F, Bhatia S, Chandra S, Bottino C, Curti A. Cancers harnessing natural killer cells in cancer immunotherapy: a review of mechanisms and novel therapies. Cancers (Basel) (2021) 13: doi: 10.3390/cancers13081988
84. Brand A, Singer K, Koehl GE, Kolitzus M, Schoenhammer G, Thiel A, et al. LDHA-associated lactic acid production blunts tumor immunosurveillance by T and NK cells. Cell Metab (2016) 24:657–71. doi: 10.1016/j.cmet.2016.08.011
85. Zheng X, Qian Y, Fu B, Jiao D, Jiang Y, Chen P, et al. Mitochondrial fragmentation limits NK cell-based tumor immunosurveillance. Nat Immunol (2019) 20:1656–67. doi: 10.1038/s41590-019-0511-1
86. Min-Oo G, Kamimura Y, Hendricks DW, Nabekura T, Lanier LL. Natural killer cells: walking three paths down memory lane. Trends Immunol (2013) 34:251–8. doi: 10.1016/j.it.2013.02.005
87. O’Leary JG, Goodarzi M, Drayton DL, von Andrian UH. T Cell- and b cell-independent adaptive immunity mediated by natural killer cells. Nat Immunol (2006) 7:507–16. doi: 10.1038/ni1332
88. Rouzaire P, Luci C, Blasco E, Bienvenu J, Walzer T, Nicolas JF, et al. Natural killer cells and T cells induce different types ofskin reactions during recall responses to haptens. Eur J Immunol (2012) 42:80–8. doi: 10.1002/eji.201141820
89. Majewska-Szczepanik M, Paust S, von Andrian UH, Askenase PW, Szczepanik M. Natural killer cell-mediated contact sensitivity develops rapidly and depends on interferon-α, interferon-γ and interleukin-12. Immunology (2013) 140:98–110. doi: 10.1111/imm.12120
90. Llames S, García-Pérez E, Meana Á, Larcher F, Del Río M. Feeder layer cell actions and applications. Tissue Eng - Part B Rev (2015) 21:345–53. doi: 10.1089/ten.teb.2014.0547
91. Liu LL, Béziat V, Oei VYS, Pfefferle A, Schaffer M, Lehmann S, et al. Ex vivo expanded adaptive NK cells effectively kill primary acute lymphoblastic leukemia cells. Cancer Immunol Res (2017) 5:654–65. doi: 10.1158/2326-6066.CIR-16-0296
92. Lim SA, Kim TJ, Lee JE, Sonn CH, Kim K, Kim J, et al. Ex vivo expansion of highly cytotoxic human NK cells by cocultivation with irradiated tumor cells for adoptive immunotherapy. Cancer Res (2013) 73:2598–607. doi: 10.1158/0008-5472.CAN-12-2893
93. Maddineni S, Silberstein JL, Sunwoo JB. Emerging NK cell therapies for cancer and the promise of next generation engineering of iPSC-derived NK cells. J Immunother Cancer (2022) 10:e004693. doi: 10.1136/jitc-2022-004693
94. Min B, Choi H, Her JH, Jung MY, Kim HJ, Jung MY, et al. Optimization of large-scale expansion and cryopreservation of human natural killer cells for anti-tumor therapy. Immune Netw (2018) 18:1–13. doi: 10.4110/in.2018.18.e31
95. Rezaeifard S, Heike Y, Masuyama JI, Rezvani A, Vojdani R, Erfani N. Autologous natural killer cell-enrichment for immune cell therapy: preclinical setting phase, Shiraz experience. Iran J Allergy Asthma Immunol (2021) 20:233–43. doi: 10.18502/ijaai.v20i2.6056
96. Cooley S, He F, Bachanova V, Vercellotti GM, DeFor TE, Curtsinger JM, et al. First-in-human trial of rhIL-15 and haploidentical natural killer cell therapy for advanced acute myeloid leukemia. Blood Adv (2019) 3:1970–80. doi: 10.1182/bloodadvances.2018028332
97. Kundu S, Gurney M, O’Dwyer M. Generating natural killer cells for adoptive transfer: expanding horizons. Cytotherapy (2021) 23:559–66. doi: 10.1016/j.jcyt.2020.12.002
98. Terrén I, Mikelez I, Odriozola I, Gredilla A, González J, Orrantia A, et al. Implication of interleukin-12/15/18 and ruxolitinib in the phenotype, proliferation, and polyfunctionality of human cytokine-preactivated natural killer cells. Front Immunol (2018) 9:737. doi: 10.3389/fimmu.2018.00737
99. Liu M, Meng Y, Zhang L, Han Z, Feng X. High-efficient generation of natural killer cells from peripheral blood with preferable cell vitality and enhanced cytotoxicity by combination of IL-2, IL-15 and IL-18. Biochem Biophys Res Commun (2021) 534:149–56. doi: 10.1016/j.bbrc.2020.12.012
100. Lapteva N, Durett AG, Sun J, Rollins LA, Huye LL, Fang J, et al. Large-Scale ex vivo expansion and characterization of natural killer cells for clinical applications. Cytotherapy (2012) 14:1131–43. doi: 10.3109/14653249.2012.700767
101. Zhang Y, Decker W, Wang Y, Zimmerman M, Wang Z. Inhibition of cancer cell immune evasion by combined application of cytotoxic T-lymphocytes and natural killer cells. J Transl Res (2018) 2:12–19.
102. Adjei IM, Jordan J, Tu N, Le TT, Kandell W, Wei S, et al. Functional recovery of natural killer cell activity by nanoparticle-mediated delivery of transforming growth factor beta 2 small interfering RNA. J Interdiscip Nanomedicine (2019) 4:98–112. doi: 10.1002/jin2.63
103. Kim KS, Kim DH, Kim DH. Recent advances to augment NK cell cancer immunotherapy using nanoparticles. Pharmaceutics (2021) 13:1–14. doi: 10.3390/pharmaceutics13040525
104. Mikelez-Alonso I, Magadán S, González-Fernández Á, Borrego F. Natural killer (NK) cell-based immunotherapies and the many faces of NK cell memory: a look into how nanoparticles enhance NK cell activity. Adv Drug Delivery Rev (2021) 176:113860. doi: 10.1016/j.addr.2021.113860
105. Cifaldi L, Locatelli F, Marasco E, Moretta L, Pistoia V. Boosting natural killer cell-based immunotherapy with anticancer drugs: a perspective. Trends Mol Med (2017) 23:1156–75. doi: 10.1016/j.molmed.2017.10.002
106. Dighe AS, Richards E, Old LJ, Schreiber RD. Enhanced in vivo growth and resistance to rejection of tumor cells expressing dominant negative IFNγ receptors. Immunity (1994) 1:447–56. doi: 10.1016/1074-7613(94)90087-6
107. Warricker F, Khakoo SI, Blunt MD. The role of NK cells in oncolytic viral therapy: a focus on hepatocellular carcinoma. J Transl Genet Genomics (2021) 5:304–322. doi: 10.20517/jtgg.2021.27
108. Friedmann KS, Knörck A, Cappello S, Hoxha C, Schwär G, Iden S, et al. Interdependence of CTL and NK cell cytotoxicity against melanoma cells. bioRxiv (2020) 2020:06.14.150672. doi: 10.1101/2020.06.14.150672
109. Bachiller M, Perez-Amill L, Battram AM, Carné SC, Najjar A, Verhoeyen E, et al. NK cells enhance CAR-T cell antitumor efficacy by enhancing immune/tumor cells cluster formation and improving CAR-T cell fitness. J Immunother Cancer (2021) 9. doi: 10.1136/jitc-2021-002866
110. Sottile R, Tannazi M, Johansson MH, Cristiani CM, Calabró L, Ventura V, et al. NK- and T-cell subsets in malignant mesothelioma patients: baseline pattern and changes in the context of anti-CTLA-4 therapy. Int J Cancer (2019) 145:2238–48. doi: 10.1002/ijc.32363
111. Böttcher JP, Bonavita E, Chakravarty P, Blees H, Cabeza-Cabrerizo M, Sammicheli S, et al. NK cells stimulate recruitment of cDC1 into the tumor microenvironment promoting cancer immune control. Cell (2018) 172:1022–1037.e14. doi: 10.1016/j.cell.2018.01.004
112. Barry KC, Hsu J, Broz ML, Cueto FJ, Binnewies M, Combes AJ, et al. A natural killer–dendritic cell axis defines checkpoint therapy–responsive tumor microenvironments. Nat Med (2018) 24:1178–91. doi: 10.1038/s41591-018-0085-8
113. Jang ES, Shin JH, Ren G, Park MJ, Cheng K, Chen X, et al. The manipulation of natural killer cells to target tumor sites using magnetic nanoparticles. Biomaterials (2012) 33:5584–92. doi: 10.1016/j.biomaterials.2012.04.041
114. Tan L, Han S, Ding S, Xiao W, Ding Y, Qian L, et al. Chitosan nanoparticle-based delivery of fused NKG2D-IL-21 gene suppresses colon cancer growth in mice. Int J Nanomedicine (2017) 12:3095–107. doi: 10.2147/IJN.S128032
115. Atukorale PU, Raghunathan SP, Raguveer V, Moon TJ, Zheng C, Bielecki PA, et al. Nanoparticle encapsulation of synergistic immune agonists enables systemic codelivery to tumor sites and IFNβ-driven antitumor immunity. Cancer Res (2019) 79:5394–406. doi: 10.1158/0008-5472.CAN-19-0381
116. Park J, Wrzesinski SH, Stern E, Look M, Criscione J, Ragheb R, et al. Combination delivery of TGF-β inhibitor and IL-2 by nanoscale liposomal polymeric gels enhances tumour immunotherapy. Nat Mater (2012) 11:895–905. doi: 10.1038/nmat3355
117. Park W, Gordon AC, Cho S, Huang X, Harris KR, Larson AC, et al. Immunomodulatory magnetic microspheres for augmenting tumor-specific infiltration of natural killer (NK) cells. ACS Appl Mater Interfaces (2017) 9:13819–24. doi: 10.1021/acsami.7b02258
118. Biber G, Sabag B, Raiff A, Ben-Shmuel A, Puthenveetil A, Benichou JIC, et al. Modulation of intrinsic inhibitory checkpoints using nano-carriers to unleash NK cell activity. EMBO Mol Med (2022) 14:1–18. doi: 10.15252/emmm.202114073
119. Garofalo C, De Marco C, Cristiani CM. NK cells in the tumor microenvironment as new potential players mediating chemotherapy effects in metastatic melanoma. Front Oncol (2021) 11:754541. doi: 10.3389/fonc.2021.754541
120. Fregni G, Perier A, Pittari G, Jacobelli S, Sastre X, Gervois N, et al. Unique functional status of natural killer cells in metastatic stage IV melanoma patients and its modulation by chemotherapy. Clin Cancer Res (2011) 17:2628–37. doi: 10.1158/1078-0432.CCR-10-2084
121. Mignot G, Hervieu A, Vabres P, Dalac S, Jeudy G, Bel B, et al. Prospective study of the evolution of blood lymphoid immune parameters during dacarbazine chemotherapy in metastatic and locally advanced melanoma patients. PLoS One (2014) 9:1–11. doi: 10.1371/journal.pone.0105907
122. Van der Meer JMR, de Jonge PKJD, van der Waart AB, Geerlings AC, Moonen JP, Brummelman J, et al. CD34+ progenitor-derived NK cell and gemcitabine combination therapy increases killing of ovarian cancer cells in NOD/SCID/IL2Rgnull mice. Oncoimmunology (2021) 10:1981049. doi: 10.1080/2162402X.2021.1981049
123. Li L, Li W, Wang C, Yan X, Wang Y, Niu C, et al. Adoptive transfer of natural killer cells in combination with chemotherapy improves outcomes of patients with locally advanced colon carcinoma. Cytotherapy (2018) 20:134–48. doi: 10.1016/j.jcyt.2017.09.009
124. Jin YY, Yang WZ, Zou S, Sun ZY, Wu CT, Yang ZY. Chemoradiotherapy combined with NK cell transfer in a patient with recurrent and metastatic nasopharyngeal carcinoma inducing long-term tumor control: a case report. Med (Baltimore) (2020) 99:e22785. doi: 10.1097/MD.0000000000022785
125. Liu B, Zhu X, Kong L, Wang M, Spanoudis C, Chaturvedi P, et al. Bifunctional TGF-β trap/IL-15 protein complex elicits potent NK cell and CD8+ T cell immunity against solid tumors. Mol Ther (2021) 29:2949–62. doi: 10.1016/j.ymthe.2021.06.001
126. Rintoul JL, Lemay CG, Tai LH, Stanford MM, Falls TJ, De Souza CT, et al. ORFV: a novel oncolytic and immune stimulating parapoxvirus therapeutic. Mol Ther (2012) 20:1148–57. doi: 10.1038/mt.2011.301
127. Miyamoto S, Inoue H, Nakamura T, Yamada M, Sakamoto C, Urata Y, et al. Coxsackievirus B3 is an oncolytic virus with immunostimulatory properties that is active against lung adenocarcinoma. Cancer Res (2012) 72:2609–21. doi: 10.1158/0008-5472.CAN-11-3185
128. Samson A, Bentham MJ, Scott K, Nuovo G, Bloy A, Appleton E, et al. Oncolytic reovirus as a combined antiviral and anti-tumour agent for the treatment of liver cancer. Gut (2018) 67:562–73. doi: 10.1136/gutjnl-2016-312009
129. Zhang Q, Bi J, Zheng X, Chen Y, Wang H, Wu W, et al. Blockade of the checkpoint receptor TIGIT prevents NK cell exhaustion and elicits potent anti-tumor immunity. Nat Immunol (2018) 19:723–32. doi: 10.1038/s41590-018-0132-0
130. Khawar MB, Sun H. CAR-NK cells: from natural basis to design for kill. Front Immunol (2021) 12:707542/BIBTEX. doi: 10.3389/FIMMU.2021.707542/BIBTEX
131. Daher M, Rezvani K. Outlook for new car-based therapies with a focus on car nk cells: what lies beyond car-engineered t cells in the race against cancer. Cancer Discovery (2021) 11:45–58. doi: 10.1158/2159-8290.CD-20-0556
132. Marofi F, Abdul-Rasheed OF, Rahman HS, Budi HS, Jalil AT, Yumashev AV, et al. CAR-NK cell in cancer immunotherapy; a promising frontier. Cancer Sci (2021) 112:3427–36. doi: 10.1111/cas.14993
133. Gang M, Marin ND, Wong P, Neal CC, Marsala L, Foster M, et al. CAR-modified memory-like NK cells exhibit potent responses to NK-resistant lymphomas. Blood (2020) 136:2308–18. doi: 10.1182/BLOOD.2020006619
134. Xu Y, Liu Q, Zhong M, Wang Z, Chen Z, Zhang Y, et al. 2B4 costimulatory domain enhancing cytotoxic ability of anti-CD5 chimeric antigen receptor engineered natural killer cells against T cell malignancies. J Hematol Oncol (2019) 12:49. doi: 10.1186/s13045-019-0732-7
135. Genßler S, Burger MC, Zhang C, Oelsner S, Mildenberger I, Wagner M, et al. Dual targeting of glioblastoma with chimeric antigen receptor-engineered natural killer cells overcomes heterogeneity of target antigen expression and enhances antitumor activity and survival. Oncoimmunology (2016) 5:e1119354. doi: 10.1080/2162402X.2015.1119354
136. Murakami T, Nakazawa T, Natsume A, Nishimura F, Nakamura M, Matsuda R, et al. Novel human NK cell line carrying CAR targeting EGFRvIII induces antitumor effects in glioblastoma cells. Anticancer Res (2018) 38:5049–56. doi: 10.21873/anticanres.12824
137. Müller N, Michen S, Tietze S, Töpfer K, Schulte A, Lamszus K, et al. Engineering NK cells modified with an EGFRvIII-specific chimeric antigen receptor to overexpress CXCR4 improves immunotherapy of CXCL12/SDF-1α-secreting glioblastoma. J Immunother (2015) 38:197–210. doi: 10.1097/CJI.0000000000000082
138. Liu Y, Zhou Y, Huang KH, Fang X, Li Y, Wang F, et al. Targeting epidermal growth factor-overexpressing triple-negative breast cancer by natural killer cells expressing a specific chimeric antigen receptor. Cell Prolif (2020) 53:1–12. doi: 10.1111/cpr.12858
139. Wu X, Huang S. HER2-specific chimeric antigen receptor-engineered natural killer cells combined with apatinib for the treatment of gastric cancer. Bull Cancer (2019) 106:946–58. doi: 10.1016/j.bulcan.2019.03.012
140. Schönfeld K, Sahm C, Zhang C, Naundorf S, Brendel C, Odendahl M, et al. Selective inhibition of tumor growth by clonal NK cells expressing an ErbB2/HER2-specific chimeric antigen receptor. Mol Ther (2015) 23:330–8. doi: 10.1038/mt.2014.219
141. Kruschinski A, Moosmann A, Poschke I, Norell H, Chmielewski M, Seliger B, et al. Engineering antigen-specific primary human NK cells against HER-2 positive carcinomas. Proc Natl Acad Sci U.S.A. (2008) 105:17481–6. doi: 10.1073/pnas.0804788105
142. Zhang C, Burger MC, Jennewein L, Genßler S, Schönfeld K, Zeiner P, et al. ErbB2/HER2-specific NK cells for targeted therapy of glioblastoma. J Natl Cancer Inst (2016) 108:1–12. doi: 10.1093/jnci/djv375
143. Chang YH, Connolly J, Shimasaki N, Mimura K, Kono K, Campana D. A chimeric receptor with NKG2D specificity enhances natural killer cell activation and killing of tumor cells. Cancer Res (2013) 73:1777–86. doi: 10.1158/0008-5472.CAN-12-3558
144. Wang Z, Guo L, Song Y, Zhang Y, Lin D, Hu B, et al. Augmented anti-tumor activity of NK-92 cells expressing chimeric receptors of TGF-βR II and NKG2D. Cancer Immunol Immunother (2017) 66:537–48. doi: 10.1007/s00262-017-1959-1
145. Wang J, Lupo KB, Chambers AM, Matosevic S. Purinergic targeting enhances immunotherapy of CD73+ solid tumors with piggyBac-engineered chimeric antigen receptor natural killer cells 11 medical and health sciences 1107 immunology. J Immunother Cancer (2018) 6:1–14. doi: 10.1186/s40425-018-0441-8
146. Lu C, Guo C, Chen H, Zhang H, Zhi L, Lv T, et al. A novel chimeric PD1-NKG2D-41BB receptor enhances antitumor activity of NK92 cells against human lung cancer H1299 cells by triggering pyroptosis. Mol Immunol (2020) 122:200–6. doi: 10.1016/j.molimm.2020.04.016
147. Kailayangiri S, Altvater B, Spurny C, Jamitzky S, Schelhaas S, Jacobs AH, et al. Targeting ewing sarcoma with activated and GD2-specific chimeric antigen receptor-engineered human nk cells induces upregulation of immune-inhibitory HLA-G. Oncoimmunology (2017) 6:e1250050. doi: 10.1080/2162402X.2016.1250050
148. Esser R, Müller T, Stefes D, Kloess S, Seidel D, Gillies SD, et al. NK cells engineered to express a GD 2-specific antigen receptor display built-in ADCC-like activity against tumour cells of neuroectodermal origin. J Cell Mol Med (2012) 16:569–81. doi: 10.1111/j.1582-4934.2011.01343.x
149. Zhang L, Meng Y, Feng X, Han Z. CAR-NK cells for cancer immunotherapy: from bench to bedside. biomark Res (2022) 10:1–19. doi: 10.1186/S40364-022-00364-6
150. Liu E, Tong Y, Dotti G, Shaim H, Savoldo B, Mukherjee M, et al. Cord blood NK cells engineered to express IL-15 and a CD19-targeted CAR show long-term persistence and potent antitumor activity. Leukemia (2018) 32:520–31. doi: 10.1038/leu.2017.226
151. Matosevic S. Viral and nonviral engineering of natural killer cells as emerging adoptive cancer immunotherapies. J Immunol Res (2018) 2018. doi: 10.1155/2018/4054815
152. Jamali A, Hadjati J, Madjd Z, Mirzaei HR, Thalheimer FB, Agarwal S, et al. Highly efficient generation of transgenically augmented CAR NK cells overexpressing CXCR4. Front Immunol (2020) 11:2028. doi: 10.3389/fimmu.2020.02028
153. Lee YE, Ju A, Choi HW, Kim JC, Kim EEK, Kim TS, et al. Rationally designed redirection of natural killer cells anchoring a cytotoxic ligand for pancreatic cancer treatment. J Control Release (2020) 326:310–23. doi: 10.1016/j.jconrel.2020.07.016
154. Karvouni M, Vidal-Manrique M, Lundqvist A, Alici E. Engineered NK cells against cancer and their potential applications beyond. Front Immunol (2022) 13:825979. doi: 10.3389/fimmu.2022.825979
155. Parameswaran R, Ramakrishnan P, Moreton SA, Xia Z, Hou Y, Lee DA, et al. Repression of GSK3 restores NK cell cytotoxicity in AML patients. Nat Commun (2016) 7:1–11. doi: 10.1038/ncomms11154
156. Cichocki F, Valamehr B, Bjordahl R, Zhang B, Rezner B, Rogers P, et al. GSK3 inhibition drives maturation of NK cells and enhances their antitumor activity. Cancer Res (2017) 77:5664–75. doi: 10.1158/0008-5472.CAN-17-0799
157. Choi C, Finlay DK. Optimising NK cell metabolism to increase the efficacy of cancer immunotherapy. Stem Cell Res Ther (2021) 12:1–10. doi: 10.1186/s13287-021-02377-8
158. Miller JS, Rooney CM, Curtsinger J, McElmurry R, McCullar V, Verneris MR, et al. Expansion and homing of adoptively transferred human natural killer cells in immunodeficient mice varies with product preparation and InVivo cytokine administration: implications for clinical therapy. Biol Blood Marrow Transplant (2014) 20:1252–7. doi: 10.1016/j.bbmt.2014.05.004
159. Zhang Q, Zhang H, Ding J, Liu H, Li H, Li H, et al. Combination therapy with EpCAM-CAR-NK-92 cells and regorafenib against human colorectal cancer models. J Immunol Res (2018) 2018:4263520. doi: 10.1155/2018/4263520
160. Liu E, Marin D, Banerjee P, Macapinlac HA, Thompson P, Basar R, et al. Use of CAR-transduced natural killer cells in CD19-positive lymphoid tumors. N Engl J Med (2020) 382:545–53. doi: 10.1056/nejmoa1910607
161. Sutlu T, Nyström S, Gilljam M, Stellan B, Applequist SE, Alici E. Inhibition of intracellular antiviral defense mechanisms augments lentiviral transduction of human natural killer cells: implications for gene therapy. Hum Gene Ther (2012) 23:1090–100. doi: 10.1089/hum.2012.080
162. Kulemzin S, Evsyukov I, Belovezhets T, Taranin A, Gorchakov A. Horses for courses in the era of cars: advancing car t and car nk cell therapies. J Pers Med (2021) 11. doi: 10.3390/jpm11111182
163. Tang X, Yang L, Li Z, Nalin AP, Dai H, Xu T, et al. Erratum: first-in-man clinical trial of CAR NK-92 cells: safety test of CD33-CAR NK-92 cells in patients with relapsed and refractory acute myeloid leukemia. Am J Cancer Res (2018) 8:1083–9.
164. Pang Z, Wang Z, Li F, Feng C, Mu X. Current progress of CAR-NK therapy in cancer treatment. Cancers (Basel) (2022) 14. doi: 10.3390/cancers14174318
165. Sabbah M, Jondreville L, Lacan C, Norol F, Vieillard V, Roos-Weil D, et al. CAR-NK cells: a chimeric hope or a promising therapy? Cancers (Basel) (2022) 14. doi: 10.3390/cancers14153839
166. Heipertz EL, Zynda ER, Stav-Noraas TE, Hungler AD, Boucher SE, Kaur N, et al. Current perspectives on “Off-The-Shelf” allogeneic NK and CAR-NK cell therapies. Front Immunol (2021) 12:732135. doi: 10.3389/fimmu.2021.732135
167. Klingemann H. Challenges of cancer therapy with natural killer cells. Cytotherapy (2015) 17:245–9. doi: 10.1016/j.jcyt.2014.09.007
168. Morgan MA, Büning H, Sauer M, Schambach A. Use of cell and genome modification technologies to generate improved “Off-the-Shelf” CAR T and CAR NK cells. Front Immunol (2020) 11:1965. doi: 10.3389/fimmu.2020.01965
169. Töpfer K, Cartellieri M, Michen S, Wiedemuth R, Müller N, Lindemann D, et al. DAP12-based activating chimeric antigen receptor for NK cell tumor immunotherapy. J Immunol (2015) 194:3201–12. doi: 10.4049/jimmunol.1400330
170. Parihar R, Rivas C, Huynh M, Omer B, Lapteva N, Metelitsa LS, et al. NK cells expressing a chimeric activating receptor eliminate MDSCs and rescue impaired CAR-T cell activity against solid tumors. Cancer Immunol Res (2019) 7:363–75. doi: 10.1158/2326-6066.CIR-18-0572
171. McBride JE, Meaney MP, John C, Nieman DC, Warin RF. Flow cytometric analysis of natural killer cell lytic activity in human whole blood. J Vis Exp (2017) 2017. doi: 10.3791/54779
172. Mark C, Czerwinski T, Roessner S, Mainka A, Hörsch F, Heublein L, et al. Cryopreservation impairs 3-d migration and cytotoxicity of natural killer cells. Nat Commun (2020) 11:1–8. doi: 10.1038/s41467-020-19094-0
173. Gong Y, Klein Wolterink RGJ, Wang J, Bos GMJ, Germeraad WTV. Chimeric antigen receptor natural killer (CAR-NK) cell design and engineering for cancer therapy. J Hematol Oncol (2021) 14:1–35. doi: 10.1186/S13045-021-01083-5
174. Fang F, Wang W, Chen M, Tian Z, Xiao W. Technical advances in NK cell-based cellular immunotherapy. Cancer Biol Med (2019) 16:647. doi: 10.20892/J.ISSN.2095-3941.2019.0187
175. Goodridge JP, Mahmood S, Zhu H, Gaidarova S, Blum R, Bjordahl R, et al. FT596: translation of first-of-Kind multi-antigen targeted off-the-Shelf CAR-NK cell with engineered persistence for the treatment of b cell malignancies. Blood (2019) 134:301. doi: 10.1182/blood-2019-129319
176. Li Y, Hermanson DL, Moriarity BS, Kaufman DS. Human iPSC-derived natural killer cells engineered with chimeric antigen receptors enhance anti-tumor activity. Cell Stem Cell (2018) 23:181–192.e5. doi: 10.1016/j.stem.2018.06.002
Keywords: autologous immunotherapy, solid tumor, memory-like natural killer cells, cellular stress, upscale production, point-of-care manufacturing
Citation: Lizana-Vasquez GD, Torres-Lugo M, Dixon R B, Powderly JD II and Warin RF (2023) The application of autologous cancer immunotherapies in the age of memory-NK cells. Front. Immunol. 14:1167666. doi: 10.3389/fimmu.2023.1167666
Received: 16 February 2023; Accepted: 17 April 2023;
Published: 02 May 2023.
Edited by:
Daniela Wesch, University of Kiel, GermanyReviewed by:
Xiaofei Shen, Nanjing Drum Tower Hospital, ChinaEleonora Ponterio, National Institute of Health (ISS), Italy
Christian Kellner, LMU Munich University Hospital, Germany
Copyright © 2023 Lizana-Vasquez, Torres-Lugo, Dixon, Powderly and Warin. This is an open-access article distributed under the terms of the Creative Commons Attribution License (CC BY). The use, distribution or reproduction in other forums is permitted, provided the original author(s) and the copyright owner(s) are credited and that the original publication in this journal is cited, in accordance with accepted academic practice. No use, distribution or reproduction is permitted which does not comply with these terms.
*Correspondence: Renaud F. Warin, rwarin@carolinabiooncology.org