- 1Institut Català de Nanociència i Nanotecnologia (ICN2), Consejo Superior de Investigaciones Científicas (CSIC), The Barcelona Institute of Science and Technology (BIST), Universitat Autònoma de Barcelona (UAB), Barcelona, Spain
- 2Centro de Investigación Biomédica en Red (CIBER) en Bioingeniería, Biomateriales y Nanomedicina, Centro de Investigación Biomédica en Red en Bioingeniería Biomateriales y Nanomedicina (CIBER-BBN), Madrid, Spain
- 3Malalties Infeccioses, Nanopartícules farmacocinétiques, Vall d’Hebron Institut de Recerca, Barcelona, Spain
- 4Institució Catalana de Recerca i Estudis Avançats (ICREA), Barcelona, Spain
The long quest for efficient drug administration has been looking for a universal carrier that can precisely transport traditional drugs, new genomic and proteic therapeutic agents. Today, researchers have found conditions to overcome the two main drug delivery dilemmas. On the one side, the versatility of the vehicle to efficiently load, protect and transport the drug and then release it at the target place. On the other hand, the questions related to the degree of PEGylation which are needed to avoid nanoparticle (NP) aggregation and opsonization while preventing cellular uptake. The development of different kinds of lipidic drug delivery vehicles and particles has resulted in the development of ionizable lipid nanoparticles (iLNPs), which can overcome most of the typical drug delivery problems. Proof of their success is the late approval and massive administration as the prophylactic vaccine for SARS-CoV-2. These ILNPs are built by electrostatic aggregation of surfactants, the therapeutic agent, and lipids that self-segregate from an aqueous solution, forming nanoparticles stabilized with lipid polymers, such as PEG. These vehicles overcome previous limitations such as low loading and high toxicity, likely thanks to low charge at the working pH and reduced size, and their entry into the cells via endocytosis rather than membrane perforation or fusion, always associated with higher toxicity. We herein revise their primary features, synthetic methods to prepare and characterize them, pharmacokinetic (administration, distribution, metabolization and excretion) aspects, and biodistribution and fate. Owing to their advantages, iLNPs are potential drug delivery systems to improve the management of various diseases and widely available for clinical use.
Introduction
The modern concept of drug delivery probably started with German Nobel laureate Paul Erlich’s “magic bullet” in 1907, a bullet that cannot miss its target (1, 2) and became common with penicillin in the early 20th century (3, 4). The initial idea was to kill prokaryotes leaving eukaryotes unharmed, thus reducing damage to the body associated with uncontrolled biodistribution. Soon it was also developed to improve dosing and, with it, therapeutic effects. More recently, the concept was actualized for chemotherapy due to its severe side effects, and thus, the firsts Drug Delivery Systems (DDS) were developed to improve the transport of antitumoral drugs such as doxorubicin (5). Before, excipients allowed the drug to solubilize and properly reach their target, but their capacity was limited to solubility issues, with poor capabilities in directing and protecting the drugs during the journey to the target. Similarly, if the development of DDS was initially intended for solubilizing common drugs, it rapidly opened the possibility of loading other substances, such as genetic material or proteins –antibodies, enzymes, etc.-. These substances cannot be administered in a free form since they are highly immunogenic and rapidly biodegraded. Therefore, the full development of DDS will not only optimize the pharmacology of current drugs but also dramatically expand the pharmacopoeia we have available for the cure, which will have a clear impact on population health.
It is important to note that therapeutic effectiveness strongly depends on pharmacokinetic aspects, on how drugs travel and interact through the body, reach their target, perform their intended effect, are modified (metabolized), and excreted. Up to now, pharmacokinetic principles were based on small drug properties, where balanced hydrophobicity and hydrophilicity allow it to reach all corners of the body (6) so that reaching the target was assured at the expense of undesirable side effects. For example, after cisplatin injection, a common chemotherapeutic agent, 60% goes to the kidney causing nephrotoxicity, 36% irreversibly binds to albumin losing its activity, and only a small fraction of the remaining 4% reaches the tumor cell’s DNA and performs its therapeutic action (7). In this scenario, protecting the drug and carrying it to the region of interest is a natural evolution of pharmacology.
Together with the quest for efficient drug administration, chemists and nanochemists have searched for a universal carrier that can accommodate many different substances. In addition, the carrier has to be safe, more than its loading. DDSs have to be so safe that repeated administrations across one person’s life should not be a problem. Also, for practical reasons, they have to be easy to produce and easy to store.
As expected, DDS have been developed and exploited to enhance the delivery of drugs in treating several diseases showing potential benefits in terms of pharmaceutical flexibility, selectivity, dose reduction and minimization of adverse effects (8). In these platforms, different drugs, ligands and biomolecules can be combined by absorption (9), loading (10), coordination bonding (11), and entrapment (12) to perform different tasks. While DDS popularization started in the 80s and developed as a full academic and technological discipline, nanotechnology irrupted in the 2000s offering unprecedented control of mater structured at the nanoscale, allowing for the rapid and widespread development of new, more precise and more functional DDS. Interestingly, the initial vehicles were called microspheres and then renamed nanocarriers, even if some nanocarriers were bigger than some microspheres.
Among DDS, polymeric nanoparticles (NPs) were the first to be employed, showing significant therapeutic benefits but accompanied by polymeric toxicity, high cost, and lack of feasibility for scaling up. Besides, liposomes have traditionally been the more developed and implemented DDS. Lipid-based NPs made of lipids and surfactants (amphipathic molecules) are simple and safe, but there is typically a low degree of loading and structural fragility. They have become an up-and-coming delivery platform for hydrophobic and hydrophilic substances or a combination of both (13, 14). These carriers can penetrate abnormal tissue, remain for a long time and release their cargo drugs, increasing drug efficacy. For example, in-vitro studies by Wang et al. (15, 16) have shown the successful target delivery of resveratrol, a poor aqueous solubility drug and curcumin hydrophobic polyphenol in breast cancer. Recently developed lipid-based formulations included micro and nanoemulsions, self-emulsifying formulations, liposomes, lipid NPs and lipid-drug conjugates. Among the extensive range of lipid formulations, the solid lipid nanoparticles (SLNs) (17–19), the nanostructured lipid carried (NLCs) (14, 20), and the lipid-based nucleic acid therapeutics (21, 22) have probably centered the majority of the attention due to their successful activities toward multiples disease models. Other alternatives have been protein aggregates for unsoluble chemotherapeutic agents such as paclitaxel in Abraxane® (23) or made of biological molecules such as polylactic-co-glycolic acid (PGLA) NPs, which has been considered one of the first universal nanocarrier platforms (24, 25).
In this work, we refer to those NPs made of a mixture of lipids, ionizable surfactants, and nucleic acids, which spontaneously form NPs by the electrostatic and hydrophobic collapse in water. These lead to highly dense and highly protective NPs, which can be singularized as ionizable lipid nanoparticles, iLNPs. These NPs can overcome the main biological barriers to cell transfection, including protection from endonucleases, and RNases, and selective targeting, when targeting moieties such as antibodies or aptamers are included, to improve the contact with the targeted tissues or cells (26), cell internalization, and intracellular release. Herein we focus on RNA-ionizable lipid NPs. RNA is not only a major player in genetic medicine that needs to be transported, but it is also a model of macromolecule that has to be protected until delivered inside the cells.
From a historical perspective, ionizable lipids evolved from years of working with permanently charged cationic lipids for transfection. The mechanism by which these cationic lipids capture nucleic acids is through complexing them by ionic interaction between the negatively charged phosphate groups on the nucleic acid molecules and a positively charged group on the lipid head, forming nucleotides-lipid complexes-, probably starting in 1987 with DOTMA, the first bi-layer forming cationic lipid, specifically designed and used for DNA transfection (27). However, due to its net positive charge, it presented unacceptable levels of toxicity at the doses necessary to produce therapeutically relevant levels of transgene expression, especially in vivo animal models, making its transition into clinical praxis impossible (28–30). In addition, the positive net charge induces plasma protein adsorption and rapid clearance by the immune system, negatively affecting transfection efficiency (31). They commonly present hemodynamic toxicities, such as the activation of the complement system and an increase in blood coagulation time (32–34). Ionizable lipids entered the field as an answer to this problem. These lipids are a pivotal element of the iLNP systems (35) and are characterized by having an ionizable functional group in their polar head with an acid-dissociation constant (pKa) below 7.0 (36). Their pH-tunable charge allows them to be neutral at physiological pH, minimizing their cationic burden and toxicity but be protonated at a lower pH at the maturing, acidified endosome. Their cationic nature inside the endosome will help its break and escape by enabling the interaction with the anionic membrane lipids (and subsequent formation of non-by-layer phases), allowing cytosolic delivery. Indeed, the ability to activate and deactivate the ionizable lipid cationicity, when necessary, enables it to adapt to the needs of the synthesis (charge on), distribution within the body (charge off) and escape from the endosome (charge on). These positively charged vesicles present the advantages of a liposome-mediated transfection (e.g., fusion with the cell membrane, protection from degradation, digestion, opsonization, etc.) and of a cationic-mediated transfection (e.g., complex formation with nucleic acids, association with the negatively charged cell surface).
Today, iLNPs have proven to be an efficient vehicle for effectively delivering RNA inside the cells, opening the doors to gene therapy. Their rapid implantation in several medicines already approved for human use is an unprecedented success within the community of nanomedicine, drug delivery and gene therapy. Though, to date, only three different systems of RNA delivery are approved by the FDA: antitumoral Patisiran and two RNA COVID-19 vaccines (Pfizer/BionTech and Moderna) (37), and many others are under clinical trial. Encouraged by the successful application of the SarCov2 mRNA-lipid vaccines produced by Moderna and Pfizer companies, the high biocompatibility of the lipid nanocarriers is being explored to treat many other diseases. Thus, in a continuous effort to cure other viral diseases, other mRNA vaccines are developing to fight against etiological agents such as Cytomegalovirus, Syncytial respiratory, or influenza viruses (Trial number: NCT05085366, NCT05127434, NCT04956575). In the field of cancer disease, the pharmaceutical companies Moderna and BioNTech are advancing in the commercialization of a potent therapy based on mRNA vaccines for melanoma; their clinical trials are in phases 1 and 2, respectively (Trial number: NCT0389788, NCT04526899). The multifunctional characteristics of the iLNPs also have been advancing in treating solid tumors. In a lack of successful results compared to the CART cell therapy in liquid tumors, the BioNTech company has developed an RNA-based CAR-T cell therapy to counter the accelerated growth in the Gastric, Pancreatic, Ovarian, and Biliary Tract Tumors (Trial number: NCT04503278) (38).
Ionizable lipid nanoparticles: Structure, composition and characterization
Ionizable Lipid NPs consist of nanometrically sized particles (39) could be understood as a dense condensation of surfactants, lipids and genetic material. These NPs can be synthesized quickly and efficiently to encapsulate genetic material with high efficiency (high density) and have sufficient stability and robustness to travel through the body to their destination without being degraded or opsonized, protecting their cargo, and able to carry out an efficient cytoplasmic delivery of the genetic material. Their standard composition consists of 5 different compounds: i) the genetic material which has to be delivered, ii) an ionizable cationic lipid to interact with the genetic material and render it hydrophobic, iii) a helper amphipathic molecule, usually a phospholipid such as DSPC, iv) a helper sterol lipid, in the majority of cases Cholesterol, to making the structure more robust, and v) a PEG-lipid at the particle surface for surface stabilization and to avoid NP aggregation and opsonization. The ratio between these components varies among different formulations, but typically it can be around: ionizable lipid 50%mol (60%mass), phospholipid 10%mol (15%mass), Cholesterol 38%mol (15%mass), PEG-lipid 1,5%mol (8%mass).
These components interact and spontaneously structure themselves through a self-assembly process based on the ethanol injection method, which consists of rapid mixing of an ethanol phase, where the lipids are dissolved, into an aqueous phase, where the nucleic acids are dissolved in an acidic buffer. This rapid mixing induces the sudden supersaturation of the lipidic molecules, which leads to burst nucleation and their assembly into NPs, trapping the surfactants and genetic material (36). The process by which the genetic material is encapsulated in the lipid structure takes place in the first steps of the synthesis process when the ethanolic phase is mixed with the aqueous phase and self-assembly of lipids occurs. The first force that drives this self-assembly is an electrostatic interaction between the polar head of the positively-charged ionizable lipids at acidic pH and the negative charges of the nucleic acid chains at the working pH (typically around 4), and the second is the increase in polarity of the lipidic solvent by the addition of water, expulsing lipidic material from the liquid phase into the NPs. Thus, as the polarity of the solvent progressively increases, inverted micelle-like structures coalesce, interacting with the rest of the lipids and surfactants, which at the NP surface closes the particle in a spherical form making the NPs soluble in water.
The PEG-lipid anchors to the NP surface’s lipidic domains while extending its water-soluble part away from the NP, forming a hydrophilic steric barrier that provides colloidal stability and prevents NP aggregation and opsonization (40). The whole phase mixing and complexation of the nucleic acid is done at pH<<pKa of the ionizable lipid so that it is cationic-charged nature, and the entrapment efficiency of the nucleic acid is maximized. Afterwards, once the synthesis is complete, the pH can be adjusted to physiological value since the nucleic acid is already complexed and integrated inside the NP. In this way, a neutral charge of the vehicle is achieved and significantly minimizes the cationic burden and its related toxicity that the particles would experiment with once they are administered (Figure 1).
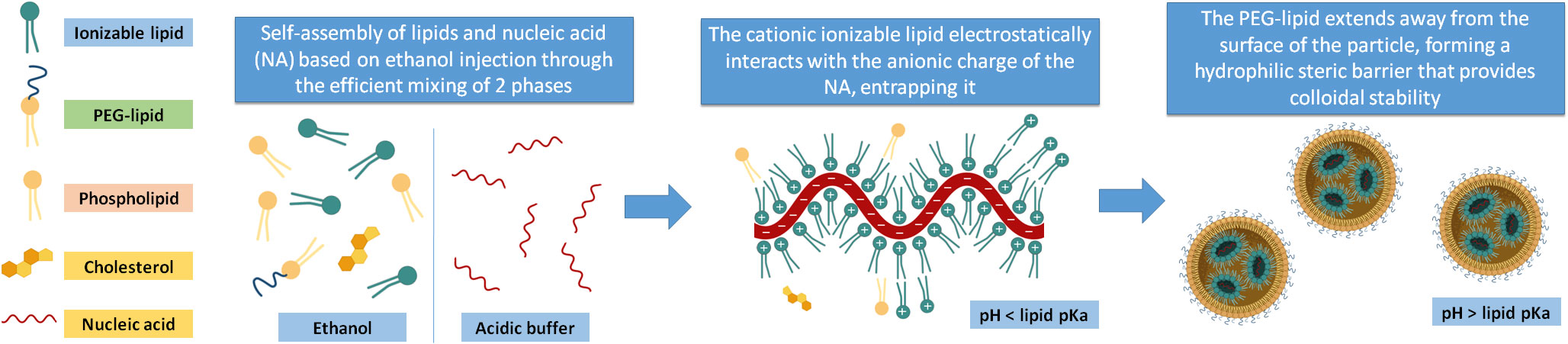
Figure 1 Main components of the iLNPs. The lipid components are dissolved in an ethanolic phase, and the nucleic acid is in an acidic buffer. When these two phases are efficiently mixed, the ionizable lipid gets protonated and electrostatically interacts with the anionic charges of the nucleic acids while the rest of the lipids self-assemble to form the iLNP structure. When the formation is complete, the pH of the batch can be brought to a value higher than the ionizable lipid pKa.
An essential part of this process is how efficient and fast the mixing between these two phases is, since it determines size, and size plays a decisive role in NP biodistribution, delivery efficiency, and transfection potency (41). As shown by Belliveau et al.in 2012 (42) when investigating the influence of the flow rate on the iLNP particle size, the NP size decreased as the flow rate of the injection of the ethanolic phase into the aqueous phase increased. Indeed, there is an universal tendency for NPs to decrease surface energy (surface-to-volume ratio) by growth. Because of that, the PEG-lipid is employed to reduce the surface energy and stabilize the NPs. Multiple studies have shown that increasing the molar ratio of the PEG-lipid, by stabilizing NPs against aggregation yields significantly smaller iLNPs, independent of other lipid components (41–43). This indicates that without PEG, or other similar biocompatible polymers, the NPs would continuously aggregate and grow until complete phase separation.
Besides size, as discussed in the previous section, a determining functional parameter is the charge, a fundamental aspect of iLNPs, which should be positively charged during iLNPs formation to allow nucleic acid complexation, neutral at physiological pH for its administration, and positively charged at the acidified, maturing endosome for membrane disruption. The pKa value of the ionizable lipid will be the factor that determines the charge on the iLNP under the different pH conditions, which has to find a balance between (44, 45) i) being acidic during RNA trapping, ii) being neutral at physiological pH, to minimize toxicity and avoid rapid immune-clearance, iii) being as positively charged as possible at late endosome stage to maximize the interaction with the endosome’s membrane and its disruption. Also important to consider the effect produced by absorbing protons during endosome acidification, inducing proton sponge effects.
The pKa value in which this balance is optimal is not a universal value for all lipids and depends on the iLNPs formulation and the nucleic acid sequences they carry. However, several studies demonstrated that a pKas between 5,5 and 6,5 tend to show maximal potency in vivo (21, 46–48). Regarding their chemical structure, as a rule of thumb, one can say that small head groups of the ionizable lipid, such as dimethylamino-based, show higher transfection efficiencies compared to higher substituted moieties, which increase their steric hindrance as well as affecting the pKa (49–51). So it is the case of DLin-MC3-DMA, the ionizable lipid part of the patisiran (Onpattro®) formulation. It is important in the history of the development of ionizable lipids and corresponding NPs since, when first synthesized, it exhibited an improvement in the potency of more than two orders of magnitude compared to the previous benchmark formulation (DLinDMA), which allowed the TTR02 (later known as patisiran) formulation to transition into clinical development (45). Notably, the structure and formulation of DLin-MC3-DMA laid the groundwork for further iLNPs development. Currently, the search for new biodegradable, ionizable lipids with more potency or different properties is at the center of research to advance iLNPs, and this effort is yielding a large array of diverse and exciting types of iLNPs to adapt them to organs and diseases (52). The structures and pKas of the ionizable lipids present in the approved iLNP formulations appear in Figure 2.
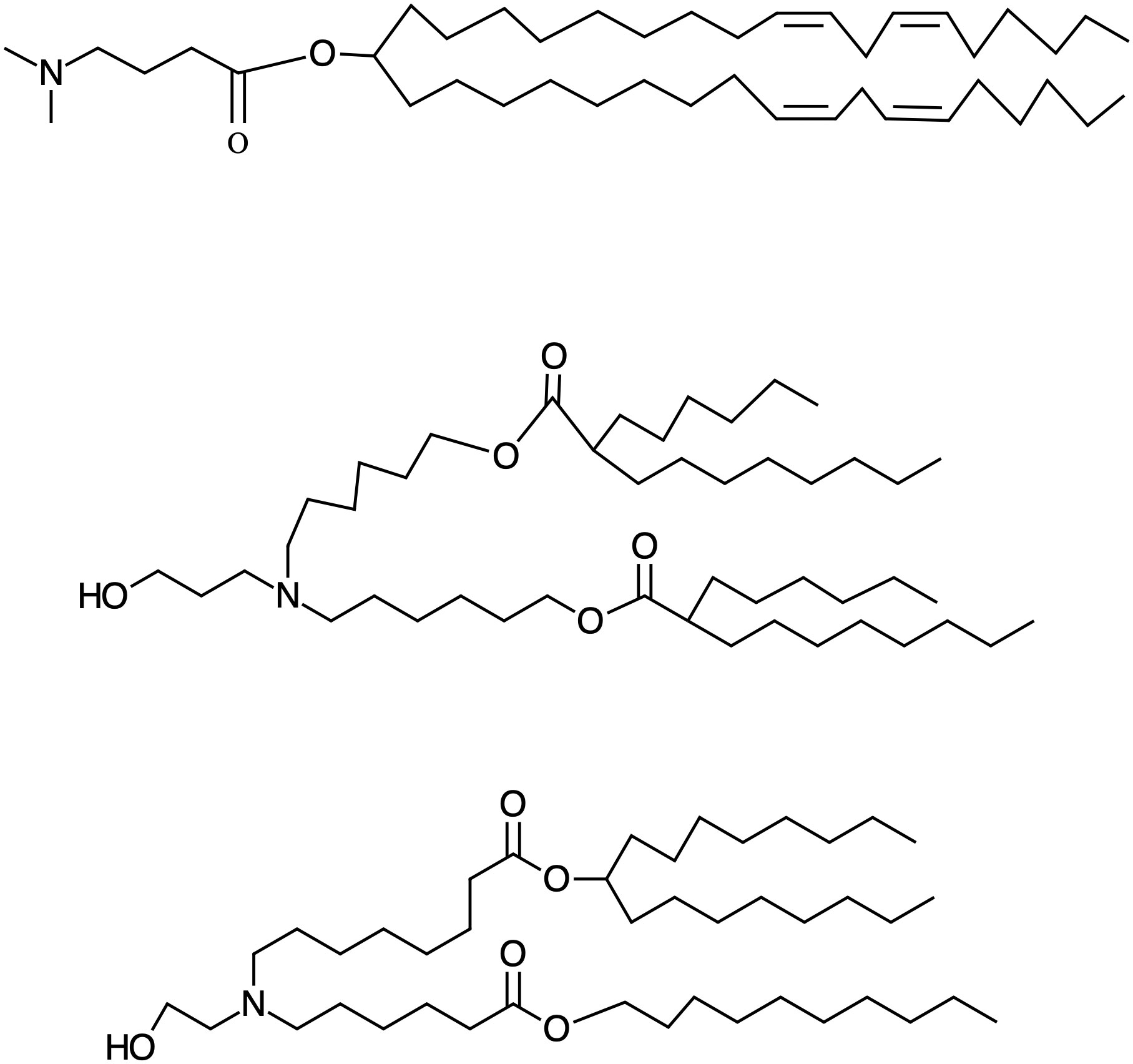
Figure 2 Structure of the approved Ionizable lipids. From up to down DLin-MC3-DMA, Alc-0315 and SM-102, the ionizable lipids present in Onpattro, Comirnaty (BioNTech/Pifizer) and Spikevax (Moderna), respectively, with the pKas: 6.44, 6.09, 6.75, respectively. The number of hydrophobic tails has geometrical consequences in the structure of the iLNPs contributing to the determination of size and robustness.
They share branched (bulky) lipophilic moieties and hydrophilic amino terminations. This geometry allows for a cone-like conformation of the amphipathic molecules and of the cationic-anionic lipid pair that occurs once the ionizable lipid interacts with the lipids of the endosomal membrane. This non-by-layer, cone-like conformation of the amphipathic molecules favors high surface curvature and is responsible for iLNP disruption. Once the endosome and the iLNP have been disrupted, the pH goes back to 7, and the iLNP release its cargo so that free mRNA can enter ribosomes for expression. This disintegration is probably simultaneous with the endosomal disruption and mediated by the many (negatively) charged and detergent-like (amphipathic) compounds inside the cell –indeed, all proteins have hydrophilic and hydrophobic domains- interfering with the amphipathic molecules of the iLNPs.
Due to their reduced size and complex and unstable (dynamic) nature, their characterization can be a serious challenge, especially in the biological matrix (Figure 3). The standard parameters to evaluate include particle size, surface charge (ζ-potential), drug content and surface state (composition and conformation). Particle size, polydispersity index and charge analysis can be measured by dynamic light scattering (DLS) and associated ζ-potential with the main advantage of not being time-consuming. Besides, electron microscopy allows for high-resolution observation of these NPs. However, they are frail in high vacuum and under the electron beam, and therefore cryo-TEM is often employed, providing 2D images of stable frozen-hydrated particles. Alternatively, low electron beam energy and staining also allow observation of the iLNPs morphology (53). These iLNPs can also be fluorescently-labelled for their visualization and quantification in fluorescence microscopy and spectroscopy techniques (54). Concentration and composition are also studied using thermogravimetry and differential scanning calorimetric analysis (55).
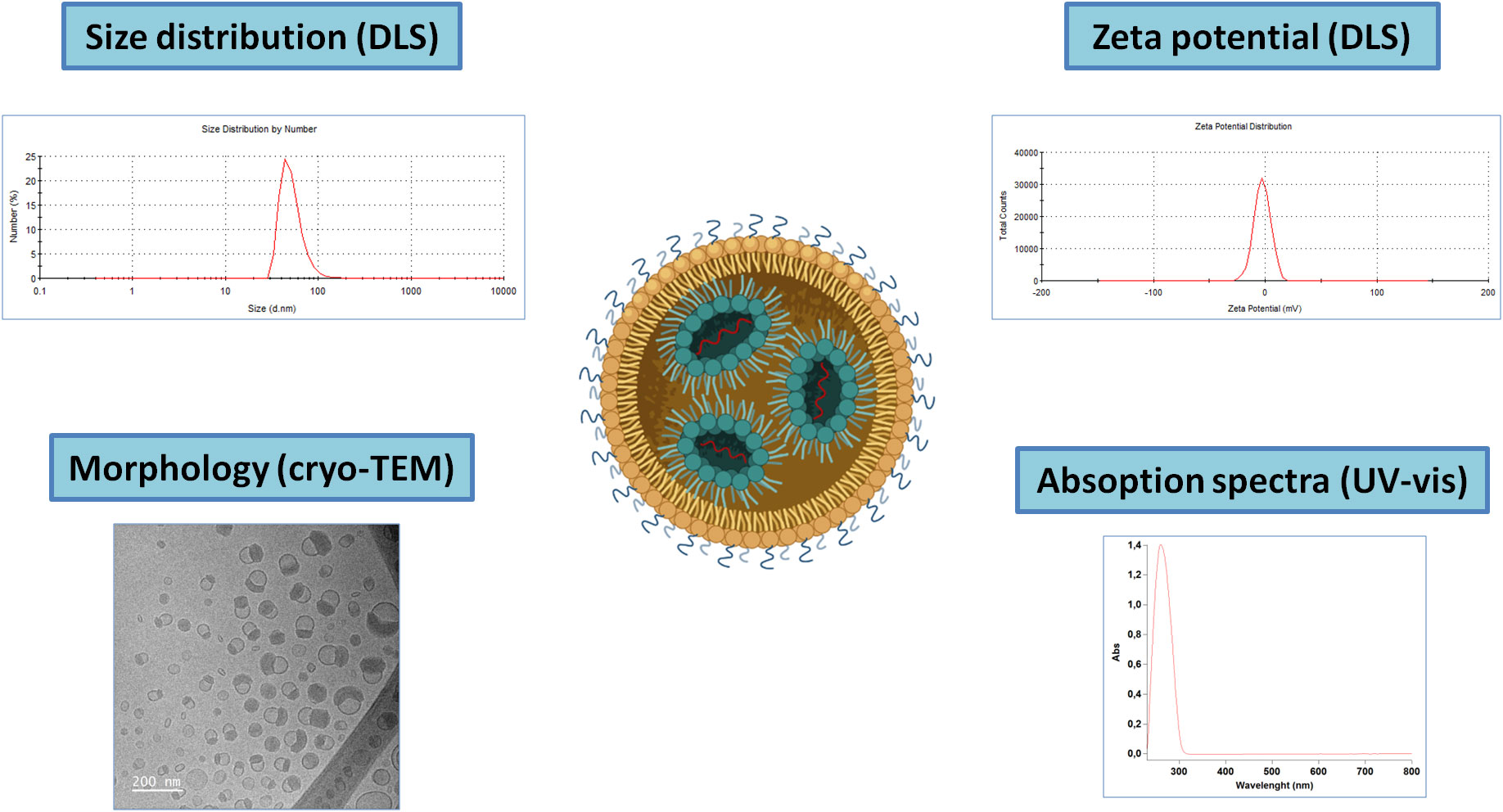
Figure 3 Schematic representation of the main techniques for iLNPs characterization. Note that for the UV-vis absorption spectra, the signal corresponding to the nucleic acids present in the sample will account for the majority part of the signal.
Information about the internal structure of the systems can be obtained through X-ray (SAXS) and neutron (SANS) small angle scattering (55). In the past decade, SAXS has been shown to be very useful at providing information about the fine structure in self-assembled soft matter materials, like iLNPs (56–59). Still, the full understanding of the nanoscale organization of the lipids and genetic material in the interior of the particle remains yet to be achieved (60). It has been observed that depending on operational factors or compositional changes, the synthesis yields different arrangements of iLNPs, such as multilamellar structures (61), Ia3d and Pm3n cubic phases (62), and other types of non-lamellar structures where the RNA molecules are inside aqueous cylinders (63).
Ionizable lipid nanoparticles: Pharmacokinetics
It is well accepted that the potential use of iLNPs in medicine is determined by the pharmacokinetic (administration, distribution, metabolization and excretion) aspects that govern iLNP behavior, which is different from previous drugs. Pharmacokinetics describes what the body does to the drug rather than what the drug does to the body; the latter would be pharmacodynamics. The field of pharmacokinetics has developed with the implantation of small-molecule drugs as principal therapeutic agents. This is because small molecule drugs can distribute across the body and enter inside the cells. However, everything changes when we pretend to employ large and structured substances such as proteins, genetic material or nanoparticles. Because of that, new pharmacokinetic models, which describe the behavior of these materials once injected until they are excreted, have to be developed for the proper implementation of new medical substances and materials into the clinic practice, taking into account that the biochemical composition of NPs and its entrance route into the human body, determines the final activity of these NPs (64). We focus on the existing clinical trials and in-vivo experimental models using RNA-lipid carrier systems. Many DDS enter the body via inhalation, oral ingestion, topical (cutaneous and ocular) application, and parenteral administration.
Herein, we first analyze the different transformations iLNPs may suffer, such as aggregation, interactions with proteins and disintegration/dissolution, and then comment on their biodistribution and excretion since the latter strongly depends on the formers. These alterations significantly impact their behavior and must be considered for their intended use in medicine. Therefore, research on iLNPs effects should strive to correlate with how they interact, evolve and are transformed during their exposure to the human body. That is, during their Administration, Distribution, Metabolization and Excretion (ADME) phases.
Transformations and metabolization
Regarding nanosized objects in general, due to interactions between NPs and components from the biological medium, NPs are known to suffer different alterations when applied. Indeed, NPs are intrinsically out of equilibrium, and transformations such as Ostwald ripening, NPs collapse, and over-grow always tend to occur. When administered, the NP’s environment radically changes from low electrolytic NP concentrated media to a media full of cells, proteins and electrolytes. The main alteration they may suffer is the loose of colloidal stability and consequent aggregation. This loss of colloidal stability and subsequent aggregation and expulsion from the media has a dramatic effect on the abilities of NPs to travel through the body and to be well dispersed in organs and tissues, provoking, in too many cases, the lack of -or unexpected- biological results (65, 66). Note that the final size of NP, which will reach the cells, is also determined by the interaction with the biological matrix, ultimately determining the distribution and kinetics of delivery.
Several factors cause the aggregation of colloidal NPs; for instance, the initial concentration of NPs, their chemical nature and the ionic strength of the medium (67). The most widely employed strategy to passivate the surface of nanosized objects, in general, has been modifying the NP surface with hydrophilic polymers as polyethyleneglycol (PEG), which acts as a steric barrier that minimizes interactions at the NP surface, indispensable to stabilize the surface and allow NPs to exist isolated in solution. This steric barrier “closes” the surface of the NP, provides colloidal stability and facilitates the small and narrow size distribution. However, the PEGylation of the NP surface dramatically difficult the interaction with cellular membranes, reducing the necessary close contact required for endocytosis. This double effect has been called the “PEG-dilemma” (68–71). Although alternative strategies are proposed, like cleavage of a PEG moiety (72), this problem is majorly addressed by a strategy based on “reversible PEGylation”, where a lipid-containing PEG slowly detaches from the NP surface once administered. This allows to take advantage of the disposing of the high PEG concentration needed for small and monodisperse synthesis and distribution, and the lower PEG concentration needed to have a good cellular uptake at the moment when the NP reaches the target organ. Note that an increase in the concentration of PEG also influences its conformation and protective effects, increasing the circulation time of the iLNPs (71). Indeed, a completely PEG-covered iLNP surface will dramatically inhibit the interaction with cells and serum proteins, modifying circulation time and biodistribution (68, 71). Such PEG-lipids remain integrated into the LNP structure during formation and under storage conditions, but in the presence of a lipid sink like in plasma, these PEG-lipids are stripped off the particle and into de medium, leaving the surface of the iLNP gradually unshielded (45). These PEG-lipids have short alkyl chains -which act as “hydrophobic anchors”-allowing a reasonable desorption rate once they enter blood circulation (71). The proper balance between a fully protected surface for iLNP synthesis and distribution, and a relatively unshielded surface for interactions with cell membranes, is achieved by finding a compromise between alkyl chain length and PEG-lipid surface concentration.
Special mention deserves the interaction of NPs with proteins, adjusted by the size and concentration of PEG at the NP surface. When NPs are administrated into the body, they first interact with biological fluids. Depending on the administration site, the biomolecules that will interact with the NPs can vary: from lung surfactants when inhaled to the interstitial fluid when locally injected into blood plasma following intravenous administration (73). Proteins, which are the most important of these biomolecules, will adsorb -to some extent- on the NP surface, especially as PEG is removed, coating the surface with a new layer that will define the biological identity of the NP, the so-called “biomolecular corona”, or “protein corona” (74, 75) which can dramatically alter the surface properties of nanosized particles and determine their in vivo fate (76). Already in 2004, it was reported that the presence of proteins in physiological media affects the entry and intracellular localization of NPs in cells, thus modulating their potential biological effects and toxicity. Later on, the formation of a protein corona on top of the NP surface was observed to control biodistribution, uptake and biological response, transforming them from innocuous to toxic or vice versa. This surface coating can be formed by hundreds of biomolecules, including albumin, apolipoproteins, immunoglobulins, coagulation factors, and many others (77). Some of these biomolecules might associate almost irreversibly with the iLNP surface, in either their native or denatured form, affecting, de facto, all subsequent interactions. It has been proposed that the corona is comprised of both these tightly bound proteins (“hard” corona), which presumably bind directly to the iLNP surface with high affinity, and also a looser, more dynamic layer (“soft” corona) which constantly exchanges with proteins in the environment (78). The hard and soft corona are both considered relevant in determining iLNP interactions with cells (73).
Traditionally, for biomedical applications, this corona has only been conceived as a disruptive effect that hinders the functionality of nanoparticles, provoking underestimated side effects like loss of colloidal stability, aggregation, sedimentation or rapid clearance by the immune system. But the protein corona also plays an active role in deciding the destination organ for delivery and accumulation of the particles. For iLNPs, it has been shown that in Onpattro, there is a close relationship between the protein corona and the target organ of delivery, mediated through the adsorption of Alipoprotein E onto the iLNP surface. Authors proposed that binding to ApoE will act as a highly effective targeting ligand by binding to lipoprotein receptors on the surface of hepatocytes, triggering the uptake by hepatocytes (45, 79). This relationship between the protein corona and the biodistribution of NPs could allow the fate of the particles to be actively altered. Indeed, the multifunctional physicochemical properties of lipids can be designed to target different body tissues. Min Qiu et al. (80) have achieved a lung-selective delivery in mice with the use of a series of ionizable lipids containing an amide bond in the tail which changes the interactions between plasma proteins in contrast with other types of lipids, like the ones with an ester bond in the PEG lipidic tail (as those present in the approved formulations), which easily accumulate in the liver (81). These are exciting and promising results for improving the delivery of iLNP beyond these organs, and other relationships between lipid composition and biodistribution should be carried out in the future. Active targeting by grafting a specific moiety, that is, the ligand of an over-expressed receptor onto the NP surface, is a very appealing strategy that, to this day, fails to impact the biodistribution drastically. However, once the NPs have reached the organs, their uptake can be influenced by targeting moieties (75)
Degradation and disintegration of iLNPs
It is well-known that NPs can dissolve in certain dispersing media (82–84). The extent of their dissolution depends not only on their intrinsic properties, such as size and shape, but also on characteristics of the surrounding media, including pH and ionic strength, as well as the presence of organic matter (85, 86). Thus, while small NPs can be preserved in solution in the appropriate (as–synthesized) conditions for a long time, they may also be prone to rapid degradation in physiological media. This is why iLNPs can be kept for a long time in storage conditions while in hours disintegrates once in the body. Indeed, it has been reported that it is below c.a. 30 nm in diameter (87), where NPs cannot support the high surface energy anymore and tends to dissolve. The driving force behind dissolution strongly depends on the solubility of the constituent ions in a given environment and their concentration gradients in the solution. This phenomenon, enhanced at the nanoscale, is referred to as the Gibbs-Thomson effect, and in NPs manifests as Ostwald ripening, where NPs in solution spontaneously dissolve or grow due to concentration gradients, becoming progressively larger and more polydisperse. Controlled release of matter from an NP is illustrated in the Noyes-Whitney equation, which relates the rate of dissolution to the properties of the components and the dissolution medium. If the released components are removed from the equilibrium because, for example, are used in competitive reactions or simple diluted in the body, the system is moved away from the saturation point, reaches sink conditions, and the NP tends towards complete dissolution. For a given mass, the kinetics of dissolution will be proportional to the specific surface area and the coordination of the constituents at that surface (which decreases with size). NPs have to release their cargo at the appropriate rate and quantity: larger NPs may release them too slowly and too much, while the smaller ones may release them too fast and an insufficient amount of it. Thus, the reactivity of the NP has to be adjusted to persist more or less inside the different parts of the body.
Biodistribution and fate of iLNPs
The primary purpose of using these iLNPs is to cross natural barriers, interact with the target cell, and deliver the treatment efficiently. The first natural barrier the iLNPs need to cross is the biological fluids, blood or lymph, sweat or tear, and the corresponding extracellular matrix, consisting of macromolecules and minerals that change in different tissues, compartments, and health status (88). To overcome these barriers, some iLNPs can interact with particular matrix components that facilitate the interaction with the target cell and the entrance by endocytosis (87). The second natural barrier is the mononuclear phagocyte system, capable of recognizing foreign substances and commensal organisms when entering the body, labelling them with opsonins and enhancing uptake by phagocytic cells, such as kupffer cells in the liver. Basically, if NPs are recognized as a foreign substance, the innate immune response reduces their plasma half-life, decreasing the drug delivery efficiency to the target cell. However, the size range of NPs is that of recognition of the immune system which is supramolecular structures and molecular patters of few tens of nm, mainly found is viruses and bacteria, in such a way that the immune response to NPs can be complex from tolerance, to pro-inflammation to immunomodulation (89). As mentioned above, the lipid nanoparticle-surface functionalization with PEG minimizes opsonization and therefore the immune response and increases blood circulation time (90). Nonetheless, the activation of the immune system has been found against some of the iLNPs components as PEG. It has been reported that some patients can develop anti-PEG antibodies after a first dose of a PEGylated drug (anti-PEG immunoglobulin M (IgM)) (91), leading to rapid NPs clearance in the liver and spleen, removing the drug from circulation (92). Fortunately, PEG immunogenicity is not so prevalent and not so aggressive, while the rest of the approved iLNPs components are safe.
The precise behavior of these materials during their full-life cycle inside the body is still relatively unknown, with controversy about disparities between the in vitro and in vivo results. Additionally, subtle modifications of their nature -composition, size, shape and surface state- may have or not have a strong influence on their behavior, affecting their interaction with proteins (93), aggregation state (94), chemical transformation and degradation (95), and consequently biological responses (96). Once they are stable and do not aggregate when administered, their behavior is very different from small molecules, and they are subordinated to the many-body barriers to protect integrity. In addition to immunogenicity, which is somewhat tolerant to small molecules, the body is full of physical barriers. Considering intravenous administration, it is essential to note that the main blood vessels and capillaries in the body have a continuous lining of endothelial cells with pores of 6 nm. Besides, the fenestrated capillaries found in the intestine and some endocrine and exocrine glands may have pores up to 50–60 nm, while discontinuous capillaries, as those found in the liver, spleen and the bone marrow, have pores between 100–1000 nm, which is where typically NPs are found (97). Special attention deserves the tight junctions, including the blood-brain barrier, placenta and testis barrier, where pores smaller than 1 nm have been reported, where the hydrophobic nature of LNPs seems to favor translocation (98). In such conditions, small molecules can diffuse in-and-out from the blood vessels into the lymph, while the passive transport of large objects, like proteins and NPs, through these porous is negligible, and they tend to accumulate in organs of the mononuclear phagocytic system, such as the liver and spleen, which are the two usual places of NPs fate and accumulation (97, 99).
It is worth noting here that blood vessel and tissue permeability is altered during the course of diseases, allowing for the passive accumulation of NPs in those areas. Indeed, this passive accumulation can increase one order of magnitude the concentration of the drug in tissue (11). For example, in solid tumors, their rapid growth results in leaky vessels with large pores resulting from a defective angiogenic process, which facilitates NP accumulation in the absence of a functional lymphatic drain. This phenomenon, known as the Enhanced Permeability and Retention effect (100), is widely reported in the literature and has been exploited to accumulate nanocarriers in tumors (101) passively. Note that protein aggregates and cell debris are naturally found in solid tumors due to the EPR effect. Thus, by increasing NP circulation times, this passive accumulation has been employed to deliver therapeutic doses of drugs. It is the case of Doxil (liposomal doxorubicin), where the inclusion of PEG-1,2-distearoyl-sn-glycero-3-phosphorylethanolamine (PEG-DSPE) extended circulation time over 4- to a 16-fold enhancement of drug level (101). With this increased circulation time, the Doxil liposome formulation could accumulate more at the tumor site (101), achieving a more significant therapeutic effect (102, 103). Besides, blood and tissue porosity increases during inflammation, which allows NPs to accumulate in those sites (104).
Once the NPs reach their target cells, they must enter and deliver the cargo. The cytoplasmatic membrane is very robust and impermeable; things naturally enter either transported, typically ions and small molecules, or endocytosed, for proteins and larger objects. Endocytosis can be divided into pinocytosis (cell “drinking”) and phagocytosis (cell “eating”). Pinocytosis, commonly termed endocytosis, is when a fraction of the membrane is invaginated, and whatever is on its surface or around it is trapped. Endocytosis can be receptor-mediated or receptor-independent when the cell membrane recycles (105). Alternatively, substances like cationic detergents can permeate through the membrane, and large liposomes can fuse with the membrane. Both pathways often show toxicity, especially membrane permeation, being endocytosis the most benign way to introduce substances inside the cell. For the case of iLNP the successful endocytosis process is determined by their size, surface composition, and target cells (99). Once the iLNP reaches the target cell and is up-taken by endocytosis (45), the cargo must be released and reach the cytoplasm. It is important to note that the relationship between cellular uptake of NPs and transfection efficiency is not trivial since this is determined by the ability to escape from the endosome. Once engulfed into the cell by the endocytic process, the early endosomes mature into late endosomes and fuse with lysosomes decreasing pH and digesting its content for recycling (106). When the pH becomes smaller than the ionizable lipid pKa, it becomes positively charged again, enabling an electrostatic interaction between the iLNP and the negatively charged lipids of the cell membranes, as cationic lipids do.
This interaction can have disruptive effects, first on the NP structure and integrity, and second, on the endosome membrane, leading to its disruption (49, 107–109), promoting endosomal escape and cytoplasmatic release of the nucleic acid cargo (107). As the electrostatic interactions between ionizable lipid and RNA increase as pH decreases, it is difficult to imagine the liberation of the cationic surfactant associated with the RNA from the NP, indicating the presence of an excess of ionizable lipids in the iLNP formulation. It has been proposed that endosome disruption is achieved by forming non-bi-layer phases due to the electrostatic interaction between the lipids. Of particular interest is the HII inverted hexagonal structure since it’s been shown that this phase is not compatible with bilayers, and, as lipids tend to adopt it once they are mixed, membrane fusion and membrane disruption events are more likely to occur (35, 49, 107, 109). Regarding endosomal disruption, another possible factor playing a significant role in endosomal disruption would be the proton sponge effect. The proton sponge effect happens when during the maturation of endosomes, HCl influxes to decrease pH for digestion. The amine groups of the cationic lipid become protonated, capturing protons from the media that resist acidification. As a result, more protons are pumped into the endosomes, followed by passive entry of more chloride ions by osmosis, the consequent increase in ionic concentration, leading to osmotic water influx and swelling, and up to rupture of the endosomes -and endolysosomes-, releasing their contents into the cytosol (110). Likely, membrane disruption and proton sponge effects happen in parallel: while the excess of ionizable lipids perturbs the membrane, RNA-bonded ionizable lipids scavenge H+ intended for acidification. Once the cargo is delivered, pH returns to 7, quenching the cationic charge and leaving the genetic material ready for action. Finally, it is also important to note the clear difference that exists typically in cellular uptake between an in vitro system and an in vivo system, since in vitro conditions, the limitation of a low charge at physiological pH is much more relaxed, allowing the use of ionizable lipids with higher pKa, which can give a higher efficiency than in vivo (3). The endosomal escape mechanism mediated by the iLNPs, is illustrated in Figure 4.
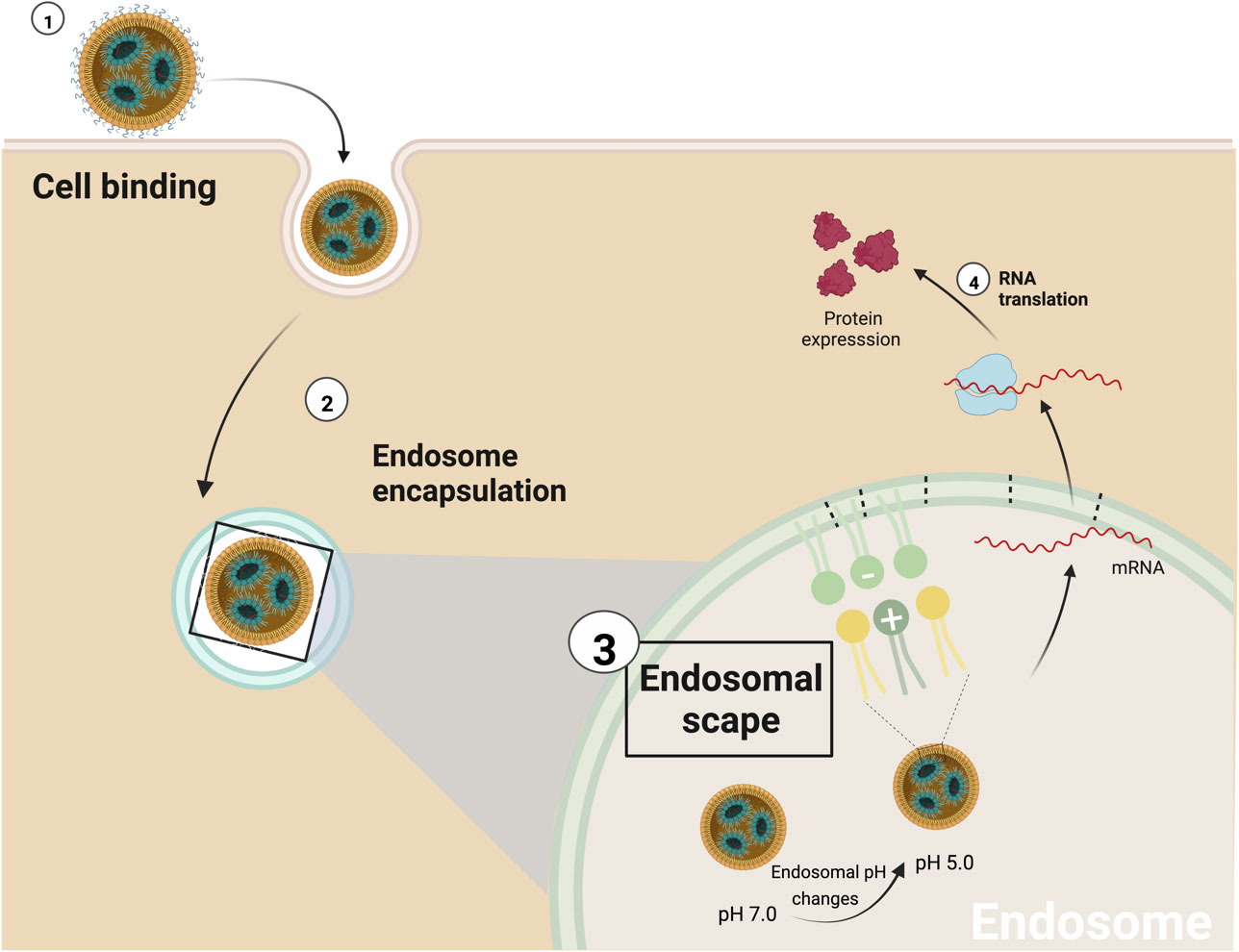
Figure 4 Schematic representation of endosomal scape mediated by iLNPs. 1) The first interaction between iLNPs and the target cell activates the endocytosis process, and 2) subsequently, the nanocarrier is encapsulated by the endosome. 3) As proton pumps reduce the pH of the endosome, the ionizable cationic lipid becomes progressively protonated (positively charged). As a result, it can interact with the endosome’s membrane anionic lipids to produce non-bilayer structures, disrupting the endosomal membrane and the iLNP structure and 4) releasing the mRNA into the cell cytoplasm.
Excretion of iLNPs
Once the NP disintegrates, its components have to be processed and excreted from the body. DSPC and Cholesterol are both part of cell membranes; therefore, degradation and metabolism of these products will occur integrated within the natural processes of the cell. Nucleases metabolize the delivered genetic material to nucleotides of various lengths. In the case of the ionizable lipids, the three (DLin-MC3-DMA, SM-102 and ALC-0315) present in the approved formulations have ester bonds, which are degraded by hydrolysis, allowing their degradation into different metabolites that are more easily excreted or harmless. The introduction of ester bonds, stable at physiological pH, which are hydrolyzed by enzymes once inside the cell or tissues, is a widely used strategy to increase the biodegradability of these lipids, reducing their accumulation and possible consequent side effects (52). DLin-MC3-DMA is primarily metabolized by hydrolysis to 4-dimethylaminobutyric acid (DMBA) and excreted from the body through the urine (ONPATRO Assessment report EMA/554262/2018). On the other hand, PEG2000-C-DMG seems not to be extensively metabolized and is suggested to be eliminated unchanged through the hepatobiliary tract to the feces (ONPATRO Assessment report EMA/554262/2018). In the case of ALC-0159, the PEG-lipid present in Comirnaty, the BioNtech Pfizer vaccine, the primary route of metabolism appears to be related to amide bond hydrolysis, yielding N,Nditetradecylamine (COMIRNATY Assessment report EMA/707383/2020).
Current challenges for ionizable lipid nanoparticles to be used in medicine
Regarding DDS, a common limitation is dosing. In systemic delivery, taking into account that there is a limitation of volume that can be injected in a single shot into the body (e.g. 10 mL/Kg for intravenous injection in mammals), the therapeutic dose might not be reached unless iLNPs have been previously concentrated because, during synthesis and storage, high concentrations lead to larger and polydisperse particles. Besides, in vitro simple tests, as those assessed in monolayer cell cultures, do not consider important factors such as organ vascularity, organ penetration and other differential properties given by the organ microenvironment (111), something that is not so critical for small molecule drugs but that is determinant for NP biodistribution and effects. Similarly, different in vivo models show variations between them, such as the different sizes of pores in vessels or different immunological responses, which may result in different efficacy for the same NPs depending on the model being used. Also, to be able to work with animals with implanted tumors, SCID (Severe Compromised Immunodeficiency) models are often used, which lack a fully functional immune system, significantly when its size ranges between virus and bacteria. In this context, 3D cell cultures have been proposed as suitable models to study the behavior of iLNPs in a particular environment due to the possibility of mimicking a controlled extracellular matrix and different organ regions (112).
Regarding the penetration and distribution inside organs, it is known that macromolecular carriers fail to penetrate deep into organs and tumors and are generally accumulated just some micrometers away from the vessels that transported them (113–115). Furthermore, solid tumor penetration is also challenging for small molecules (111). Finally, as carriers, they could carry the substance to the wrong place with high precision, producing unexpected side effects. Also, regarding safety, as the vehicle aims at universality, it will be applied repeatedly to the individual across their life, which may end up triggering pro-inflammatory immune responses towards the iLNPs or some of their components, for example, PEG (116, 117). Beyond parenteral application, in the following, we list some of the current iLNPs developments showing the universality of the vehicle’s ability to transport different sequences through different organs and portals of entry:
Oral ingestion
The most preferred administration route for medical treatments is oral delivery. Several studies suggest that the lipid nanoparticle composition enchases their biodisponibility in the gastrointestinal mucosae following the natural entrance in the digestion process (118). Additionally, studies on the siRNA- lipid nanoparticle stability showed that the LPNs remained potent and stable after exposure to solutions with pH values as low as 1.2. However, future research needs to increase cargo delivery and improve the effectiveness once mucin is present in the intestines (119). Interestingly, new research conducted by Sung et al. (120), demonstrates the efficiency deliver of IL-22 mRNA -loaded iLPS administrated by oral route in a mouse model of acute colitis. The results showed a high level of expression of interleukin 22, the recovery of body weight, and an accelerated healing process in the colon tissues.
Ocular applications
The main medicaments designed for ocular applications act in the anterior part of the eye. However, some degenerative processes, such as age-related macular degeneration, retinitis pigmentosa, and diabetic retinopathy, occur at the retina level in the posterior part of the eye. Here iLNPs provide sustained gene expression that could overcome these limitations (121). Recently, Wang and co-workers employed an iLNP to generate a cell-specific gene delivery system with sustained gene expression in the eye tissue (122).
Cutaneous application
As an external barrier, skin homeostasis is a complex process. Therefore, a broad spectrum of topical medication treats diverse cutaneous diseases. Herein, iLNPs are a promissory agent to improve drug penetration through the stratum corneum. Although to date, no iLNP has been reported for commercial cutaneous applications, the efficient encapsulation of distinct cosmetic agents such as oils, vitamins, and antimycotic and anti-age compounds are the most common pharmaceutical approximations (123). As an approach to treating a chronic wound, Gainza and co-workers loaded the recombinant human epidermal growth factor (rhEGF) into an iLNP showing an essential recovery in healing grade and wound maturity (124).
Conclusions and future perspectives
The iLNPs are a promissory concept to explore the efficient drug delivery of a broad range of substances, including genetic material, proteins and other NPs, decreasing collateral effects in healthy tissues and cells. However, despite the current knowledge on the subject being scattered and too heterogeneous, many recent discoveries and advances preclude the inevitable success of iLNPs. Indeed it has been predicted that Nanoparticulate DDS will be the most innovative and crucial cornerstones in pharmaceutical research, with a tremendous economic impact, where the possibility to adjust their physicochemical characteristics and increase the interaction with the target cell, and control the escape from the endosomal compartment allow for precision medicine. Thus, novel iLNPs will be developed with growing interest and improved pharmacokinetic profiles compared to standard drug delivery.
They are simple to produce, can load hydrophobic and hydrophilic substances, and are easily functionalizable with target moieties to ensure better precise delivery once an organ is reached. Indeed, encapsulating the genetic material into iLNPs is one of the fastest currently developing pharmaceutical technology. This success is the result of its clever design, which, in a biomimetic way, adapts to the different barriers and biological difficulties encountered along the way. All in all, in the near future, iLNPs may result in a quantum leap in medicine history.
Author contributions
RG-R, VS, NB, and VP contributed to the design and implementation of the bibliographic research, the analysis and discussion of the literature and the writing of the manuscript. All authors contributed to the article and approved the submitted version.
Acknowledgments
We acknowledge financial support from the Spanish Ministerio de Ciencia, Innovación y Universidades (MCIU) (RTI2018-099965-B-I00, AEI/FEDER,UE) proyectos de I+D+i de programación conjunta internacional MCIN/AEI (CONCORD, PCI2019-103436) cofunded by the European Union and Generalitat de Catalunya (2017-SGR-1431). ICN2 is supported by the Severo Ochoa program from Spanish MINECO (SEV-2017-0706) and is funded by the CERCA Programme/Generalitat de Catalunya.
Conflict of interest
The authors declare that the research was conducted in the absence of any commercial or financial relationships that could be construed as a potential conflict of interest.
Publisher’s note
All claims expressed in this article are solely those of the authors and do not necessarily represent those of their affiliated organizations, or those of the publisher, the editors and the reviewers. Any product that may be evaluated in this article, or claim that may be made by its manufacturer, is not guaranteed or endorsed by the publisher.
References
1. Ehrlich P. Experimental researches on specific therapy. In: The collected papers of Paul Ehrlich (1960) (Elsevier Science). p. 106–17.
2. Zipfel PF, Skerka C. From magic bullets to modern therapeutics: Paul Ehrlich, the German immunobiologist and physician coined the term 'complement'. Mol Immunol (2022) 150:90–8. doi: 10.1016/j.molimm.2022.08.002
3. Park K. Controlled drug delivery systems: Past forward and future back. J Control Release (2014) 190:3–8. doi: 10.1016/j.jconrel.2014.03.054
4. Gaynes R. The discovery of penicillin–new insights after more than 75 years of clinical use. Emerging Infect Dis (2017) 23(5):849–53. doi: 10.3201/eid2305.161556
5. Zhao N, Woodle MC, Mixson AJ. Advances in delivery systems for doxorubicin. J Nanomed Nanotechnol (2018) 9(5), 1000519. doi: 10.4172/2157-7439.1000519
6. Lipinski CA, Lombardo F, Dominy BW, Feeney PJ. Experimental and computational approaches to estimate solubility and permeability in drug discovery and development settings. Adv Drug Delivery Rev (2001) 46(1-3):3–26. doi: 10.1016/S0169-409X(00)00129-0
7. Das S, Jagan L, Isiah R, Rajesh B., Backianathan S., Subhashini J., et al. Nanotechnology in oncology: Characterization and in vitro release kinetics of cisplatin-loaded albumin nanoparticles: Implications in anticancer drug delivery. Indian J Pharmacol (2011) 43(4):409–13. doi: 10.4103/0253-7613.83111
8. Pelaz B, Alexiou C, Alvarez-Puebla RA, Alves F., Andrews A. M., Ashraf S., et al. Diverse applications of nanomedicine. ACS Nano (2017) 11(3):2313–81. doi: 10.1021/acsnano.6b06040
9. Jain PK, Huang X, El-Sayed IH, El-Sayed MA. Noble metals on the nanoscale: Optical and photothermal properties and some applications in imaging, sensing, biology, and medicine. Acc. Chem Res (2008) 41(12):1578–86. doi: 10.1021/ar7002804
10. Xia Y, Li W, Cobley CM, Chen J., Xia X., Zhang Q., et al. Gold nanocages: from synthesis to theranostic applications. Acc Chem Res (2011) 44(10):914–24. doi: 10.1021/ar200061q
11. Comenge J, Sotelo C, Romero F, Gallego O., Barnadas A., Parada T. G., et al. Detoxifying antitumoral drugs via nanoconjugation: The case of gold nanoparticles and cisplatin. PloS One (2012) 7(10):e47562. doi: 10.1371/journal.pone.0047562
12. Puntes VF, Comenge J. The role of PEG conformation in mixed layers: From protein corona substrate to steric stabilization avoiding protein adsorption. ScienceOpen Res (2015). doi: 10.14293/S2199-1006.1.SOR-MATSCI.A0Z6OM.v1
13. Daraee H, Etemadi A, Kouhi M, Alimirzalu S, Akbarzadeh A. Application of liposomes in medicine and drug delivery. Artif Cells Nanomed Biotechnol (2016) 44(1):381–91. doi: 10.3109/21691401.2014.953633
14. Garg J, Pathania K, Sah SP, Pawar SV. Nanostructured lipid carriers: a promising drug carrier for targeting brain tumours. Future J Pharm Sci (2022) 8(1), 1. doi: 10.1186/s43094-022-00414-8
15. Wang W, Zhang L, Chen T, Guo W., Bao X., Wang D., et al. Anticancer effects of resveratrol-loaded solid lipid nanoparticles on human breast cancer cells. Molecules (2017) 22(11):1814. doi: 10.3390/molecules22111814
16. Wang W, Chen T, Xu H, Ren B., Cheng X., Qi R., et al. Curcumin-loaded solid lipid nanoparticles enhanced anticancer efficiency in breast cancer. Molecules (2018) 23(7):1578. doi: 10.3390/molecules23071578
17. Mk D, Karthikeyan M, Ad A, Pc S., Sd O., Rc S., et al. Comprehensive review on solid lipid nanoparticles. Ann Pharmacol Pharmaceutics (2020) 5(4):1–6. doi: 10.47583/ijpsrr.2021.v67i01.027
18. Scioli Montoto S, Muraca G, Ruiz ME. Solid lipid nanoparticles for drug delivery: Pharmacological and biopharmaceutical aspects. Front Mol Biosci (2020) 7:587997. doi: 10.3389/fmolb.2020.587997
19. Mishra V, Bansal KK, Verma A, Yadav N., Thakur S., Sudhakar K., et al. Solid lipid nanoparticles: Emerging colloidal nano drug delivery systems. Pharmaceutics (2018) 10(4):191. doi: 10.3390/pharmaceutics10040191
20. Khosa A, Reddi S, Saha RN. Nanostructured lipid carriers for site-specific drug delivery. BioMed Pharmacother (2018) 103:598–613. doi: 10.1016/j.biopha.2018.04.055
21. Buck J, Grossen P, Cullis PR, Huwyler J, Witzigmann D. Lipid-based DNA therapeutics: Hallmarks of non-viral gene delivery. ACS Nano (2019) 13(4):3754–82. doi: 10.1021/acsnano.8b07858
22. Zhang C, Ma Y, Zhang J, Kuo J. C., Zhang Z., Xie H., et al. Modification of lipid-based nanoparticles: An efficient delivery system for nucleic acid-based immunotherapy. Molecules (2022) 27(6):943. doi: 10.3390/molecules27061943
23. Miele E, Spinelli GP, Miele E, Tomao F, Tomao S. Albumin-bound formulation of paclitaxel (Abraxane ABI-007) in the treatment of breast cancer. Int J Nanomedicine (2009) 4(1):99–105. doi: 10.2147/ijn.s3061
24. Shi J, Votruba AR, Farokhzad OC, Langer R. Nanotechnology in drug delivery and tissue engineering: from discovery to applications. Nano Lett (2010) 10(9):3223–30. doi: 10.1021/nl102184c
25. Hernandez-Giottonini KY, Rodriguez-Cordova RJ, Gutierrez-Valenzuela CA, Penunuri-Miranda O., Zavala-Rivera P., Guerrero-German P., et al. PLGA nanoparticle preparations by emulsification and nanoprecipitation techniques: effects of formulation parameters. RSC Adv (2020) 10(8):4218–31. doi: 10.1039/C9RA10857B
26. Zu H, Gao D. Non-viral vectors in gene therapy: Recent development, challenges, and prospects. AAPS J (2021) 23(4):78. doi: 10.1208/s12248-021-00608-7
27. Felgner PL, Gadek TR, Holm M, Roman R., Chan H. W., Wenz M., et al. Lipofection: a highly efficient, lipid-mediated DNA-transfection procedure. Proc Natl Acad Sci U.S.A. (1987) 84(21):7413–7. doi: 10.1073/pnas.84.21.7413
28. Tam YK, Madden TD, Hope MJ. Pieter cullis' quest for a lipid-based, fusogenic delivery system for nucleic acid therapeutics: success with siRNA so what about mRNA? J Drug Target (2016) 24(9):774–9. doi: 10.1080/1061186X.2016.1221955
29. Tan Y, Huang L. Overcoming the inflammatory toxicity of cationic gene vectors. J Drug Target (2002) 10(2):153–60. doi: 10.1080/10611860290016757
30. Zhang JS, Liu F, Huang L. Implications of pharmacokinetic behavior of lipoplex for its inflammatory toxicity. Advanced Drug Delivery Rev (2005) 57:689–98. doi: 10.1016/j.addr.2004.12.004
31. Semple SC, Klimuk SK, Harasym TO, Dos Santos N., Ansell S. M., Wong K. F., et al. Efficient encapsulation of antisense oligonucleotides in lipid vesicles using ionizable aminolipids: Formation of novel small multilamellar vesicle structures. Biochim Biophys Acta (2001) 1510(1-2):152–66. doi: 10.1016/S0005-2736(00)00343-6
32. Plank C, Mechtler K, Szoka FC Jr., Wagner E. Activation of the complement system by synthetic DNA complexes: a potential barrier for intravenous gene delivery. Hum Gene Ther (1996) 7(12):1437–46. doi: 10.1089/hum.1996.7.12-1437
33. Devine DV, Wong K, Serrano K, Chonn A, Cullis PR. Liposome-complement interactions in rat serum: Implications for liposome survival studies. Biochim Biophys Acta (1994) 1191(1):43–51. doi: 10.1016/0005-2736(94)90231-3
34. Marjan J, Xie Z, Devine DV. Liposome-induced activation of the classical complement pathway does not require immunoglobulin. Biochim Biophys Acta (1994) 1192(1):35–44. doi: 10.1016/0005-2736(94)90140-6
35. Fenton OS, Kauffman KJ, McClellan RL, Appel E. A., Dorkin J. R., Tibbitt M. W., et al. Bioinspired alkenyl amino alcohol ionizable lipid materials for highly potent in vivo mRNA delivery. Adv Mater (2016) 28(15):2939–43. doi: 10.1002/adma.201505822
36. Evers MJW, Kulkarni JA, van der Meel R, Cullis P. R., Vader P., Schiffelers R. M., et al. State-of-the-Art design and rapid-mixing production techniques of lipid nanoparticles for nucleic acid delivery. Small Methods (2018) 2(9):1700375. doi: 10.1002/smtd.201700375
37. Suzuki Y, Ishihara H. Difference in the lipid nanoparticle technology employed in three approved siRNA (Patisiran) and mRNA (COVID-19 vaccine) drugs. Drug Metab Pharmacokinet (2021) 41:100424. doi: 10.1016/j.dmpk.2021.100424
38. Guevara ML, Persano F, Persano S. Advances in lipid nanoparticles for mRNA-based cancer immunotherapy. Front Chem (2020) 8:589959. doi: 10.3389/fchem.2020.589959
39. Gomez-Aguado I, Rodriguez-Castejon J, Vicente-Pascual M, Rodriguez-Gascon A., Pozo-Rodriguez A. D., Solinis Aspiazu M. A., et al. Nucleic acid delivery by solid lipid nanoparticles containing switchable lipids: Plasmid DNA vs. messenger RNA. Molecules (2020) 25(24):5995. doi: 10.3390/molecules25245995
40. Hald Albertsen C, Kulkarni JA, Witzigmann D, Lind M., Petersson K., Simonsen J. B., et al. The role of lipid components in lipid nanoparticles for vaccines and gene therapy. Adv Drug Delivery Rev (2022) 188:114416. doi: 10.1016/j.addr.2022.114416
41. Kulkarni JA, Witzigmann D, Leung J, Tam YYC, Cullis PR. On the role of helper lipids in lipid nanoparticle formulations of siRNA. Nanoscale (2019) 11(45):21733–9. doi: 10.1039/C9NR09347H
42. Belliveau NM, Huft J, Lin PJ, Chen S., Leung A. K., Leaver T. J., et al. Microfluidic synthesis of highly potent limit-size lipid nanoparticles for in vivo delivery of siRNA. Mol Ther Nucleic Acids (2012) 1(8):e37. doi: 10.1038/mtna.2012.28
43. Kauffman KJ, Dorkin JR, Yang JH, Heartlein M. W., DeRosa F., Mir F. F., et al. Optimization of lipid nanoparticle formulations for mRNA delivery in vivo with fractional factorial and definitive screening designs. Nano Lett (2015) 15(11):7300–6. doi: 10.1021/acs.nanolett.5b02497
44. Jayaraman M, Ansell SM, Mui BL, Tam Y. K., Chen J., Du X., et al. Maximizing the potency of siRNA lipid nanoparticles for hepatic gene silencing in vivo. Angew Chem Int Ed Engl (2012) 51(34):8529–33. doi: 10.1002/anie.201203263
45. Akinc A, Maier MA, Manoharan M, Fitzgerald K., Jayaraman M., Barros S., et al. The onpattro story and the clinical translation of nanomedicines containing nucleic acid-based drugs. Nat Nanotechnol (2019) 14(12):1084–7. doi: 10.1038/s41565-019-0591-y
46. Maier MA, Jayaraman M, Matsuda S, Liu J., Barros S., Querbes W., et al. Biodegradable lipids enabling rapidly eliminated lipid nanoparticles for systemic delivery of RNAi therapeutics. Mol Ther (2013) 21(8):1570–8. doi: 10.1038/mt.2013.124
47. Whitehead KA, Dorkin JR, Vegas AJ, Chang P. H., Veiseh O., Matthews J., et al. Degradable lipid nanoparticles with predictable in vivo siRNA delivery activity. Nat Commun (2014) 5(1):4277. doi: 10.1038/ncomms5277
48. Hao J, Kos P, Zhou K, Miller J. B., Xue L., Yan Y., et al. Rapid synthesis of a lipocationic polyester library via ring-opening polymerization of functional valerolactones for efficacious siRNA delivery. J Am Chem Soc (2015) 137(29):9206–9. doi: 10.1021/jacs.5b03429
49. Semple SC, Akinc A, Chen J, Sandhu A. P., Mui B. L., Cho C. K., et al. Rational design of cationic lipids for siRNA delivery. Nat Biotechnol (2010) 28(2):172–6. doi: 10.1038/nbt.1602
50. Li AV, Moon JJ, Abraham W, Suh H., Elkhader J., Seidman M. A., et al. Generation of effector memory T cell-based mucosal and systemic immunity with pulmonary nanoparticle vaccination. Sci Transl Med (2013) 5(204):204ra130. doi: 10.1126/scitranslmed.3006516
51. Miller JB, Kos P, Tieu V, Zhou K, Siegwart DJ. Development of cationic quaternary ammonium sulfonamide amino lipids for nucleic acid delivery. ACS Appl Mater Interfaces (2018) 10(3):2302–11. doi: 10.1021/acsami.7b15982
52. Han X, Zhang H, Butowska K, Swingle K. L., Alameh M. G., Weissman D., et al. An ionizable lipid toolbox for RNA delivery. Nat Commun (2021) 12(1):7233. doi: 10.1038/s41467-021-27493-0
53. Crawford R, Dogdas B, Keough E, Haas R. M., Wepukhulu W., Krotzer S., et al. Analysis of lipid nanoparticles by cryo-EM for characterizing siRNA delivery vehicles. Int J Pharm (2011) 403(1-2):237–44. doi: 10.1016/j.ijpharm.2010.10.025
54. Chen C, Chen C, Li Y, Gu R, Yan X. Characterization of lipid-based nanomedicines at the single-particle level. Fundam Res (2022). doi: 10.1016/j.fmre.2022.09.011
55. Bunjes H, Unruh T. Characterization of lipid nanoparticles by differential scanning calorimetry, X-ray and neutron scattering. Advanced Drug Delivery Rev (2007) 59:379–402. doi: 10.1016/j.addr.2007.04.013
56. Schilt Y, Berman T, Wei X, Barenholz Y, Raviv U. Using solution X-ray scattering to determine the high-resolution structure and morphology of PEGylated liposomal doxorubicin nanodrugs. Biochim Biophys Acta (2016) 1860(1 Pt A):108–19. doi: 10.1016/j.bbagen.2015.09.012
57. Dong YD, Boyd BJ. Applications of X-ray scattering in pharmaceutical science. Int J Pharm (2011) 417(1-2):101–11. doi: 10.1016/j.ijpharm.2011.01.022
58. Pabst G, Kucerka N, Nieh MP, Rheinstadter MC, Katsaras J. Applications of neutron and X-ray scattering to the study of biologically relevant model membranes. Chem Phys Lipids (2010) 163(6):460–79. doi: 10.1016/j.chemphyslip.2010.03.010
59. Di Cola E, Grillo I, Ristori S. Small angle X-ray and neutron scattering: Powerful tools for studying the structure of drug-loaded liposomes. Pharmaceutics (2016) 8(2):10. doi: 10.3390/pharmaceutics8020010
60. Cui L, Renzi S, Quagliarini E, Digiacomo L., Amenitsch H., Masuelli L., et al. Efficient delivery of DNA using lipid nanoparticles. Pharmaceutics (2022) 14(8):1698. doi: 10.3390/pharmaceutics14081698
61. Viger-Gravel J, Schantz A, Pinon AC, Rossini A. J., Schantz S., Emsley L., et al. Structure of lipid nanoparticles containing siRNA or mRNA by dynamic nuclear polarization-enhanced NMR spectroscopy. J Phys Chem B (2018) 122(7):2073–81. doi: 10.1021/acs.jpcb.7b10795
62. Martinez-Negro M, Kumar K, Barran-Berdon AL, Datta S., Kondaiah P., Junquera E., et al. Efficient cellular knockdown mediated by siRNA nanovectors of Gemini cationic lipids having delocalizable headgroups and oligo-oxyethylene spacers. ACS Appl Mater Interfaces (2016) 8(34):22113–26. doi: 10.1021/acsami.6b08823
63. Yanez Arteta M, Kjellman T, Bartesaghi S, Wallin S., Wu X., Kvist A. J., et al. Successful reprogramming of cellular protein production through mRNA delivered by functionalized lipid nanoparticles. Proc Natl Acad Sci U.S.A. (2018) 115(15):E3351–60. doi: 10.1073/pnas.1720542115
64. Zak MM, Zangi L. Lipid nanoparticles for organ-specific mRNA therapeutic delivery. Pharmaceutics (2021) 13(10):1675. doi: 10.3390/pharmaceutics13101675
65. Cho EC, Zhang Q, Xia Y. The effect of sedimentation and diffusion on cellular uptake of gold nanoparticles. Nat Nanotechnol (2011) 6(6):385–91. doi: 10.1038/nnano.2011.58
66. Krug HF. Nanosafety research–are we on the right track? Angew Chem Int Ed Engl (2014) 53(46):12304–19. doi: 10.1002/anie.201403367
67. Bian SW, Mudunkotuwa IA, Rupasinghe T, Grassian VH. Aggregation and dissolution of 4 nm ZnO nanoparticles in aqueous environments: influence of pH, ionic strength, size, and adsorption of humic acid. Langmuir (2011) 27(10):6059–68. doi: 10.1021/la200570n
68. Xia Y, Tian J, Chen X. Effect of surface properties on liposomal siRNA delivery. Biomaterials (2016) 79:56–68. doi: 10.1016/j.biomaterials.2015.11.056
69. Harvie P, Wong FMP, Bally MB. Use of poly(ethylene glycol)-lipid conjugates to regulate the surface attributes and transfection activity of lipid-DNA particles. J Pharm Sci (2000) 89:652–63. doi: 10.1002/(SICI)1520-6017(200005)89:5<652::AID-JPS11>3.0.CO;2-H
70. Song L. Y., Ahkong Q. F., Rong Q., Wang Z., Ansell S., Hope M. J., et al. Characterization of the inhibitory effect of PEG-lipid conjugates on the intracellular delivery of plasmid and antisense DNA mediated by cationic lipid liposomes. Biochim Biophys Acta (2002) 1558(1):1–13. doi: 10.1016/S0005-2736(01)00399-6
71. Mui BL, Tam YK, Jayaraman M, Ansell S. M., Du X., Tam Y. Y., et al. Influence of polyethylene glycol lipid desorption rates on pharmacokinetics and pharmacodynamics of siRNA lipid nanoparticles. Mol Ther Nucleic Acids (2013) 2(12):e139. doi: 10.1038/mtna.2013.66
72. Hashiba K, Sato Y, Harashima H. pH-labile PEGylation of siRNA-loaded lipid nanoparticle improves active targeting and gene silencing activity in hepatocytes. J Control Release (2017) 262:239–46. doi: 10.1016/j.jconrel.2017.07.046
73. Francia V, Schiffelers RM, Cullis PR, Witzigmann D. The biomolecular corona of lipid nanoparticles for gene therapy. Bioconjug Chem (2020) 31(9):2046–59. doi: 10.1021/acs.bioconjchem.0c00366
74. Walczyk D, Bombelli FB, Monopoli MP, Lynch I, Dawson KA. What the cell "sees" in bionanoscience. J Am Chem Soc (2010) 132(16):5761–8. doi: 10.1021/ja910675v
75. Monopoli MP, Aberg C, Salvati A, Dawson KA. Biomolecular coronas provide the biological identity of nanosized materials. Nat Nanotechnol (2012) 7(12):779–86. doi: 10.1038/nnano.2012.207
76. Cai R, Chen C. The crown and the scepter: Roles of the protein corona in nanomedicine. Adv Mater (2019) 31(45):e1805740. doi: 10.1002/adma.201805740
77. Nguyen VH, Lee BJ. Protein corona: A new approach for nanomedicine design. Int J Nanomedicine (2017) 12:3137–51. doi: 10.2147/IJN.S129300
78. Casals E, Pfaller T, Duschl A, Oostingh GJ, Puntes V. Time evolution of the nanoparticle protein corona. ACS Nano (2010) 4(7):3623–32. doi: 10.1021/nn901372t
79. Akinc A, Querbes W, De S, Qin J., Frank-Kamenetsky M., Jayaprakash K. N., et al. Targeted delivery of RNAi therapeutics with endogenous and exogenous ligand-based mechanisms. Mol Ther (2010) 18(7):1357–64. doi: 10.1038/mt.2010.85
80. Qiu M, Tang Y, Chen J, Muriph R., Ye Z., Huang C., et al. Lung-selective mRNA delivery of synthetic lipid nanoparticles for the treatment of pulmonary lymphangioleiomyomatosis. Proc Natl Acad Sci U.S.A. (2022) 119(8):e2116271119. doi: 10.1073/pnas.2116271119
81. Rejman J, Oberle V, Zuhorn IS, Hoekstra D. Size-dependent internalization of particles via the pathways of clathrin- and caveolae-mediated endocytosis. Biochem J (2004) 377(Pt 1):159–69. doi: 10.1042/bj20031253
82. Geranio L, Heuberger M, Nowack B. The behavior of silver nanotextiles during washing. Environ Sci Technol (2009) 43(21):8113–8. doi: 10.1021/es9018332
83. Elzey S, Grassian VH. Agglomeration, isolation and dissolution of commercially manufactured silver nanoparticles in aqueous environments. J Nanoparticle Res (2009) 12(5):1945–58. doi: 10.1007/s11051-009-9783-y
84. Li X, Lenhart JJ, Walker HW. Aggregation kinetics and dissolution of coated silver nanoparticles. Langmuir (2012) 28(2):1095–104. doi: 10.1021/la202328n
85. Li X, Lenhart JJ, Walker HW. Dissolution-accompanied aggregation kinetics of silver nanoparticles. Langmuir (2010) 26(22):16690–8. doi: 10.1021/la101768n
86. Liu J, Hurt RH. Ion release kinetics and particle persistence in aqueous nano-silver colloids. Environ Sci Technol (2010) 44(6):2169–75. doi: 10.1021/es9035557
87. Hwang J, Sullivan MO, Kiick KL. Targeted drug delivery via the use of ECM-mimetic materials. Front Bioeng Biotechnol (2020) 8:69. doi: 10.3389/fbioe.2020.00069
88. Henke E, Nandigama R, Ergün S. Extracellular matrix in the tumor microenvironment and its impact on cancer therapy. Front Mol Biosci (2020) 6. doi: 10.3389/fmolb.2019.00160
89. Ernst LM, Casals E, Italiani P, Boraschi D, Puntes V. The interactions between nanoparticles and the innate immune system from a nanotechnologist perspective. Nanomaterials (Basel) (2021) 11(11):2991. doi: 10.3390/nano11112991
90. Arana L, Bayon-Cordero L, Sarasola LI, Berasategi M., Ruiz S., Alkorta I., et al. Solid lipid nanoparticles surface modification modulates cell internalization and improves chemotoxic treatment in an oral carcinoma cell line. Nanomaterials (Basel) (2019) 9(3):464. doi: 10.3390/nano9030464
91. Sebak AA. Limitations of pegylated nanocarriers: Unfavourable physicochemical properties, biodistribution patterns and cellular and subcellular fates. Int J Appl Pharmaceutics (2018) 10(5):6–12. doi: 10.22159/ijap.2018v10i5.27568
92. Hoang Thi TT, Pilkington EH, Nguyen DH, Lee J. S., Park K. D., Truong N. P., et al. The importance of poly(ethylene glycol) alternatives for overcoming PEG immunogenicity in drug delivery and bioconjugation. Polymers (Basel) (2020) 12(2):298. doi: 10.3390/polym12020298
93. Goy-Lopez S, Juarez J, Alatorre-Meda M, Casals E., Puntes V. F., Taboada P., et al. Physicochemical characteristics of proteina-NP bioconjugates: the role of particle curvature and solution conditions on human serum albumin conformation and fibrillogenesis inhibition. Langmuir (2012) 28(24):9113–26. doi: 10.1021/la300402w
94. Ojea-Jimenez I, Puntes V. Instability of cationic gold nanoparticle bioconjugates: the role of citrate ions. J Am Chem Soc (2009) 131(37):13320–7. doi: 10.1021/ja902894s
95. Casals E, Barrena R, Garcia A, Gonzalez E., Delgado L., Busquets-Fite M., et al. Programmed iron oxide nanoparticles disintegration in anaerobic digesters boosts biogas production. Small (2014) 10(14):2801–8, 2741. doi: 10.1002/smll.201303703
96. Bastus NG, Casals E, Vázquez-Campos S, Puntes V. Reactivity of engineered inorganic nanoparticles and carbon nanostructures in biological media. Nanotoxicology (2008) 2(3):99–112. doi: 10.1080/17435390802217830
97. Barua S, Mitragotri S. Challenges associated with penetration of nanoparticles across cell and tissue barriers: A review of current status and future prospects. Nano Today (2014) 9(2):223–43. doi: 10.1016/j.nantod.2014.04.008
98. Dal Magro R, Ornaghi F, Cambianica I, Beretta S., Re F., Musicanti C., et al. ApoE-modified solid lipid nanoparticles: A feasible strategy to cross the blood-brain barrier. J Control Release (2017) 249:103–10. doi: 10.1016/j.jconrel.2017.01.039
99. Carlander U, Li D, Jolliet O, Emond C, Johanson G. Toward a general physiologically-based pharmacokinetic model for intravenously injected nanoparticles. Int J Nanomedicine (2016) 11:625–40. doi: 10.2147/IJN.S94370
100. Maeda H, Wu J, Sawa T, Matsumura Y, Hori K. Tumor vascular permeability and the EPR effect in macromolecular therapeutics: a review. J Control Release (2000) 65(1-2):271–84. doi: 10.1016/S0168-3659(99)00248-5
101. Martin F, Huang A, Uziely B, Kaufman B., Safra T., Gabizon A., et al. Prolonged circulation time and enhanced accumulation in malignant exudates of doxorubicin encapsulated in polyethylene-glycol coated liposomes. Cancer Researc (1994) 54(4):987–92.
102. Safra T, Muggia F, Jeffers S, Tsao-Wei D. D., Groshen S., Lyass O., et al. Pegylated liposomal doxorubicin (doxil): Reduced clinical cardiotoxicity in patients reaching or exceeding cumulative doses of 500 mg/m2. Ann Oncol (2000) 11(8):1029–33. doi: 10.1023/A:1008365716693
103. Gabizon A, Shmeeda H, Barenholz Y. Pharmacokinetics of pegylated liposomal doxorubicin: Review of animal and human studies. Clin Pharmacokinet (2003) 42(5):419–36. doi: 10.2165/00003088-200342050-00002
104. Brusini R, Varna M, Couvreur P. Advanced nanomedicines for the treatment of inflammatory diseases. Adv Drug Delivery Rev (2020) 157:161–78. doi: 10.1016/j.addr.2020.07.010
105. Rennick JJ, Johnston APR, Parton RG. Key principles and methods for studying the endocytosis of biological and nanoparticle therapeutics. Nat Nanotechnol (2021) 16(3):266–76. doi: 10.1038/s41565-021-00858-8
106. Herrera M, Kim J, Eygeris Y, Jozic A, Sahay G. Illuminating endosomal escape of polymorphic lipid nanoparticles that boost mRNA delivery. Biomater Sci (2021) 9(12):4289–300. doi: 10.1039/D0BM01947J
107. Hafez IM, Maurer N, Cullis PR. On the mechanism whereby cationic lipids promote intracellular delivery of polynucleic acids. Gene Ther (2001) 8(15):1188–96. doi: 10.1038/sj.gt.3301506
108. Miao L, Lin J, Huang Y, Li L., Delcassian D., Ge Y., et al. Synergistic lipid compositions for albumin receptor mediated delivery of mRNA to the liver. Nat Commun (2020) 11(1):2424. doi: 10.1038/s41467-020-16248-y
109. Cullis PR, Hope MJ. Lipid nanoparticle systems for enabling gene therapies. Mol Ther (2017) 25(7):1467–75. doi: 10.1016/j.ymthe.2017.03.013
110. Behr J-P. The proton sponge: A trick to enter cells the viruses did not exploit. Chimia (1997) 51(1-2):34. doi: 10.2533/chimia.1997.34
111. Tredan O, Galmarini CM, Patel K, Tannock IF. Drug resistance and the solid tumor microenvironment. J Natl Cancer Inst (2007) 99(19):1441–54. doi: 10.1093/jnci/djm135
112. Papi M, Pozzi D, Palmieri V, Caracciolo G. Principles for optimization and validation of mRNA lipid nanoparticle vaccines against COVID-19 using 3D bioprinting. Nano Today (2022) 43:101403. doi: 10.1016/j.nantod.2022.101403
113. Ruenraroengsak P, Cook JM, Florence AT. Nanosystem drug targeting: Facing up to complex realities. J Control Release (2010) 141(3):265–76. doi: 10.1016/j.jconrel.2009.10.032
114. Perrault SD, Walkey C, Jennings T, Fischer HC, Chan WC. Mediating tumor targeting efficiency of nanoparticles through design. Nano Lett (2009) 9(5):1909–15. doi: 10.1021/nl900031y
115. Minchinton AI, Tannock IF. Drug penetration in solid tumours. Nat Rev Cancer (2006) 6(8):583–92. doi: 10.1038/nrc1893
116. Chen BM, Cheng TL, Roffler SR. Polyethylene glycol immunogenicity: Theoretical, clinical, and practical aspects of anti-polyethylene glycol antibodies. ACS Nano (2021) 15(9):14022–48. doi: 10.1021/acsnano.1c05922
117. Kong YW, Dreaden EC. PEG: Will it come back to you? polyethelyne glycol immunogenicity, COVID vaccines, and the case for new PEG derivatives and alternatives. Front Bioeng Biotechnol (2022) 10:879988. doi: 10.3389/fbioe.2022.879988
118. Talegaonkar S, Bhattacharyya A. Potential of lipid nanoparticles (SLNs and NLCs) in enhancing oral bioavailability of drugs with poor intestinal permeability. AAPS PharmSciTech (2019) 20(3):121. doi: 10.1208/s12249-019-1337-8
119. Ball RL, Bajaj P, Whitehead KA. Oral delivery of siRNA lipid nanoparticles: Fate in the GI tract. Sci Rep (2018) 8(1):2178. doi: 10.1038/s41598-018-20632-6
120. Sung J, Alghoul Z, Long D, Yang C, Merlin D. Oral delivery of IL-22 mRNA-loaded lipid nanoparticles targeting the injured intestinal mucosa: A novel therapeutic solution to treat ulcerative colitis. Biomaterials (2022) 288(August):121707. doi: 10.1016/j.biomaterials.2022.121707
121. Wang Y, Rajala A, Rajala RV. Lipid nanoparticles for ocular gene delivery. J Funct Biomater (2015) 6(2):379–94. doi: 10.3390/jfb6020379
122. Toualbi L, Toms M, Moosajee M. The landscape of non-viral gene augmentation strategies for inherited retinal diseases. Int J Mol Sci (2021) 22(5):1–14. doi: 10.3390/ijms22052318
123. Garces A, Amaral MH, Sousa Lobo JM, Silva AC. Formulations based on solid lipid nanoparticles (SLN) and nanostructured lipid carriers (NLC) for cutaneous use: A review. Eur J Pharm Sci (2018) 112(September 2017):159–67. doi: 10.1016/j.ejps.2017.11.023
124. Gainza G, Bonafonte DC, Moreno B, Aguirre J. J., Gutierrez F. B., Villullas S., et al. The topical administration of rhEGF-loaded nanostructured lipid carriers (rhEGF-NLC) improves healing in a porcine full-thickness excisional wound model. J Control Release (2015) 197:41–7. doi: 10.1016/j.jconrel.2014.10.033
Keywords: ionizable lipid nanoparticles, RNA-loading, drug delivery carriers, pharmacokinetics, biodistribution and clearance
Citation: González-Rioja R, Salazar VA, Bastús NG and Puntes V (2023) The development of highly dense highly protected surfactant ionizable lipid RNA loaded nanoparticles. Front. Immunol. 14:1129296. doi: 10.3389/fimmu.2023.1129296
Received: 21 December 2022; Accepted: 14 February 2023;
Published: 27 February 2023.
Edited by:
Kirill Afonin, University of North Carolina at Charlotte, United StatesReviewed by:
Paolo Macor, University of Trieste, ItalyBorislav Angelov, Institute of Physics (ASCR), Czechia
Copyright © 2023 González-Rioja, Salazar, Bastús and Puntes. This is an open-access article distributed under the terms of the Creative Commons Attribution License (CC BY). The use, distribution or reproduction in other forums is permitted, provided the original author(s) and the copyright owner(s) are credited and that the original publication in this journal is cited, in accordance with accepted academic practice. No use, distribution or reproduction is permitted which does not comply with these terms.
*Correspondence: Victor Puntes, victor.puntes@icn2.cat