- 1National Clinical Research Center for Metabolic Diseases, Key Laboratory of Diabetes Immunology, Ministry of Education, and Department of Metabolism and Endocrinology, The Second Xiangya Hospital of Central South University, Changsha, China
- 2Section on Hematopoiesis and Lymphocyte Biology, Eunice Kennedy Shriver National Institute of Child Health and Human Development, National Institutes of Health, Bethesda, MD, United States
T cell development in the thymus is tightly controlled by complex regulatory mechanisms at multiple checkpoints. Currently, many studies have focused on the transcriptional and posttranslational control of the intrathymic journey of T-cell precursors. However, over the last few years, compelling evidence has highlighted cell metabolism as a critical regulator in this process. Different thymocyte subsets are directed by distinct metabolic pathways and signaling networks to match the specific functional requirements of the stage. Here, we epitomize these metabolic alterations during the development of a T cell and review several recent works that provide insights into equilibrating metabolic quiescence and activation programs. Ultimately, understanding the interplay between cellular metabolism and T cell developmental programs may offer an opportunity to selectively regulate T cell subset functions and to provide potential novel therapeutic approaches to modulate autoimmunity.
Introduction
T cell development is tightly regulated by multiple checkpoints and proliferative events before the emergence of naive T cells from the thymus (1–3). Early thymic progenitors (ETPs), also known as double-negative 1 (DN1) cells, differentiate into DN2 thymocytes in the thymic parenchyma, gaining T-lineage commitment (4, 5). A paramount event in T cell development called β-selection occurs during the DN3 stage, and thymocytes that successfully assemble the pre-T cell receptor (TCR) hasten to the DN4 phase and initiate rapid cell cycling governed by complex regulatory metabolism (6, 7). Additionally, γδ and αβ T cell lineages diverge at the DN3 stage (8, 9). Thymocytes return to a quiescence state in double-positive (DP) stage, undergoing positive/negative selection and DP to single-positive (SP) transition before becoming mature CD4+ or CD8+ T cells (3, 10, 11).
Cellular metabolism integrates multiple pathways and large networks of chemical reactions, and plays a critical role in regulating almost all cellular processes (12). Different thymocyte subsets have distinct metabolic patterns tailored to meet the bioenergetic demands required at each stage (13–15). In summary, catabolic metabolism of amino acids and glucose promotes the energy and biosynthesis that quiescent thymocytes require, whereas the transition from resting cells into highly activated phenotypes requires substantial metabolic reprogramming comprising aerobic glycolysis (Warburg effect), glutaminolysis, and mitochondrial biogenesis, which expedites oxidative phosphorylation (OXPHOS) and one-carbon metabolism (10, 15–17). These metabolic alterations constitute complex signaling mechanisms that connect external signals with transcriptional events and fate verdicts (17, 18). Here, we summarize recent findings on the metabolic control of T cell development, and highlight the roles of cell-intrinsic and cell-extrinsic metabolic factors involved in these processes.
Thymocytes are relatively quiescent before β-selection
ETPs settling in the thymus are quiescent before the first run of proliferation (11). Cytokines, such as Interleukin-7 (IL-7), Kit, and C-X-C motif chemokine receptor 4 (CXCR4), as well as Notch and Wnt signaling, account for the proliferation of thymocytes before β-selection (6, 19–29). During the four DN stages, Notch and IL-7 signaling drive the maturation of thymocytes and regulate cellular metabolism by interacting with the phosphatidylinositol-3-kinase/protein kinase B/mammalian target of rapamycin (PI3K/Akt/mTOR) signaling pathway (18, 21, 30–38). Before accelerating the multiplication at the DN3b stage, thymocytes are quiescent and primarily rely on OXPHOS to maintain bioenergetic homeostasis. AMP-activated protein kinase (AMPK) is a major regulator of metabolism that senses bioenergetic undulation and maintains energy homeostasis in cells (39–41). AMPK works in concert with liver kinase B1 (LKB1) to suppress biosynthesis and energy production, such as glycolysis and lipid synthesis, to restrain the anabolic growth of thymocytes (41–43). Loss of LKB1 leads to thymocyte developmental block and reduction of peripheral T cells (44, 45). Fatty acid-binding protein 3 (FABP3) regulates cellular lipid metabolism by binding to polyunsaturated fatty acids (PUFAs), such as ω-6 PUFAs. Recently, it has been shown that loss of FABP3 redirects DN2 thymocytes to pathogenic Vγ4+ γδ T cells (46). Mitochondrial metabolism also plays a role in thymocyte development. For example, the Janus mitochondrial protein apoptosis-inducing factor (AIF) has been shown to affect thymocyte transitions from DN1 to DN4 by modulating mitochondrial metabolism (47).
β-selected thymocytes exhibit robust cell proliferation and metabolic reprogramming
Transition from the DN3 to the immature single-positive (ISP) phase, thymocytes undergo V(D)J rearrangement and β-selection. This exclusive selection event then gives rise to robust cell growth and proliferation in which cells have to thoroughly alter their metabolism to meet the increased energy demand. Energy generation by cycling thymocytes after β-selection is largely dependent on aerobic glycolytic metabolism for prioritizing efficient and rapid biosynthesis of intracellular constituents, including nucleic acids and lipids (3, 11). The metabolic switch is mainly triggered by pre-TCR signaling (6, 48–50). Signaling of pre-TCR, Notch, and IL-7 converges to activate PI3K signaling, which stimulates the transition to anabolic metabolism, especially glycolysis. Glucose transporter type 1 (Glut1), an important glucose transporter, is induced at these phases, and its expression level is dependent on the activation of PI3K-Akt signaling (51–54).
The PI3K-phosphoinositol-dependent protein kinase-1 (PDK1)-Akt axis plays a critical role in thymocyte maturation (55, 56). In addition to their well-described role in protein synthesis via mTOR signaling, PI3K dominates aerobic glycolysis and glucose metabolism in a variety of biological processes (57, 58). PDK1 regulates the expression of key amino acid and iron transporters and controls the switch of glucose metabolism from aerobic oxidation to glycolysis in the thymus (55, 59). Loss of PDK1 impairs nutrient receptor expression and hence renders metabolically deficient to meet the energy demands from the DN to DP stage transition (55, 60). Furthermore, Akt signaling is a major stimulus of anabolism (21, 61, 62). Deletion of Akt alters thymocyte subsets with a development blocked after DN3 stage (56, 63). Similar to AKT, PIM kinases are also linchpins that regulate the expansion of thymocytes undergoing β-selection (64–66). Of note, the lipid kinase inositol-trisphosphate 3-kinase B (Itpkb) affects β-selection by restricting metabolic activation in DN3 thymocytes. The deficiency of Itpkb leads to accelerated development from DN3 cells to DP cells by activating Akt-mTOR signaling and breaking the balance between Notch and pre-TCR signaling (67).
As a master regulator of cell growth and metabolism, mTOR regulates multiple metabolic pathways, such as glutaminolysis, glycolysis, mitochondrial biogenesis, and protein synthesis (68, 69). It has been shown that mTOR signaling regulates thymocyte proliferation, anabolism, and development via integrated signals from TCRs, costimulatory molecules, cytokines, and nutrients (70–72). mTOR forms two structurally and functionally distinct complexes, mTOR complex 1 (mTORC1) and mTORC2 (71). Specifically, mTORC1 could integrate TCR and Notch signaling and induce the expression of transcription factors such as cellular myelocytomatosis oncogene (c-Myc) and Sterol-regulatory element binding proteins (SREBPs) for lipid synthesis and ROS production (71, 73). It has been reported that mTORC1 is involved in the reciprocal development of two fundamentally distinct T cell lineages, αβ and γδ T thymocytes (70). Loss of Raptor-mediated mTORC1 activity impairs the development of αβ T cells but promotes γδ T cell generation (70, 74). In addition, hypoxia-inducible factor 1-α (HIF1α) induced by mTORC1 signaling could increase glycolysis metabolism and the pentose phosphate pathway by controlling the production of glycolytic cascade members such as hexokinase 2 (HK2) and PDK1 (75–78). On the other hand, mTORC2 regulates glycolysis by activating Akt (61, 63, 79). Deletion of the mTORC2 component Rictor leads to thymocyte developmental blocks at the ISP phase, resulting in a reduction of DP cells (80, 81). Thymocyte specific ablation of Sin1, an important component of mTORC2, leads to developmental block at the DN3 to DN4 transition due to impaired proliferation and reduced expression of the glycolytic enzyme pyruvate kinase M2 (PKM2) through mTORC2-peroxisome proliferator-activated receptor γ (PPAR-γ)-PKM2 axes (82, 83). Taken together, the distinct regulation of thymocyte metabolism between mTORC1 and mTORC2 warrants further biological validation.
Myc expression is transcriptionally induced in thymocytes to facilitate the developmental progression from the DN to the DP stage (84–87). As Myc downstream target genes, the thioredoxin-1 (Trx1) system is a biosensor of glucose and energy metabolism that maintains cellular redox balance. Recent data from an animal study have shown that deletion of thioredoxin reductase-1 (Txnrd1), which is critical for the last step of nucleotide biosynthesis, precludes the expansion of DN cells (88). c-Myc gene expression is also regulated by bromodomain protein 4 (BRD4), which is a transcriptional and epigenetic regulator with functions throughout the cell cycle for proliferative regulation (89–93). It has been shown that BRD4 governs the development and differentiation of ISP thymocytes by modulating metabolic pathways and cell cycle progression (94). Deletion of BRD4 in ISP cells leads to a block in the transition to the DP phase, as well as inhibition of glycolysis (94). The mitochondrial protein Optic atrophy 1 (Opa1) was demonstrated to regulate OXPHOS and was required for thymocyte development during β-selection at the DN3 stage (37). The absence of Opa1 damages cellular respiration and induces apoptotic cell death. Mothe-Satney and collaborators found that overexpression of the transcription factor peroxisome proliferator-activated receptor β (PPARβ) increases fatty acid oxidation instead of glucose oxidation, hence restricting the expansion of DN4 cells (95). Furthermore, Zhao et al. demonstrated that PPARβ regulates the expression of the key genes and enzymes in glycolysis, oxidative phosphorylation, and lipogenesis in β-selected thymocytes. PPARβmut mice exhibited a reduction in thymocyte cell number starting at the DN4 stage (96). Recent laboratory work demonstrated that depletion of mitochondrial pyruvate carrier (MPC) 1, an MPC transporter subunit responsible for moving pyruvate into mitochondria, led to impaired β-selection and decreased αβT cells due to upregulated glycolysis and reduced OXPHOS (97). MicroRNAs and other noncoding RNAs have also been documented to be involved in T cell development as regulators of anabolism and catabolism (98–103). For example, as an important regulator of PI3K, miR-181 has been shown to regulate T cell development, including conventional and unconventional T cells (104–108). The transition from the DN to DP stage was severely impaired in miR-181-deficient mice (109). Single-cell RNA data showed that key genes of the glycolytic, pentose phosphate, and nucleotide biosynthetic pathways were deregulated in miR-181a1b1-deficient DP cells (104). Furthermore, miR-146a, a suppressor of nuclear factor-kappa B (NF-κB) signaling, participates in the regulation of thymocyte positive selection and amino acid metabolism (110).
Metabolic programming has been proposed to govern distinct immune cell lineages and functions, whereas γδ T cell metabolism is still poorly understood (70, 111). γδ T cells express mature γδTCR complex and undergo extensive proliferation comparable to αβ T cells (112–114). Unlike conventional T cells that exit the thymus as naïve T cells and further differentiate in peripheral organs upon activation, a large portion of γδ T cells commits to producing either IL-17 or IFN-γ during the development in the thymus, called the IL-17-producing γδ T cell (γδT17) and the IFN-γ-producing γδ T cell (γδT1), respectively (115–117). The two have intrinsically different metabolic requirements. Notably, the TCRγδ signaling appears to be more favorable for the metabolic transition of thymic γδ progenitors to γδT1 cells that are highly glycolytic. In contrast, γδT17 mainly engages OXPHOS (118). The metabolic dichotomy established in the thymus has a significant impact on the expansion and function of γδT1/17 cells, which could be used in tumor and autoimmune disease therapies (118–123). Yang et al. demonstrated that both γδT1 and γδT17 cells require mTORC1 for proliferation and survival, whereas mTORC2 is only essential for γδT17 cells. In Raptor KO mice, γδT17 differentiation was impaired due to suppressed glycolysis. In contrast, mTORC2 potentiated γδT17 induction by reducing mitochondrial ROS production (122). Moreover, nitric oxide synthase 2 (NOS2) deficient mice exhibited substantially reduced glycolysis and proliferation of γδ T cells (124). Glutaminase 1-mediated glutaminolysis was aberrantly activated and promoted γδT17 differentiation, thereby resulting in the development and immune imbalance of psoriasis (125). A recent study revealed a key role of c-Maf in regulating the function of γδT17 effectors through IDH2-mediated metabolic reprogramming (126). In conclusion, thymocyte development is orchestrated by these key metabolic regulators in the thymus.
Thymocytes return to a resting state from the small DP stage to CD4 or CD8 SP stage
After massive proliferation, DP thymocytes return to a resting state and initiate rearrangement of the Tcra gene for positive and negative selection. When quiescence occurs along a continuum as thymocytes differentiate from DP cells into SP cells, glucose metabolism must drastically decrease and revert to mitochondrial oxidative metabolism for maximal ATP generation (10, 127). Key metabolic regulators, such as c-Myc and HIF1α, need to be downregulated to safeguard the transition from the DP blast to the resting small DP stage (77, 84, 128). Moreover, the rise in metabolic activity in SP cells after the DP late stage could be due to functional TCR signaling that promotes the restoration of sensitivity to cytokines that fine-tune cellular metabolism (129, 130).
Although apoptosis of those DP cells that fail to be selected is crucial for thymocyte maturation program, it is also essential for pre-selection thymocytes to maintain a relatively low metabolic state to survive long enough waiting to be tested for their responses to self-peptide/MHC. Retinoid-related orphan nuclear receptor γt (RORγt) belongs to the nuclear hormone receptor superfamily of transcription factors and serves as a signaling node to connect lipid metabolism, inflammation, and immune cell responses (131). It has been demonstrated that RORγt expression reduces the abundance of cytokine receptors of the common chain (γc) and suppresses cellular metabolism and mitochondrial biogenesis in preselection DP thymocytes. DP thymocytes lacking RORγt exhibit features identical to persistent T cell expansion (132). O-linked β-N-acetylglucosamine protein O-GlcNAcylation shows a concomitant dynamic regulation with consecutive phases of T cell development and is controlled by Notch, c-Myc, and the T cell antigen receptors (133). O-GlcNAc transferase (OGT) acts as a signaling hub to integrate thymocyte responses to developmental stimuli through modifying changes in glucose and glutamine supply (134). An interesting finding has been shown that Ogt-/-CD4cre/+ mice had normal numbers of DP thymocytes but failed to differentiate into mature CD4+ or CD8+ SP thymocytes (134, 135). Pyruvate dehydrogenase (PDH) is required for thymocytes to regulate metabolic processes such as the tricarboxylic acid (TCA) cycle, redox homeostasis, glutathione levels, and pyruvate accumulation (136–138). Thymocytes can oxidize more glucose in the TCA cycle through PDH, and loss of PDH decreases the number of DP cells (139). Heme (iron-protoporphyrin IX) is an essential cofactor and signaling molecule involved in a vast array of biological processes, including cellular metabolism (140). Philip et al. reported a surprising finding that feline leukemia virus subgroup C receptor (FLVCR), the major facilitator superfamily (MFS) metabolite transporter and the heme exporter, was required for thymocyte development beyond the DP stage by supporting heme metabolism (141, 142). With FLVCR deletion during the DN stage, mice had a complete block in αβ T cell development at the DN-DP transition, whereas loss of FLVCR at the DP stage affects peripheral T cell proliferation and apoptosis (143).
THEMIS, a T cell specific protein that is highly expressed in DP cells, directly regulates the catalytic activity of SHP-1 by promoting ROS-mediated oxidation of the SHP-1 active site cysteine to facilitate thymic positive selection (144). N-linked glycosylation (NLG) also has an important impact on thymocyte selection. On the one hand, NLG negatively regulates the activity of high-affinity TCR, allowing thymocytes with these receptors to survive during negative selection. On the other hand, NLG increases expression of the CD4/CD8 co-receptors, allowing thymocytes with low-affinity TCR to survive during positive selection (145, 146).
Thymocyte egress is a crucial determinant factor of T cell homeostasis and adaptive immunity (147). Recently, protein geranylgeranyltransferase type I catalytic β-subunit (Pggt1b) has been shown to be involved in thymocyte egress by maintaining mevalonate metabolism–fueled posttranslational modification. Du et al. demonstrated that the expression of Pggt1b was upregulated in SP cells in comparison with DP cells, and deletion of Pggt1b impaired thymocyte egress, resulting in severe peripheral T cell lymphopenia but the accumulation of mature SP thymocytes in the thymus (148). Ultimately, CD4+ or CD8+ single-positive cells exit the thymus and circulate to peripheral organs, such as the spleen and lymph node.
The development of invariant natural killer T (iNKT) cells is more sensitive to changes in mitochondrial electron transport chain function than conventional αβT cells (149). There have been several informative reviews recently, so we won’t go over those details here (150–152).
Conclusion and perspective
In the past few years, extensive studies have largely focused on the metabolic regulation of T cell differentiation and responses. However, our knowledge of how cellular metabolism modulates thymocyte maturation in response to developmental signaling pathways and microenvironmental cues remains limited. Emerging studies using gene knockout mouse models have demonstrated that key metabolic regulators and enzymes are involved in different stages of thymocyte maturation by modulating the metabolic pathways and signaling networks to match the specific functional requirements of the stage (Figures 1 and 2). Recent progress in single-cell metabolomics, CRISPR/Cas9, and spatially resolved metabolomics will continue to add valuable findings to this field (10, 153). Future work on the molecular mechanisms of cell context-dependent regulation of these metabolic processes will not only enhance our understanding of the interplay between cellular metabolism and T cell developmental programs but also provide potential novel therapeutic strategies to modulate immune responses.
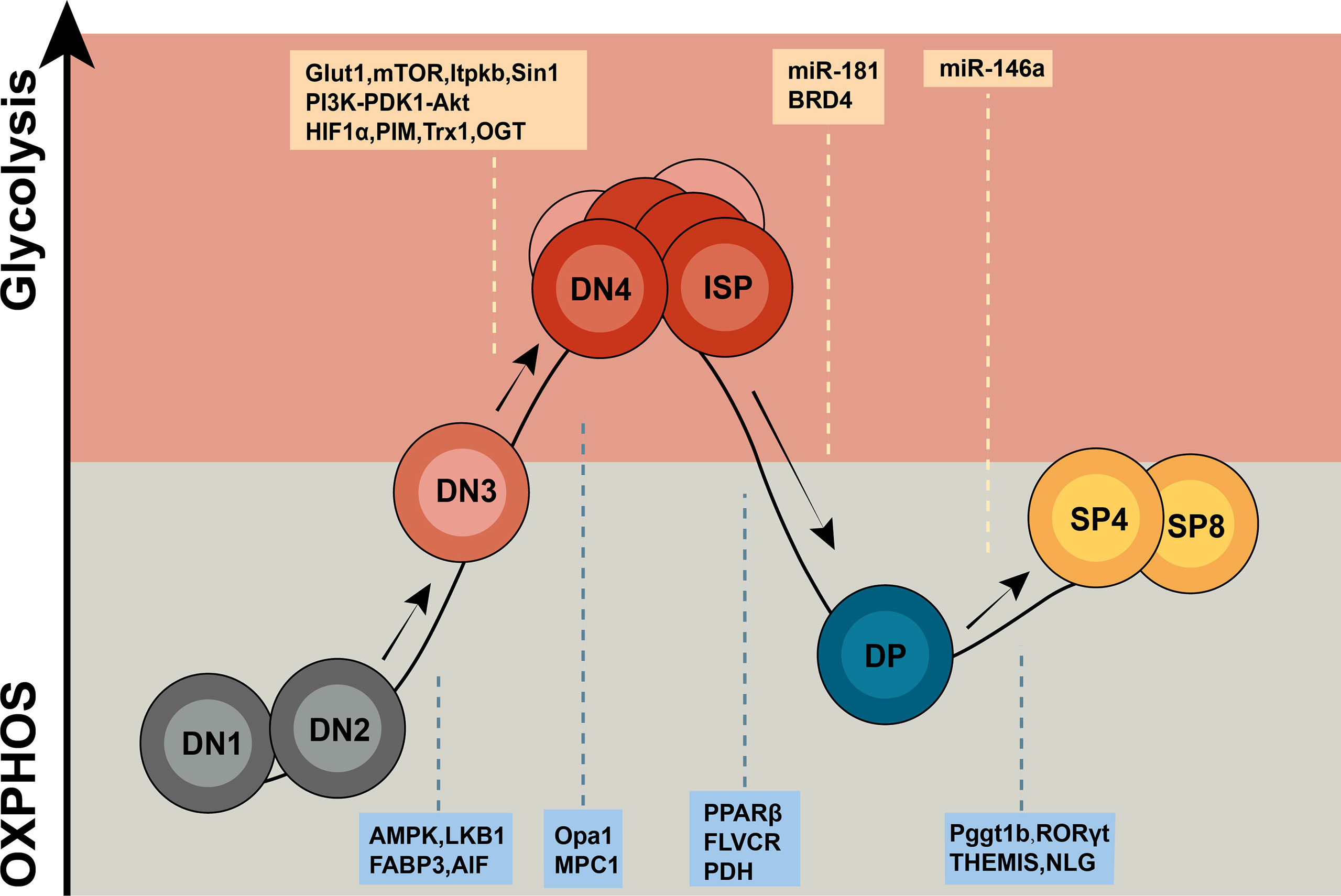
Figure 1 Overview of metabolic regulators in T cell development. Thymocytes display distinct metabolic profiles depending upon their states of development. DN1 and DN2 T cells are metabolically quiescent and adopt a basal level of nutritional intake, relying on OXPHOS as the primary approach of ATP production. Upon proliferation, T cells from the DN3b stage to early DP stage shift to a state of metabolic activation characterized by incremental nutrient uptake and elevated glycolysis. Then, T cells return to a resting state from the small DP stage to CD4+/CD8+ SP stage. The letters in the yellow box represent glycolysis regulators, and the letters in the blue box represent OXPHOS regulators.
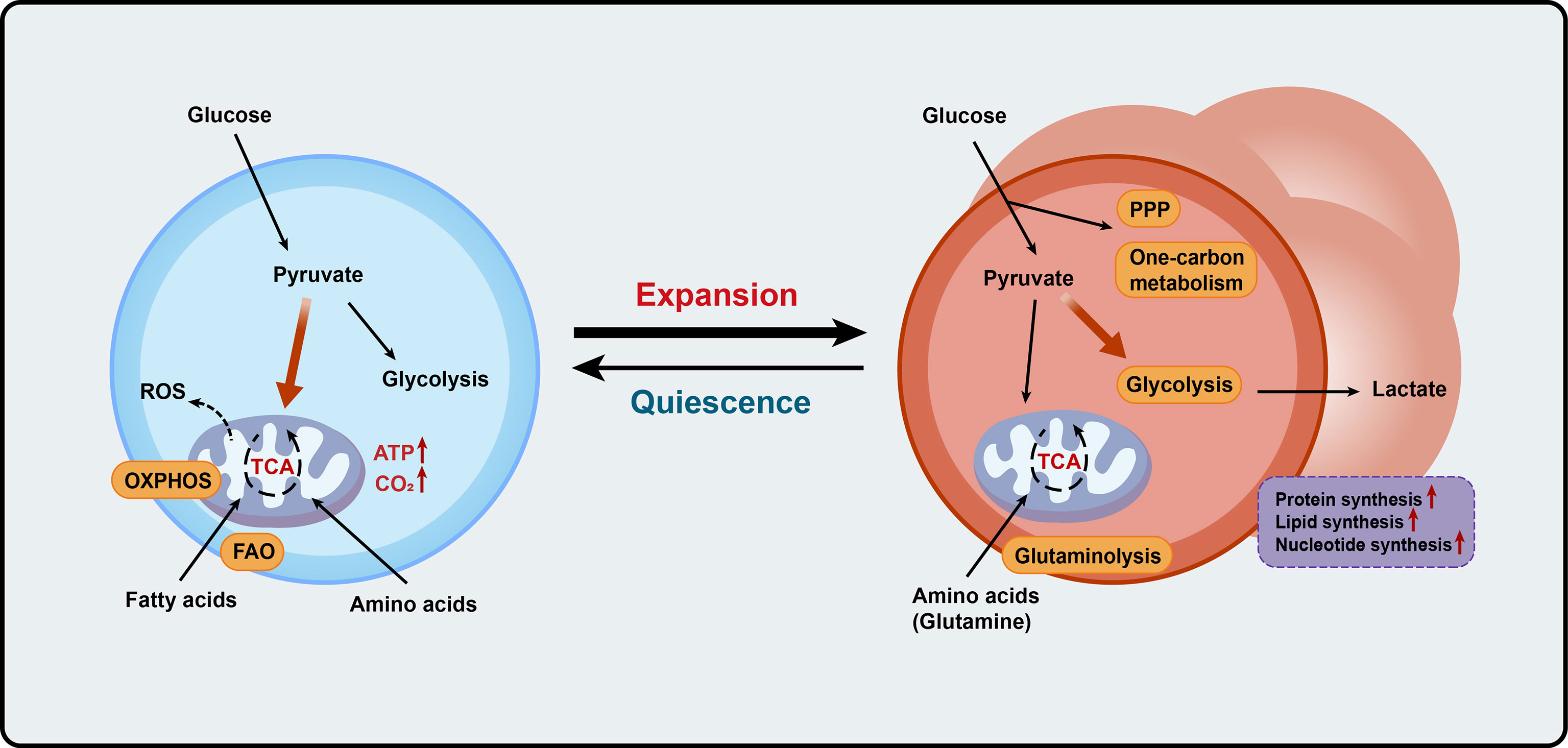
Figure 2 Metabolic programs match expansion demands of thymocytes. Blue cells on the left represent the quiescent thymocytes, and red cells on the right represent proliferative thymocytes. OXPHOS, oxidative phosphorylation; FAO, fatty acid oxidation; ROS, reactive oxygen species; ATP, adenosine triphosphate; PPP, pentose phosphate pathway; TCA, the tricarboxylic acid.
Author contributions
JH and BZ: conceptualization and guidance. MZ wrote and edited the manuscript. All authors contributed to the article and approved the submitted version.
Funding
This study was supported by the National Natural Science Foundation of China (grant numbers 82170795 and 82100949), and the Outstanding Young Investigator of Hunan Province (2022JJ10094).
Conflict of interest
The authors declare that the research was conducted in the absence of any commercial or financial relationships that could be construed as a potential conflict of interest.
Publisher’s note
All claims expressed in this article are solely those of the authors and do not necessarily represent those of their affiliated organizations, or those of the publisher, the editors and the reviewers. Any product that may be evaluated in this article, or claim that may be made by its manufacturer, is not guaranteed or endorsed by the publisher.
References
1. Kumar BV, Connors TJ, Farber DL. Human T cell development, localization, and function throughout life. Immunity (2018) 48(2):202–13. doi: 10.1016/j.immuni.2018.01.007
2. Hosokawa H, Rothenberg EV. How transcription factors drive choice of the T cell fate. Nat Rev Immunol (2021) 21(3):162–76. doi: 10.1038/s41577-020-00426-6
3. Balyan R, Gautam N, Gascoigne NRJ. The ups and downs of metabolism during the lifespan of a T cell. Int J Mol Sci (2020) 21(21):7972. doi: 10.3390/ijms21217972
4. Park JE, Botting RA, Dominguez Conde C, Popescu DM, Lavaert M, Kunz DJ, et al. A cell atlas of human thymic development defines T cell repertoire formation. Science (2020) 367(6480):eaay3224. doi: 10.1126/science.aay3224
5. Famili F, Wiekmeijer AS, Staal FJ. The development of T cells from stem cells in mice and humans. Future Sci OA (2017) 3(3):FSO186. doi: 10.4155/fsoa-2016-0095
6. Dutta A, Zhao B, Love PE. New insights into tcr beta-selection. Trends Immunol (2021) 42(8):735–50. doi: 10.1016/j.it.2021.06.005
7. Chen H, Yang T, Zhu L, Zhao Y. Cellular metabolism on T-cell development and function. Int Rev Immunol (2015) 34(1):19–33. doi: 10.3109/08830185.2014.902452
8. Ciofani M, Zuniga-Pflucker JC. Determining gammadelta versus alphass T cell development. Nat Rev Immunol (2010) 10(9):657–63. doi: 10.1038/nri2820
9. Xiong N, Raulet DH. Development and selection of gammadelta T cells. Immunol Rev (2007) 215:15–31. doi: 10.1111/j.1600-065X.2006.00478.x
10. Sun V, Sharpley M, Kaczor-Urbanowicz KE, Chang P, Montel-Hagen A, Lopez S, et al. The metabolic landscape of thymic T cell development in vivo and in vitro. Front Immunol (2021) 12:716661. doi: 10.3389/fimmu.2021.716661
11. Chapman NM, Boothby MR, Chi H. Metabolic coordination of T cell quiescence and activation. Nat Rev Immunol (2020) 20:55–70. doi: 10.1038/s41577-019-0203-y
12. Hu Z, Zou Q, Su B. Regulation of T cell immunity by cellular metabolism. Front Med (2018) 12(4):463–72. doi: 10.1007/s11684-018-0668-2
13. MacIver NJ, Michalek RD, Rathmell JC. Metabolic regulation of T lymphocytes. Annu Rev Immunol (2013) 31:259–83. doi: 10.1146/annurev-immunol-032712-095956
14. Almeida L, Lochner M, Berod L, Sparwasser T. Metabolic pathways in T cell activation and lineage differentiation. Semin Immunol (2016) 28(5):514–24. doi: 10.1016/j.smim.2016.10.009
15. Palmer CS, Ostrowski M, Balderson B, Christian N, Crowe SM. Glucose metabolism regulates T cell activation, differentiation, and functions. Front Immunol (2015) 6:1(1). doi: 10.3389/fimmu.2015.00001
16. Geltink RIK, Kyle RL, Pearce EL. Unraveling the complex interplay between T cell metabolism and function. Annu Rev Immunol (2018) 36:461–88. doi: 10.1146/annurev-immunol-042617-053019
17. van der Windt GJW, Pearce EL. Metabolic switching and fuel choice during T-cell differentiation and memory development. Immunol Rev (2012) 249(1):27–42. doi: 10.1111/j.1600-065X.2012.01150.x
18. Gerriets VA, Rathmell JC. Metabolic pathways in T cell fate and function. Trends Immunol (2012) 33(4):168–73. doi: 10.1016/j.it.2012.01.010
19. Barata J, Durum S, Seddon B. Flip the coin: Il-7 and il-7r in health and disease. Nat Immunol (2019) 20(12):1584–93. doi: 10.1038/s41590-019-0479-x
20. Boudil A, Matei IR, Shih HY, Bogdanoski G, Yuan JS, Chang SG, et al. Il-7 coordinates proliferation, differentiation and tcra recombination during thymocyte beta-selection. Nat Immunol (2015) 16(4):397–405. doi: 10.1038/ni.3122
21. Ciofani M, Zuniga-Pflucker JC. Notch promotes survival of pre-T cells at the beta-selection checkpoint by regulating cellular metabolism. Nat Immunol (2005) 6(9):881–8. doi: 10.1038/ni1234
22. Akashi K, Weissman IL. The c-kit+ maturation pathway in mouse thymic T cell development: Lineages and selection. Immunity (1996) 5(2):147–61. doi: 10.1016/s1074-7613(00)80491-4
23. Buono M, Facchini R, Matsuoka S, Thongjuea S, Waithe D, Luis TC, et al. A dynamic niche provides kit ligand in a stage-specific manner to the earliest thymocyte progenitors. Nat Cell Biol (2016) 18(2):157–67. doi: 10.1038/ncb3299
24. Hernández-López C, Varas A, Sacedón R, Jiménez E, Muñoz JJ, Zapata AG, et al. Stromal cell-derived factor 1/Cxcr4 signaling is critical for early human T-cell development. Blood (2002) 99(2):546–54. doi: 10.1182/blood.v99.2.546
25. Allam AH, Charnley M, Pham K, Russell SM. Developing T cells form an immunological synapse for passage through the β-selection checkpoint. J Cell Biol (2021) 220(3):e201908108. doi: 10.1083/jcb.201908108
26. Staal FJ, Luis TC, Tiemessen MM. Wnt signalling in the immune system: Wnt is spreading its wings. Nat Rev Immunol (2008) 8(8):581–93. doi: 10.1038/nri2360
27. Balciunaite G, Keller MP, Balciunaite E, Piali L, Zuklys S, Mathieu YD, et al. Wnt glycoproteins regulate the expression of Foxn1, the gene defective in nude mice. Nat Immunol (2002) 3(11):1102–8. doi: 10.1038/ni850
28. Trampont PC, Tosello-Trampont AC, Shen Y, Duley AK, Sutherland AE, Bender TP, et al. Cxcr4 acts as a costimulator during thymic beta-selection. Nat Immunol (2010) 11(2):162–70. doi: 10.1038/ni.1830
29. Janas ML, Varano G, Gudmundsson K, Noda M, Nagasawa T, Turner M. Thymic development beyond beta-selection requires phosphatidylinositol 3-kinase activation by Cxcr4. J Exp Med (2010) 207(1):247–61. doi: 10.1084/jem.20091430
30. Wong GW, Knowles GC, Mak TW, Ferrando AA, Zúñiga-Pflücker JC. Hes1 opposes a pten-dependent check on survival, differentiation, and proliferation of tcrβ-selected mouse thymocytes. Blood (2012) 120(7):1439–48. doi: 10.1182/blood-2011-12-395319
31. Hagenbeek TJ, Naspetti M, Malergue F, Garçon F, Nunès JA, Cleutjens KB, et al. The loss of pten allows tcr alphabeta lineage thymocytes to bypass il-7 and pre-Tcr-Mediated signaling. J Exp Med (2004) 200(7):883–94. doi: 10.1084/jem.20040495
32. Juntilla MM, Koretzky GA. Critical roles of the Pi3k/Akt signaling pathway in T cell development. Immunol Lett (2008) 116(2):104–10. doi: 10.1016/j.imlet.2007.12.008
33. Lee K, Nam KT, Cho SH, Gudapati P, Hwang Y, Park DS, et al. Vital roles of mtor complex 2 in notch-driven thymocyte differentiation and leukemia. J Exp Med (2012) 209(4):713–28. doi: 10.1084/jem.20111470
34. Radtke F, Wilson A, Stark G, Bauer M, van Meerwijk J, MacDonald HR, et al. Deficient T cell fate specification in mice with an induced inactivation of Notch1. Immunity (1999) 10(5):547–58. doi: 10.1016/s1074-7613(00)80054-0
35. Besseyrias V, Fiorini E, Strobl LJ, Zimber-Strobl U, Dumortier A, Koch U, et al. Hierarchy of notch-delta interactions promoting T cell lineage commitment and maturation. J Exp Med (2007) 204(2):331–43. doi: 10.1084/jem.20061442
36. Perumalsamy LR, Nagala M, Sarin A. Notch-activated signaling cascade interacts with mitochondrial remodeling proteins to regulate cell survival. Proc Natl Acad Sci USA (2010) 107(15):6882–7. doi: 10.1073/pnas.0910060107
37. Corrado M, Samardzic D, Giacomello M, Rana N, Pearce EL, Scorrano L. Deletion of the mitochondria-shaping protein Opa1 during early thymocyte maturation impacts mature memory T cell metabolism. Cell Death Differ (2021) 28(7):2194–206. doi: 10.1038/s41418-021-00747-6
38. Amsen D, Helbig C, Backer RA. Notch in T cell differentiation: All things considered. Trends Immunol (2015) 36(12):802–14. doi: 10.1016/j.it.2015.10.007
39. Ma EH, Poffenberger MC, Wong AH, Jones RG. The role of ampk in T cell metabolism and function. Curr Opin Immunol (2017) 46:45–52. doi: 10.1016/j.coi.2017.04.004
40. Herzig S, Shaw RJ. Ampk: Guardian of metabolism and mitochondrial homeostasis. Nat Rev Mol Cell Biol (2018) 19(2):121–35. doi: 10.1038/nrm.2017.95
41. Tamas P, Hawley SA, Clarke RG, Mustard KJ, Green K, Hardie DG, et al. Regulation of the energy sensor amp-activated protein kinase by antigen receptor and Ca2+ in T lymphocytes. J Exp Med (2006) 203(7):1665–70. doi: 10.1084/jem.20052469
42. MacIver NJ, Blagih J, Saucillo DC, Tonelli L, Griss T, Rathmell JC, et al. The liver kinase B1 is a central regulator of T cell development, activation, and metabolism. J Immunol (2011) 187(8):4187–98. doi: 10.4049/jimmunol.1100367
43. Blagih J, Krawczyk CM, Jones RG. Lkb1 and ampk: Central regulators of lymphocyte metabolism and function. Immunol Rev (2012) 249(1):59–71. doi: 10.1111/j.1600-065X.2012.01157.x
44. Cao Y, Li H, Liu H, Zheng C, Ji H, Liu X. The Serine/Threonine kinase Lkb1 controls thymocyte survival through regulation of ampk activation and bcl-xl expression. Cell Res (2010) 20(1):99–108. doi: 10.1038/cr.2009.141
45. Tamás P, Macintyre A, Finlay D, Clarke R, Feijoo-Carnero C, Ashworth A, et al. Lkb1 is essential for the proliferation of T-cell progenitors and mature peripheral T cells. Eur J Immunol (2010) 40(1):242–53. doi: 10.1002/eji.200939677
46. Kobayashi S, Phung HT, Kagawa Y, Miyazaki H, Takahashi Y, Asao A, et al. Fatty acid-binding protein 3 controls contact hypersensitivity through regulating skin dermal Vγ4+Γ/Δ T cell in a murine model. Allergy (2020) 76(6):1776–88. doi: 10.1111/all.14630
47. Cabon L, Bertaux A, Brunelle-Navas MN, Nemazanyy I, Scourzic L, Delavallee L, et al. Aif loss deregulates hematopoiesis and reveals different adaptive metabolic responses in bone marrow cells and thymocytes. Cell Death Differ (2018) 25(5):983–1001. doi: 10.1038/s41418-017-0035-x
48. Aifantis I, Mandal M, Sawai K, Ferrando A, Vilimas T. Regulation of T-cell progenitor survival and cell-cycle entry by the pre-T-Cell receptor. Immunol Rev (2006) 209:159–69. doi: 10.1111/j.0105-2896.2006.00343.x
49. Rangel Rivera GO, Knochelmann HM, Dwyer CJ, Smith AS, Wyatt MM, Rivera-Reyes AM, et al. Fundamentals of T cell metabolism and strategies to enhance cancer immunotherapy. Front Immunol (2021) 12:645242. doi: 10.3389/fimmu.2021.645242
50. Diskin C, Ryan TAJ, O'Neill LAJ. Modification of proteins by metabolites in immunity. Immunity (2021) 54(1):19–31. doi: 10.1016/j.immuni.2020.09.014
51. Swainson L, Kinet S, Manel N, Battini JL, Sitbon M, Taylor N. Glucose transporter 1 expression identifies a population of cycling Cd4+ Cd8+ human thymocytes with high Cxcr4-induced chemotaxis. Proc Natl Acad Sci USA (2005) 102(36):12867–72. doi: 10.1073/pnas.0503603102
52. Barata JT, Silva A, Brandao JG, Nadler LM, Cardoso AA, Boussiotis VA. Activation of Pi3k is indispensable for interleukin 7-mediated viability, proliferation, glucose use, and growth of T cell acute lymphoblastic leukemia cells. J Exp Med (2004) 200(5):659–69. doi: 10.1084/jem.20040789
53. Zhang XY, Yang BY, Wang JY, Mo X, Zhang J, Hua ZC. Fadd is essential for glucose uptake and survival of thymocytes. Biochem Biophys Res Commun (2014) 451(2):202–7. doi: 10.1016/j.bbrc.2014.07.092
54. Macintyre AN, Gerriets VA, Nichols AG, Michalek RD, Rudolph MC, Deoliveira D, et al. The glucose transporter Glut1 is selectively essential for Cd4 T cell activation and effector function. Cell Metab (2014) 20(1):61–72. doi: 10.1016/j.cmet.2014.05.004
55. Kelly AP, Finlay DK, Hinton HJ, Clarke RG, Fiorini E, Radtke F, et al. Notch-induced T cell development requires phosphoinositide-dependent kinase 1. EMBO J (2007) 26(14):3441–50. doi: 10.1038/sj.emboj.7601761
56. Juntilla MM, Wofford JA, Birnbaum MJ, Rathmell JC, Koretzky GA. Akt1 and Akt2 are required for alphabeta thymocyte survival and differentiation. Proc Natl Acad Sci U.S.A. (2007) 104(29):12105–10. doi: 10.1073/pnas.0705285104
57. Ji H, Rintelen F, Waltzinger C, Bertschy Meier D, Bilancio A, Pearce W, et al. Inactivation of Pi3kgamma and Pi3kdelta distorts T-cell development and causes multiple organ inflammation. Blood (2007) 110(8):2940–7. doi: 10.1182/blood-2007-04-086751
58. Swat W, Montgrain V, Doggett TA, Douangpanya J, Puri K, Vermi W, et al. Essential role of Pi3kdelta and Pi3kgamma in thymocyte survival. Blood (2006) 107(6):2415–22. doi: 10.1182/blood-2005-08-3300
59. Hinton HJ, Alessi DR, Cantrell DA. The serine kinase phosphoinositide-dependent kinase 1 (Pdk1) regulates T cell development. Nat Immunol (2004) 5(5):539–45. doi: 10.1038/ni1062
60. Kelly AP, Hinton HJ, Clarke RG, Cantrell DA. Phosphoinositide-dependent kinase l (Pdk1) haplo-insufficiency inhibits production of Alpha/Beta (Alpha/Beta) but not gamma delta (Gamma/Delta) T lymphocytes. FEBS Lett (2006) 580(8):2135–40. doi: 10.1016/j.febslet.2006.03.022
61. Abdullah L, Hills LB, Winter EB, Huang YH. Diverse roles of akt in T cells. Immunometabolism (2021) 3(1):e210007. doi: 10.20900/immunometab20210007
62. Hoxhaj G, Manning BD. The Pi3k-akt network at the interface of oncogenic signalling and cancer metabolism. Nat Rev Cancer (2020) 20(2):74–88. doi: 10.1038/s41568-019-0216-7
63. Mao C, Tili EG, Dose M, Haks MC, Bear SE, Maroulakou I, et al. Unequal contribution of akt isoforms in the double-negative to double-positive thymocyte transition. J Immunol (2007) 178(9):5443–53. doi: 10.4049/jimmunol.178.9.5443
64. Leduc I, Karsunky H, Mathieu N, Schmidt T, Verthuy C, Ferrier P, et al. The pim-1 kinase stimulates maturation of tcrbeta-deficient T cell progenitors: Implications for the mechanism of pim-1 action. Int Immunol (2000) 12(10):1389–96. doi: 10.1093/intimm/12.10.1389
65. Pearson R, Weston K. C-myb regulates the proliferation of immature thymocytes following beta-selection. EMBO J (2000) 19(22):6112–20. doi: 10.1093/emboj/19.22.6112
66. Malone T, Schafer L, Simon N, Heavey S, Cuffe S, Finn S, et al. Current perspectives on targeting pim kinases to overcome mechanisms of drug resistance and immune evasion in cancer. Pharmacol Ther (2020) 207:107454. doi: 10.1016/j.pharmthera.2019.107454
67. Westernberg L, Conche C, Huang YH, Rigaud S, Deng Y, Siegemund S, et al. Non-canonical antagonism of Pi3k by the kinase itpkb delays thymocyte β-selection and renders it notch-dependent. Elife (2016) 5:e10786. doi: 10.7554/eLife.10786
68. Liu GY, Sabatini DM. Mtor at the nexus of nutrition, growth, ageing and disease. Nat Rev Mol Cell Biol (2020) 21(4):183–203. doi: 10.1038/s41580-019-0199-y
69. Mossmann D, Park S, Hall MN. Mtor signalling and cellular metabolism are mutual determinants in cancer. Nat Rev Cancer (2018) 18(12):744–57. doi: 10.1038/s41568-018-0074-8
70. Yang K, Blanco DB, Chen X, Dash P, Neale G, Rosencrance C, et al. Metabolic signaling directs the reciprocal lineage decisions of αβ and Γδ T cells. Sci Immunol (2018) 3(25):eaas9818. doi: 10.1126/sciimmunol.aas9818
71. Werlen G, Jain R, Jacinto E. Mtor signaling and metabolism in early T cell development. Genes (Basel) (2021) 12(5):728. doi: 10.3390/genes12050728
72. Huang H, Long L, Zhou P, Chapman NM, Chi H. Mtor signaling at the crossroads of environmental signals and T-cell fate decisions. Immunol Rev (2020) 295(1):15–38. doi: 10.1111/imr.12845
73. Peterson TR, Sengupta SS, Harris TE, Carmack AE, Kang SA, Balderas E, et al. Mtor complex 1 regulates lipin 1 localization to control the srebp pathway. Cell (2011) 146(3):408–20. doi: 10.1016/j.cell.2011.06.034
74. Hoshii T, Kasada A, Hatakeyama T, Ohtani M, Tadokoro Y, Naka K, et al. Loss of mtor complex 1 induces developmental blockage in early T-lymphopoiesis and eradicates T-cell acute lymphoblastic leukemia cells. Proc Natl Acad Sci USA (2014) 111(10):3805–10. doi: 10.1073/pnas.1320265111
75. Mergenthaler P, Kahl A, Kamitz A, van Laak V, Stohlmann K, Thomsen S, et al. Mitochondrial hexokinase ii (Hkii) and phosphoprotein enriched in astrocytes (Pea15) form a molecular switch governing cellular fate depending on the metabolic state. Proc Natl Acad Sci USA (2012) 109(5):1518–23. doi: 10.1073/pnas.1108225109
76. Semba H, Takeda N, Isagawa T, Sugiura Y, Honda K, Wake M, et al. Hif-1α-Pdk1 axis-induced active glycolysis plays an essential role in macrophage migratory capacity. Nat Commun (2016) 7:11635. doi: 10.1038/ncomms11635
77. Biju MP, Neumann AK, Bensinger SJ, Johnson RS, Turka LA, Haase VH. Vhlh gene deletion induces hif-1-Mediated cell death in thymocytes. Mol Cell Biol (2004) 24(20):9038–47. doi: 10.1128/mcb.24.20.9038-9047.2004
78. Cheng SC, Quintin J, Cramer RA, Shepardson KM, Saeed S, Kumar V, et al. Mtor- and hif-1α-Mediated aerobic glycolysis as metabolic basis for trained immunity. Science (2014) 345(6204):1250684. doi: 10.1126/science.1250684
79. Yuan HX, Guan KL. The Sin1-ph domain connects Mtorc2 to Pi3k. Cancer Discovery (2015) 5(11):1127–9. doi: 10.1158/2159-8290.Cd-15-1125
80. Chou P-C, Oh WJ, Wu C-C, Moloughney J, Rüegg MA, Hall MN, et al. Mammalian target of rapamycin complex 2 modulates αβtcr processing and surface expression during thymocyte development. J Immunol (2014) 193(3):1162–70. doi: 10.4049/jimmunol.1303162
81. Wang HX, Cheng JS, Chu S, Qiu YR, Zhong XP. Mtorc2 in thymic epithelial cells controls thymopoiesis and T cell development. J Immunol (2016) 197(1):141–50. doi: 10.4049/jimmunol.1502698
82. Chi H. Sin1-Mtorc2 signaling drives glycolysis of developing thymocytes. J Mol Cell Biol (2019) 11(2):91–2. doi: 10.1093/jmcb/mjy078
83. Ouyang X, Han Y, Qu G, Li M, Wu N, Liu H, et al. Metabolic regulation of T cell development by Sin1-Mtorc2 is mediated by pyruvate kinase M2. J Mol Cell Biol (2019) 11(2):93–106. doi: 10.1093/jmcb/mjy065
84. Dose M, Khan I, Guo Z, Kovalovsky D, Krueger A, von Boehmer H, et al. C-myc mediates pre-Tcr-Induced proliferation but not developmental progression. Blood (2006) 108(8):2669–77. doi: 10.1182/blood-2006-02-005900
85. Douglas NC, Jacobs H, Bothwell AL, Hayday AC. Defining the specific physiological requirements for c-myc in T cell development. Nat Immunol (2001) 2(4):307–15. doi: 10.1038/86308
86. Li Q, Pan S, Xie T, Liu H. Myc in T-cell acute lymphoblastic leukemia: Functional implications and targeted strategies. Blood Sci (Baltimore Md) (2021) 3(3):65–70. doi: 10.1097/bs9.0000000000000073
87. Wang R, Dillon CP, Shi LZ, Milasta S, Carter R, Finkelstein D, et al. The transcription factor myc controls metabolic reprogramming upon T lymphocyte activation. Immunity (2011) 35(6):871–82. doi: 10.1016/j.immuni.2011.09.021
88. Muri J, Heer S, Matsushita M, Pohlmeier L, Tortola L, Fuhrer T, et al. The thioredoxin-1 system is essential for fueling DNA synthesis during T-cell metabolic reprogramming and proliferation. Nat Commun (2018) 9(1):1851. doi: 10.1038/s41467-018-04274-w
89. Liang Y, Tian J, Wu T. Brd4 in physiology and pathology: ''Bet'' on its partners. BioEssays News Rev Mol Cell Dev Biol (2021) 43(12):e2100180. doi: 10.1002/bies.202100180
90. Donati B, Lorenzini E, Ciarrocchi A. Brd4 and cancer: Going beyond transcriptional regulation. Mol Cancer (2018) 17(1):164. doi: 10.1186/s12943-018-0915-9
91. Wang N, Wu R, Tang D, Kang R. The bet family in immunity and disease. Signal Transduction Targeted Ther (2021) 6(1):23. doi: 10.1038/s41392-020-00384-4
92. Devaiah BN, Case-Borden C, Gegonne A, Hsu CH, Chen Q, Meerzaman D, et al. Erratum: Brd4 is a histone acetyltransferase that evicts nucleosomes from chromatin. Nat Struct Mol Biol (2017) 24(2):194. doi: 10.1038/nsmb0217-194c
93. Dey A, Nishiyama A, Karpova T, McNally J, Ozato K. Brd4 marks select genes on mitotic chromatin and directs postmitotic transcription. Mol Biol Cell (2009) 20(23):4899–909. doi: 10.1091/mbc.e09-05-0380
94. Gegonne A, Chen QR, Dey A, Etzensperger R, Tai X, Singer A, et al. Immature Cd8 single-positive thymocytes are a molecularly distinct subpopulation, selectively dependent on Brd4 for their differentiation. Cell Rep (2018) 24(1):117–29. doi: 10.1016/j.celrep.2018.06.007
95. Mothe-Satney I, Murdaca J, Sibille B, Rousseau AS, Squillace R, Le Menn G, et al. A role for peroxisome proliferator-activated receptor beta in T cell development. Sci Rep (2016) 6:34317. doi: 10.1038/srep34317
96. Zhao FL, Ahn JJ, Chen ELY, Yi TJ, Stickle NH, Spaner D, et al. Peroxisome proliferator-activated receptor-delta supports the metabolic requirements of cell growth in tcrbeta-selected thymocytes and peripheral Cd4(+) T cells. J Immunol (2018) 201(9):2664–82. doi: 10.4049/jimmunol.1800374
97. Ramstead AG, Wallace JA, Lee SH, Bauer KM, Tang WW, Ekiz HA, et al. Mitochondrial pyruvate carrier 1 promotes peripheral T cell homeostasis through metabolic regulation of thymic development. Cell Rep (2020) 30(9):2889–99.e6. doi: 10.1016/j.celrep.2020.02.042
98. Mildner A, Chapnik E, Varol D, Aychek T, Lampl N, Rivkin N, et al. Microrna-142 controls thymocyte proliferation. Eur J Immunol (2017) 47(7):1142–52. doi: 10.1002/eji.201746987
99. Wang J, Li K, Zhang X, Li G, Liu T, Wu X, et al. Microrna-155 controls inkt cell development and lineage differentiation by coordinating multiple regulating pathways. Front Cell Dev Biol (2020) 8:619220. doi: 10.3389/fcell.2020.619220
100. Nikhat S, Yadavalli AD, Prusty A, Narayan PK, Palakodeti D, Murre C, et al. A regulatory network of micrornas confers lineage commitment during early developmental trajectories of b and T lymphocytes. Proc Natl Acad Sci USA (2021) 118(46):e2104297118. doi: 10.1073/pnas.2104297118
101. Hu L, Zhou Y, Yang J, Zhao X, Mao L, Zheng W, et al. Microrna-7 overexpression positively regulates the Cd8+ sp cell development Via targeting Pik3r1. Exp Cell Res (2021) 407(2):112824. doi: 10.1016/j.yexcr.2021.112824
102. Emamgolizadeh Gurt Tapeh B, Mosayyebi B, Samei M, Beyrampour Basmenj H, Mohammadi A, Alivand MR, et al. Micrornas involved in T-cell development, selection, activation, and hemostasis. J Cell Physiol (2020) 235(11):8461–71. doi: 10.1002/jcp.29689
103. Cho S, Dong J, Lu LF. Cell-intrinsic and -extrinsic roles of mirnas in regulating T cell immunity. Immunol Rev (2021) 304(1):126–40. doi: 10.1111/imr.13029
104. Henao-Mejia J, Williams A, Goff LA, Staron M, Licona-Limon P, Kaech SM, et al. The microrna mir-181 is a critical cellular metabolic rheostat essential for nkt cell ontogenesis and lymphocyte development and homeostasis. Immunity (2013) 38(5):984–97. doi: 10.1016/j.immuni.2013.02.021
105. Grewers Z, Krueger A. Microrna mir-181-a rheostat for tcr signaling in thymic selection and peripheral T-cell function. Int J Mol Sci (2020) 21(17):6200. doi: 10.3390/ijms21176200
106. Li QJ, Chau J, Ebert PJ, Sylvester G, Min H, Liu G, et al. Mir-181a is an intrinsic modulator of T cell sensitivity and selection. Cell (2007) 129(1):147–61. doi: 10.1016/j.cell.2007.03.008
107. Winter SJ, Kunze-Schumacher H, Imelmann E, Grewers Z, Osthues T, Krueger A. Microrna mir-181a/B-1 controls mait cell development. Immunol Cell Biol (2019) 97(2):190–202. doi: 10.1111/imcb.12211
108. Łyszkiewicz M, Winter SJ, Witzlau K, Föhse L, Brownlie R, Puchałka J, et al. Mir-181a/B-1 controls thymic selection of treg cells and tunes their suppressive capacity. PLoS Biol (2019) 17(3):e2006716. doi: 10.1371/journal.pbio.2006716
109. Fragoso R, Mao T, Wang S, Schaffert S, Gong X, Yue S, et al. Modulating the strength and threshold of notch oncogenic signals by mir-181a-1/B-1. PLoS Genet (2012) 8(8):e1002855. doi: 10.1371/journal.pgen.1002855
110. Li Z, Zhang S, Wan Y, Cai M, Wang W, Zhu Y, et al. Microrna-146a overexpression impairs the positive selection during T cell development. Front Immunol (2017) 8:2006. doi: 10.3389/fimmu.2017.02006
111. Meng Z, Cao G, Yang Q, Yang H, Hao J, Yin Z. Metabolic control of Γδ T cell function. Infect Microbe Dis(2021) 3(3):142–8. doi: 10.1097/im9.0000000000000054
112. Ciofani M, Zuniga-Pflucker JC. The thymus as an inductive site for T lymphopoiesis. Annu Rev Cell Dev Biol (2007) 23:463–93. doi: 10.1146/annurev.cellbio.23.090506.123547
113. Hayes SM, Love PE. A retrospective on the requirements for gammadelta T-cell development. Immunol Rev (2007) 215:8–14. doi: 10.1111/j.1600-065X.2006.00476.x
114. Prinz I, Sansoni A, Kissenpfennig A, Ardouin L, Malissen M, Malissen B. Visualization of the earliest steps of Γδ T cell development in the adult thymus. Nat Immunol (2006) 7(9):995–1003. doi: 10.1038/ni1371
115. Jensen KD, Su X, Shin S, Li L, Youssef S, Yamasaki S, et al. Thymic selection determines gammadelta T cell effector fate: Antigen-naive cells make interleukin-17 and antigen-experienced cells make interferon gamma. Immunity (2008) 29(1):90–100. doi: 10.1016/j.immuni.2008.04.022
116. Fiala GJ, Gomes AQ, Silva-Santos B. From thymus to periphery: Molecular basis of effector gammadelta-T cell differentiation. Immunol Rev (2020) 298(1):47–60. doi: 10.1111/imr.12918
117. Parker ME, Ciofani M. Regulation of gammadelta T cell effector diversification in the thymus. Front Immunol (2020) 11:42. doi: 10.3389/fimmu.2020.00042
118. Lopes N, McIntyre C, Martin S, Raverdeau M, Sumaria N, Kohlgruber AC, et al. Distinct metabolic programs established in the thymus control effector functions of gammadelta T cell subsets in tumor microenvironments. Nat Immunol (2021) 22(2):179–92. doi: 10.1038/s41590-020-00848-3
119. Li G, Liu L, Yin Z, Ye Z, Shen N. Glutamine metabolism is essential for the production of il-17a in gammadelta T cells and skin inflammation. Tissue Cell (2021) 71:101569. doi: 10.1016/j.tice.2021.101569
120. Park JH, Kim HJ, Kim CW, Kim HC, Jung Y, Lee HS, et al. Tumor hypoxia represses gammadelta T cell-mediated antitumor immunity against brain tumors. Nat Immunol (2021) 22(3):336–46. doi: 10.1038/s41590-020-00860-7
121. Lopes N, Silva-Santos B. Functional and metabolic dichotomy of murine gammadelta T cell subsets in cancer immunity. Eur J Immunol (2021) 51(1):17–26. doi: 10.1002/eji.201948402
122. Yang Q, Liu X, Liu Q, Guan Z, Luo J, Cao G, et al. Roles of Mtorc1 and Mtorc2 in controlling gammadelta T1 and gammadelta T17 differentiation and function. Cell Death Differ (2020) 27(7):2248–62. doi: 10.1038/s41418-020-0500-9
123. Jee MH, Mraz V, Geisler C, Bonefeld CM. Gammadelta T cells and inflammatory skin diseases. Immunol Rev (2020) 298(1):61–73. doi: 10.1111/imr.12913
124. Douguet L, Cherfils-Vicini J, Bod L, Lengagne R, Gilson E, Prevost-Blondel A. Nitric oxide synthase 2 improves proliferation and glycolysis of peripheral gammadelta T cells. PLoS One (2016) 11(11):e0165639. doi: 10.1371/journal.pone.0165639
125. Xia X, Cao G, Sun G, Zhu L, Tian Y, Song Y, et al. Gls1-mediated glutaminolysis unbridled by Malt1 protease promotes psoriasis pathogenesis. J Clin Invest (2020) 130(10):5180–96. doi: 10.1172/JCI129269
126. Chen X, Cai Y, Hu X, Ding C, He L, Zhang X, et al. Differential metabolic requirement governed by transcription factor c-maf dictates innate Γδt17 effector functionality in mice and humans. Sci Adv (2022) 8(21):eabm9120. doi: 10.1126/sciadv.abm9120
127. Ron-Harel N, Sharpe AH, Haigis MC. Mitochondrial metabolism in T cell activation and senescence: A mini-review. Gerontology (2015) 61(2):131–8. doi: 10.1159/000362502
128. Mingueneau M, Kreslavsky T, Gray D, Heng T, Cruse R, Ericson J, et al. The transcriptional landscape of αβ T cell differentiation. Nat Immunol (2013) 14(6):619–32. doi: 10.1038/ni.2590
129. Gaud G, Lesourne R, Love PE. Regulatory mechanisms in T cell receptor signalling. Nat Rev Immunol (2018) 18(8):485–97. doi: 10.1038/s41577-018-0020-8
130. Gascoigne NR, Rybakin V, Acuto O, Brzostek J. Tcr signal strength and T cell development. Annu Rev Cell Dev Biol (2016) 32:327–48. doi: 10.1146/annurev-cellbio-111315-125324
131. Eberl G. Rorγt, a multitask nuclear receptor at mucosal surfaces. Mucosal Immunol (2017) 10(1):27–34. doi: 10.1038/mi.2016.86
132. Ligons DL, Hwang S, Waickman AT, Park JY, Luckey MA, Park JH. Rorγt limits the amount of the cytokine receptor Γc through the prosurvival factor bcl-X(L) in developing thymocytes. Sci Signal (2018) 11(545):eaam8939. doi: 10.1126/scisignal.aam8939
133. Abramowitz LK, Hanover JA. T Cell development and the physiological role of O-glcnac. FEBS Lett (2018) 592(23):3943–9. doi: 10.1002/1873-3468.13159
134. Swamy M, Pathak S, Grzes KM, Damerow S, Sinclair LV, van Aalten DM, et al. Glucose and glutamine fuel protein O-glcnacylation to control T cell self-renewal and malignancy. Nat Immunol (2016) 17(6):712–20. doi: 10.1038/ni.3439
135. Abramowitz LK, Harly C, Das A, Bhandoola A, Hanover JA. Blocked O-glcnac cycling disrupts mouse hematopoeitic stem cell maintenance and early T cell development. Sci Rep (2019) 9(1):12569. doi: 10.1038/s41598-019-48991-8
136. Golias T, Kery M, Radenkovic S, Papandreou I. Microenvironmental control of glucose metabolism in tumors by regulation of pyruvate dehydrogenase. Int J Cancer (2019) 144(4):674–86. doi: 10.1002/ijc.31812
137. Park S, Jeon JH, Min BK, Ha CM, Thoudam T, Park BY, et al. Role of the pyruvate dehydrogenase complex in metabolic remodeling: Differential pyruvate dehydrogenase complex functions in metabolism. Diabetes Metab J (2018) 42(4):270–81. doi: 10.4093/dmj.2018.0101
138. Anwar S, Shamsi A, Mohammad T, Islam A, Hassan MI. Targeting pyruvate dehydrogenase kinase signaling in the development of effective cancer therapy. Biochim Biophys Acta Rev Cancer (2021) 1876(1):188568. doi: 10.1016/j.bbcan.2021.188568
139. Jun S, Mahesula S, Mathews TP, Martin-Sandoval MS, Zhao Z, Piskounova E, et al. The requirement for pyruvate dehydrogenase in leukemogenesis depends on cell lineage. Cell Metab (2021) 33(9):1777–92.e8. doi: 10.1016/j.cmet.2021.07.016
140. Keel SB, Doty RT, Yang Z, Quigley JG, Chen J, Knoblaugh S, et al. A heme export protein is required for red blood cell differentiation and iron homeostasis. Science (2008) 319(5864):825–8. doi: 10.1126/science.1151133
141. Feng D, Lazar MA. Clocks, metabolism, and the epigenome. Mol Cell (2012) 47(2):158–67. doi: 10.1016/j.molcel.2012.06.026
142. Reddy VS, Shlykov MA, Castillo R, Sun EI, Saier MH Jr. The major facilitator superfamily (Mfs) revisited. FEBS J (2012) 279(11):2022–35. doi: 10.1111/j.1742-4658.2012.08588.x
143. Philip M, Funkhouser SA, Chiu EY, Phelps SR, Delrow JJ, Cox J, et al. Heme exporter flvcr is required for T cell development and peripheral survival. J Immunol (2015) 194(4):1677–85. doi: 10.4049/jimmunol.1402172
144. Choi S, Warzecha C, Zvezdova E, Lee J, Argenty J, Lesourne R, et al. Themis enhances tcr signaling and enables positive selection by selective inhibition of the phosphatase shp-1. Nat Immunol (2017) 18(4):433–41. doi: 10.1038/ni.3692
145. Zhou RW, Mkhikian H, Grigorian A, Hong A, Chen D, Arakelyan A, et al. N-glycosylation bidirectionally extends the boundaries of thymocyte positive selection by decoupling lck from Ca²+ signaling. Nat Immunol (2014) 15(11):1038–45. doi: 10.1038/ni.3007
146. Shyer JA, Flavell RA, Bailis W. Metabolic signaling in T cells. Cell Res (2020) 30(8):649–59. doi: 10.1038/s41422-020-0379-5
147. Buck MD, Sowell RT, Kaech SM, Pearce EL. Metabolic instruction of immunity. Cell (2017) 169(4):570–86. doi: 10.1016/j.cell.2017.04.004
148. Du X, Zeng H, Liu S, Guy C, Dhungana Y, Neale G, et al. Mevalonate metabolism-dependent protein geranylgeranylation regulates thymocyte egress. J Exp Med (2020) 217(2):e20190969. doi: 10.1084/jem.20190969
149. Weng X, Kumar A, Cao L, He Y, Morgun E, Visvabharathy L, et al. Mitochondrial metabolism is essential for invariant natural killer T cell development and function. Proc Natl Acad Sci U.S.A. (2021) 118(13):e2021385118. doi: 10.1073/pnas.2021385118
150. Yarosz EL, Chang C-H, Kumar A. Metabolism in invariant natural killer T cells: An overview. Immunometabolism (2021) 3(2):. doi: 10.20900/immunometab20210010
151. Yang G, Driver JP, Van Kaer L. The role of autophagy in inkt cell development. Front Immunol (2018) 9:2653. doi: 10.3389/fimmu.2018.02653
152. Shissler SC, Webb TJ. The ins and outs of type I inkt cell development. Mol Immunol (2019) 105:116–30. doi: 10.1016/j.molimm.2018.09.023
Keywords: T cell development, thymocytes, T cell metabolism, thymus, thymocyte metabolism
Citation: Zhang M, Lin X, Yang Z, Li X, Zhou Z, Love PE, Huang J and Zhao B (2022) Metabolic regulation of T cell development. Front. Immunol. 13:946119. doi: 10.3389/fimmu.2022.946119
Received: 17 May 2022; Accepted: 24 June 2022;
Published: 25 July 2022.
Edited by:
Michele Kay Anderson, University of Toronto, CanadaReviewed by:
Bruno Silva-Santos, Universidade de Lisboa, PortugalNicole M. Chapman, St. Jude Children’s Research Hospital, United States
Copyright © 2022 Zhang, Lin, Yang, Li, Zhou, Love, Huang and Zhao. This is an open-access article distributed under the terms of the Creative Commons Attribution License (CC BY). The use, distribution or reproduction in other forums is permitted, provided the original author(s) and the copyright owner(s) are credited and that the original publication in this journal is cited, in accordance with accepted academic practice. No use, distribution or reproduction is permitted which does not comply with these terms.
*Correspondence: Bin Zhao, binzhao@csu.edu.cn; bin.zhao@live.com; Jiaqi Huang, jiaqi.huang@csu.edu.cn;; jiaqi.huang@live.com
†These authors jointly supervised this work