- Laboratory of Microenvironmental and Metabolic Health Sciences, Center for Disease Biology and Integrative Medicine, Graduate School of Medicine, The University of Tokyo, Bunkyo-ku, Japan
Lipids play fundamental roles in life as an essential component of cell membranes, as a major source of energy, as a body surface barrier, and as signaling molecules that transmit intracellular and intercellular signals. Lipid mediators, a group of bioactive lipids that mediates intercellular signals, are produced via specific biosynthetic enzymes and transmit signals via specific receptors. Mast cells, a tissue-resident immune cell population, produce several lipid mediators that contribute to exacerbation or amelioration of allergic responses and also non-allergic inflammation, host defense, cancer and fibrosis by controlling the functions of microenvironmental cells as well as mast cell themselves in paracrine and autocrine fashions. Additionally, several bioactive lipids produced by stromal cells regulate the differentiation, maturation and activation of neighboring mast cells. Many of the bioactive lipids are stored in membrane phospholipids as precursor forms and released spatiotemporally by phospholipase A2 (PLA2) enzymes. Through a series of studies employing gene targeting and lipidomics, several enzymes belonging to the PLA2 superfamily have been demonstrated to participate in mast cell-related diseases by mobilizing unique bioactive lipids in multiple ways. In this review, we provide an overview of our current understanding of the regulatory roles of several PLA2-driven lipid pathways in mast cell biology.
Introduction
Altered tissue sensitivity to environmental triggers contributes to the development of allergic inflammation, which is often associated with type 2 immunity (1, 2). Allergic diseases have become very common in hygienic countries, with a prevalence that has increased by 2–3-fold within the last two decades. Patients with anaphylaxis, food allergy, asthma, allergic rhinitis, and atopic eczema typically have elevated levels of serum IgE in association with activation of mast cells (3). Development of mast cells in extravascular tissues depends essentially on the stromal cytokine stem cell factor (SCF) and its receptor c-Kit, with various cytokines and adhesion molecules having accessory roles in tissue- or disease-specific contexts (4). Crosslinking of FcϵRI, a high affinity IgE receptor on the surface of mast cells, with IgE and antigen (allergen), or exposure to several IgE-independent stimuli such as substance P, which is released from TRPV1+ sensory neurons and acts on the MRGPR family receptors on mast cells (5), triggers exocytosis of granule contents (e.g., histamine and proteases) and production of various cytokines and chemokines, thereby promoting harmful allergic reactions and also participating in beneficial host defense against invading microorganisms or venom components (6). Mast cells also generate a variety of bioactive lipids called lipid mediators, which take part in fine-tuning of allergic responses by regulating the functions of various cell types. Furthermore, proper maturation and activation of mast cells are positively or negatively affected by various microenvironmental factors, including bioactive or structural lipids. For instance, the CD300 immunoreceptor family binds to structural lipids (e.g., ceramides, sphingomyelin, phosphatidylserine (PS) and phosphatidylethanolamine (PE)) as functional ligands and negatively regulates FcϵRI signaling to put a brake on excessive immediate allergic reactions (7, 8).
Lipid mediators, on which we put a specific focus in this article, are produced through specific biosynthetic pathways, are released extracellularly via diffusion, specific transporters or extracellular vesicles (EVs), act on specific receptors on target cells, and are rapidly degraded or inactivated within local tissue microenvironments. Individual lipid mediators display pleiotropic functions and can have both offensive and protective effects by acting on distinct receptor subtypes expressed on different cell types. Lipid mediators are categorized into several classes, including eicosanoids derived from ω6 arachidonic acid (AA), such as prostaglandins (PGs) and leukotrienes (LTs); specialized pro-resolving lipid mediators (SPMs) derived from ω3 eicosapentaenoic acid (EPA) or docosahexaenoic acid (DHA), such as resolvins, maresins and protectins; and lysophospholipid-derived mediators, such as lysophosphatidic acid (LPA) and platelet-activating factor (PAF) (9–12). Biosynthesis of these lipid mediators is initiated in many if not all cases by hydrolysis of membrane phospholipids by phospholipase A2s (PLA2s). The mammalian genome encodes more than 50 PLA2-related enzymes, which are classified into several structurally related families, including the cytosolic PLA2 (cPLA2), Ca2+-independent PLA2 (iPLA2), secreted PLA2 (sPLA2), PAF acetylhydrolase (PAF-AH), lysosomal PLA2, PLA-acyltransferase (PLAAT), and α/β hydrolase (ABHD) families (13). The properties and functions of individual PLA2s, as revealed by studies using knockout or transgenic mice for individual PLA2s in combination with comprehensive lipidomics, have been summarized in current reviews (14, 15).
Importantly, genetic and pharmacological studies have provided evidence that several intracellular and extracellular PLA2s uniquely regulate the maturation and functions of mast cells through driving distinct lipid pathways in vivo (16–23). These include (i) cPLA2α-driven generation of AA metabolites by activated mast cells for propagation or sequestration of allergic inflammation, (ii) PAF-AH2-driven constitutive generation of EPA/DHA metabolites for optimization of mast cell activation, (iii) sPLA2-III-driven paracrine PGD2 circuit for mast cell maturation, (iv) sPLA2-IIA-driven modulation of gut microbiota that indirectly affects mast cells, and (v) miscellaneous PLA2s whose roles in mast cells are controversial. In this article, we will make an overview of the roles of these PLA2-driven lipid pathways in mast cell biology.
Regulatory Roles of Intracellular PLA2s in Mast Cell Biology
Eicosanoid Generation by cPLA2α in Activated Mast Cells
In general, PLA2 acts as the most upstream enzyme in the biosynthetic pathways for various lipid mediators derived from membrane phospholipids (Figure 1). It is well established that group IVA cPLA2 (cPLA2α or PLA2G4A), the only PLA2 subtype that shows a striking substrate specificity for AA-containing phospholipids, is essential for stimulus-coupled release of AA from phospholipids and thereby production of eicosanoids in many cell types including mast cells (24, 25). cPLA2α has an N-terminal C2 domain, which allows translocation of the enzyme from the cytosol to perinuclear, ER and Golgi membranes in response to an increase in cytosolic Ca2+ following cell activation (24). In addition, cPLA2α is phosphorylated by mitogen-activated protein kinases (MAPKs) and possibly other kinases, an event that is essential for full activation of cPLA2α (25). Phosphatidylinositol-4,5-bisphosphate (PIP2) and ceramide-1-phosphate (C1P) modulate the subcellular localization and activation of cPLA2α (26, 27). These properties place cPLA2α as a central regulator of the stimulus-coupled generation of eicosanoids, a class of AA-derived lipid mediators including PGs and LTs.
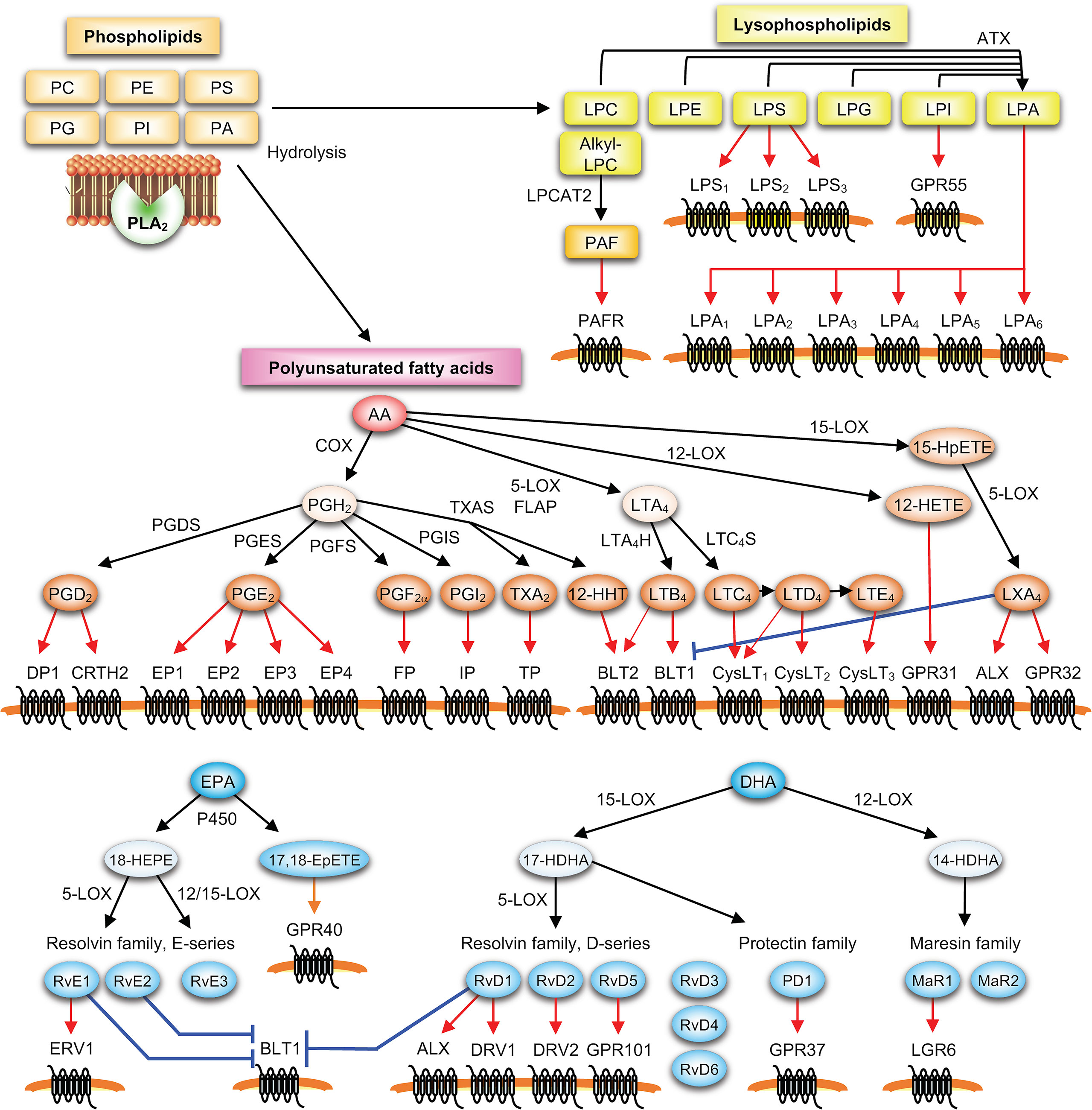
Figure 1 PLA2-driven lipid mediator pathways. Lysophospholipids (such as lysophosphatidylcholine (LPC), lysophosphatidylethanolamine (LPE), lysophosphatidylserine (LPS), lysophosphatidylglycerol (LPG), lysophosphatidylinositol (LPI) and LPA) and polyunsaturated fatty acids (such as AA, EPA and DHA) released from membrane phospholipids (PC, PE, PS, phosphatidylinositol (PI), phosphatidylglycerol (PG) and phosphatidic acid (PA)) by PLA2 are metabolized by downstream enzymes into various lipid mediators, which in turn act on their specific receptors on target cells. In the PLA2-lysophospholipid axis, various lysophospholipids are converted by autotaxin (ATX) into LPA, and alkyl-LPC is converted by LPC acyltransferase 2 (LPCAT2) into PAF. In the PLA2-PUFA axis, ω6 AA released by PLA2 is metabolized into prostanoids including PGD2, PGE2, PGF2α, PGI2, TXA2, and 12-hydroxyheptadecatrenoic acid (12-HHT) via the COX pathway involving COX-1 or COX-2 and terminal PG synthases (PGDS, PGES, PGF2α synthase (PGFS), PGI2 synthase (PGIS) and TXA2 synthase (TXAS)), or into LTB4 and cysLTs (LTC4, D4 and E4) via the 5-LOX pathway involving 5-LOX, its cofactor 5-LOX-activating protein (FLAP), and terminal LT synthases (LTA4H and LTC4S). Combined actions of 15-LOX and 5-LOX give rise to lipoxins (e.g., LXA4), an AA-derived SPM. ω3 PUFAs (e.g., EPA and DHA) are metabolized by LOXs and/or CYP450s into various SPMs including hydroxy-EPA (e.g., 18-HEPE), hydroxy-DHA (e.g., 14- and 17-HDHAs), E- and D-series resolvins (e.g., RvE1-3 and RvD1-6), protectin D1 (PD1), maresins (e.g., MaR1-2), and ω3 epoxides (e.g., 17,18-EpETE).
Activated mast cells produce LTB4, LTC4 and PGD2 as major AA-derived eicosanoids. Following FcεRI-dependent or -independent activation of mast cells, the AA released by cPLA2α from membrane phosphatidylcholine (PC) and phosphatidylethanolamine (PE) is converted by the sequential action of 5-lipoxygenase (LOX) and terminal LT synthases to LTs (the 5-LOX pathway), or by that of cyclooxygenases (COXs) and hematopoietic PGD synthase (H-PGDS) to PGD2 (the COX pathway) (Figure 2), with preferential production of PGD2 by connective-tissue mast cells (CTMCs) and LTC4 by mucosal mast cells (MMCs) (28–30). After being exported from mast cells via the specific transporter MRP1, LTC4 is converted to LTD4 and then to LTE4 by extracellular peptidases; therefore, these three LTs, which have a glutathione-derived cysteine in their structures, are often referred to as cysteinyl LTs (cysLTs) (31). Of the two COX isoforms COX-1 and -2, pre-existing COX-1 is mainly responsible for immediate PGD2 generation that occurs within a few minutes, while inducible COX-2 is responsible for delayed PGD2 generation that lasts for several hours (32, 33). IL-3-driven bone marrow-derived mast cells (BMMCs), a relatively immature mast cell population, produce LTC4 in preference to PGD2, thus resembling MMCs. Coculture of BMMCs with fibroblasts in the presence of SCF facilitates their maturation toward a CTMC-like phenotype, with an eicosanoid balance shift from LTs to PGD2 that is accompanied by increased expression of cPLA2α, COX-2, H-PGDS and LTB4 dehydrogenase (which inactivates LTB4) and by decreased expression of LTA4 hydrolase (LTA4H; LTB4 synthase) and LTC4 synthase (LTC4S) (34, 35).
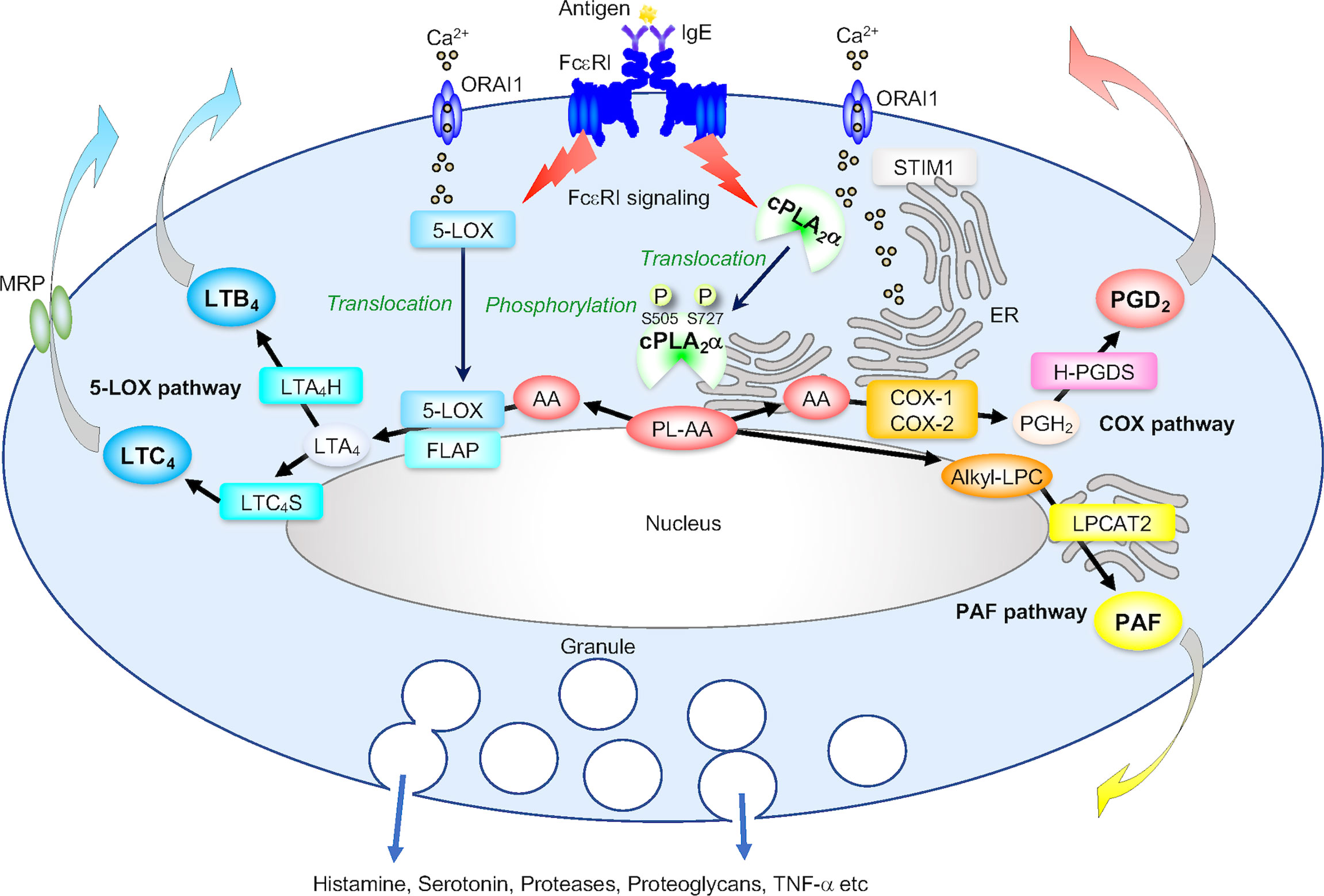
Figure 2 The cPLA2α-eicosanoid axis. (A) The role of cPLA2α in generation of PGD2 and LTs in IgE/antigen (Ag)-activated mast cells. Following FcεRI-dependent signaling that is linked to STIM1/ORAI1-driven Ca2+ influx, cPLA2α translocates from the cytosol to the perinuclear, Golgi and ER membranes, where several downstream eicosanoid-biosynthetic enzymes are also located. The activity of cPLA2α is augmented by phosphorylation at Ser505 by MAPKs, Ser727 by MAPK-activated protein kinases (MAPKAPKs), and possibly other kinases. The AA released from AA-containing phospholipids (PL-AA) by cPLA2α is then metabolized into PGD2 via the COX pathway involving COX-1 (or COX-2 if it is induced) and H-PGDS and into LTB4 and LTC4 via the 5-LOX pathway involving 5-LOX (which also translocates from the cytosol to the perinuclear membrane in response to Ca2+), FLAP (a cofactor that presents AA to 5-LOX), LTA4H and LTC4S. Alternatively, cPLA2α-generated alkyl-LPC is acetylated by LPCAT2 to PAF. These reactions occur independently of degranulation and cytokine induction.
Following FcεRI-dependent or -independent activation, BMMCs from cPLA2α-deficient (Pla2g4a–/–) mice fail to produce LTs and PGD2 as well as PAF, a lysophospholipid-derived lipid mediator that also participates in allergic responses, with no change (17) or a slight increase (36, 37) in degranulation. Pla2g4a–/– mice or WT mice treated with a cPLA2α inhibitor display reduced asthmatic responses upon pulmonary antigen challenge (16, 38). As in mice lacking cPLA2α, asthmatic responses are attenuated in mice lacking biosynthetic enzymes or receptors for LTs, PGD2, or PAF (39–45), implying a critical role of the cPLA2α-LT/PGD2/PAF axis in this pulmonary allergic disease. In contrast, passive cutaneous anaphylaxis (PCA), a model of immediate-type allergic reactions, is not altered in Pla2g4a–/– mice, likely due to simultaneous shutdown of the generation of pro-anaphylactic LTC4 and anti-anaphylactic PGD2 by mast cells (20).
LTB4 is a potent chemoattractant for leukocytes, while cysLTs promote microvascular permeability, sustained smooth muscle contraction, and mucus secretion. Among the three cysLT receptors (CysLT1~3), CysLT1 binds to LTD4 > LTC4 > LTE4, CysLT2 to LTC4 = LTD4 > LTE4, and CysLT3 to LTE4 as determined by transfection systems, although their specificity in vivo is less clear. Besides being produced by mast cells as well as by macrophages, eosinophils and basophils, cysLTs can also activate mast cells to produce contractile PGs via a mechanism involving a receptor sensitive to montelukast (46, 47), a CysLT1 antagonist that is now clinically used for treating patients with asthma (48). IL-4-dependent mast cell proliferation requires autocrine/paracrine cysLT signaling via CysLT1, and CysLT2 interacts with CysLT1 to dampen cysLT-dependent mitogenic responses of mast cells (49, 50). Recently, cysLTs have attracted attention as critical regulators of group 2 innate lymphoid cells (ILC2s). CysLTs induce CysLT1-dependent production of Th2 cytokines (IL-4, IL-5, and IL-13) by ILC2s in cooperation with the epithelial-derived cytokines IL-25 and IL-33, promoting the expansion of ILC2s, Th2 cells, and eosinophils after allergen challenge (51–53). CysLT2-driven production of IL-33 by type 2 alveolar cells leads to expansion of ILC2s, and pharmacological inhibition of CysLT2 blocks IL-33-driven mast cell activation and associated lung inflammation (54). Antigen inhalation leads to expansion of IL-25-producing airway brush cells or tuft cells, which is attenuated by genetic deletion of LTC4S or CysLT3, revealing an importance of the airway LTE4-CysLT3 axis in type 2 inflammation (55). Similarly, cysLTs produced by intestinal tuft cells cooperate with IL-25 to activate ILC2s, and tuft cell-specific deletion of cysLT generation blocks type 2 immune responses against food allergens or helminth infection (56). In the context of atopic dermatitis, tape stripping of the stratus corneum (equivalent to scratching) causes keratinocytes to systemically release IL-33, which synergizes with intestinal tuft cell-derived IL-25 and cysLTs to drive the expansion and activation of ILC2s that produce IL-4, eventually promoting mast cell expansion in the intestine (57). In addition, LTC4 derived from basophils rather than mast cells acts on CysLT2 on natriuretic polypeptide-positive (Nppb+) sensory neurons to elicit itch (58, 59), underscoring a novel mechanism for acute itch flares in atopic dermatitis.
PGD2 exerts pro- and anti-allergic functions through two PGD receptors, DP1 and CRTH2 (DP2), depending on the disease contexts. Global or mast cell-specific deletion of DP1 ameliorates asthma and anaphylaxis, which may be attributable in part to the impaired maturation of mast cells (see below) (20, 43). In contrast, DP1 deficiency increases the migration of antigen-captured dendritic cells into the draining lymph nodes, thereby exacerbating contact hypersensitivity (CHS), a Th1-dependent delayed-type allergic response (60, 61). Global or mast cell-specific deletion of H-PGDS worsens anaphylaxis (20, 62) and food allergy with mast cell hyperplasia (63). The anti-inflammatory action of PGD2 is mediated, at least in part, by its non-enzymatic conversion to 15-deoxy-PGJ2, which acts on the nuclear receptor PPARγ to attenuate pro-inflammatory NF-κB signaling (64). Mast cell-derived PGD2 is involved in ILC2 expansion via CRTH2 (65), and CRTH2-deficient mice exhibit reduced pulmonary ILC2 responses and type 2 inflammation, an event that is rescued by transfer of CRTH2-sufficient ILC2s (66). LTE4 enhances the ability of PGD2 to induce ILC2 and Th2 cells (52, 67), implying a synergistic role of the two mast cell-derived eicosanoids in promoting type 2 immunity. Activation of cPLA2α in intestinal tuft cells leads to generation of not only LTC4 (see above) but also PGD2, the latter of which increases mucus secretion by goblet cells to impede pathogen invasion (68). Thus, it seems that mast cells and tuft cells share common characteristics in that they produce both PGD2 (via the cPLA2α/COX-2/H-PGDS pathway) and LTC4 (via the cPLA2α/5-LOX/LTC4S pathway) in response to specific stimuli and participate in fine-tuning allergic responses as well as host defense against pathogens.
Although mast cells produce PGE2 minimally, this pleiotropic eicosanoid is produced by stromal cells surrounding mast cells and plays an anti-allergic role in general by acting on four types of its receptor (EP1–4). During mast cell-fibroblast coculture, the AA released by cPLA2α in mast cells is transferred to adjacent fibroblasts through the transcellular route and then metabolized there by PGE2 synthase (mPGES-1) to PGE2 (17), which acts on stromal EP3 to counteract allergic reaction. As such, mice lacking EP3 display more severe asthma, anaphylaxis, and CHS (20, 69, 70). Paradoxically, however, PGE2 directly elicits mast cell activation via EP3 by evoking Ca2+ signaling (71). Genetic deletion of mPGES-1 or pharmacological inhibition of COX-1 rather than COX-2 mimics aspirin-exacerbated respiratory disease (AERD) that is characterized by bronchoconstriction, eosinophilia, and mucus secretion upon exposure to non-steroidal anti-inflammatory drugs (NSAIDs; COX inhibitors), an event that is reversed by EP2 (or to a lesser extent EP1) agonist (72). In this AERD pathology, aspirin-induced mast cell activation and cysLT overproduction depend on the adherent interaction of platelets with granulocytes and thromboxane A2 (TXA2) signaling (72, 73). In addition, PGE2 alleviates mast cell activation (74–76), increases vascular remodeling (77), prevents eosinophilia (78, 79), reduces proliferation of ILC2s and Th2 cells (80, 81), and attenuates atopic dermatitis by reducing TSLP expression (82) via EP2 signaling. The mast cell-related eicosanoid network is illustrated in Figure 3.
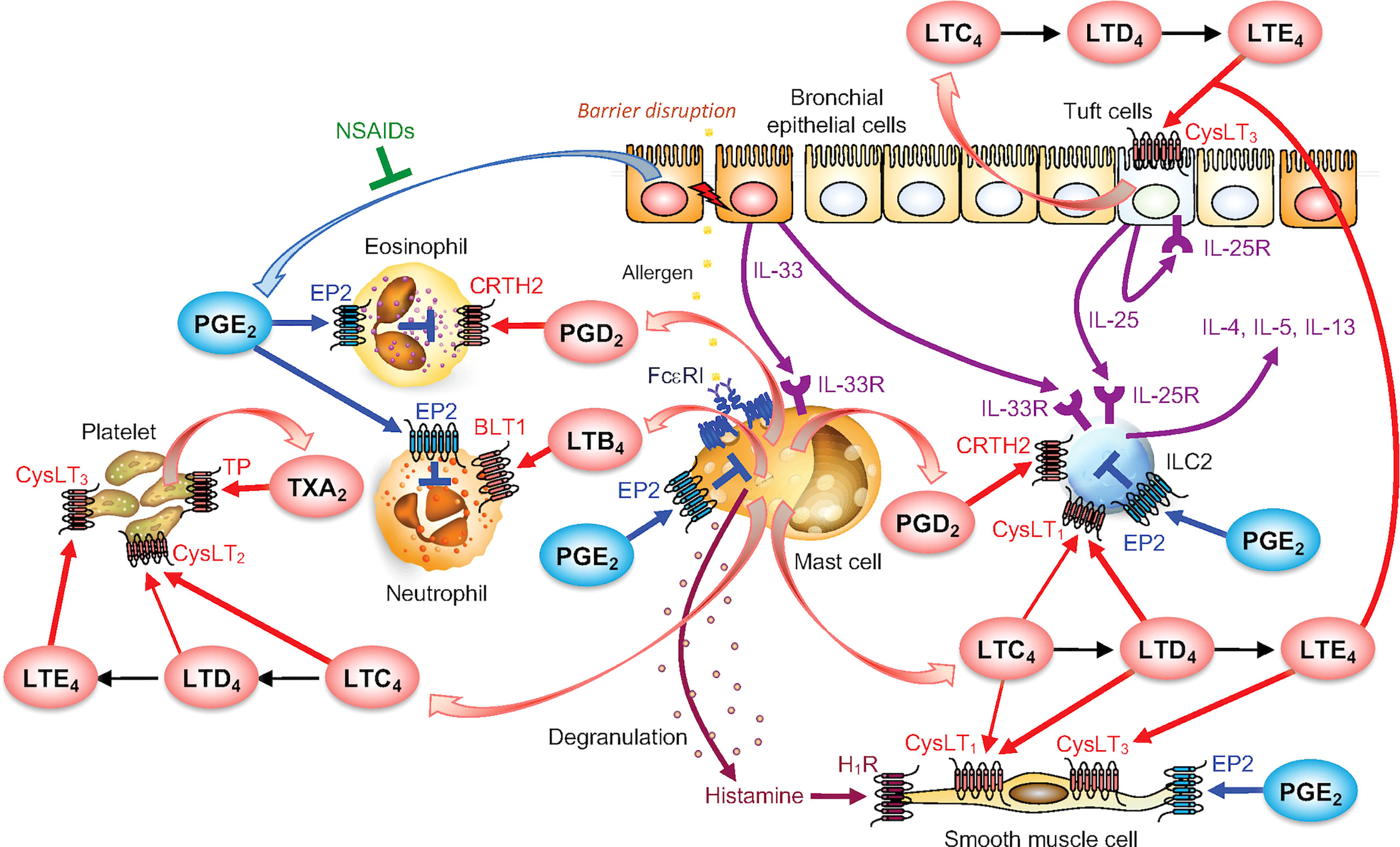
Figure 3 The mast cell-driven eicosanoid network in asthma. PGD2 and LTs produced by activated mast cells act on various cell types including leukocytes, platelets, bronchial epithelial cells and smooth muscle cells to promote allergic responses. PGE2, produced by various cells such as bronchial epithelial cells (but not mast cells), dampens allergic responses. NSAIDs (COX inhibitors) blocks PGE2 production, thereby exacerbates asthma known as AERD. For details, please see the text.
Constitutive Generation of ω3 Epoxides by PAF-AH2 in Mast Cells
PAF-AHs were originally identified as a unique group of PLA2s having the capacity to hydrolyze and thereby inactivate PAF (83, 84). Plasma-type PAF-AH (PLA2G7 or group VIIA PLA2) is a secreted protein produced by macrophages, mast cells or other sources, and is now more generally referred to as lipoprotein-associated PLA2 (Lp-PLA2), existing as a low-density lipoprotein (LDL)-bound form in human plasma (85). PAF-AH2 (group VIIB PLA2) is a cytosolic enzyme that shows significant structural homology with Lp-PLA2. Despite their names, however, there has been no confirmative evidence that Lp-PLA2 or PAF-AH2 has a protective role against allergic reactions by degrading PAF in vivo. Rather, it is now recognized that Lp-PLA2 and PAF-AH2 preferentially hydrolyze oxidized phospholipids in lipoproteins and cells, respectively. Importantly, PAF-AH2 is abundantly expressed in mast cells and contributes to constitutive (i.e., stimulus-independent) generation of unique epoxy-metabolites of ω3 fatty acids (ω3 epoxides), which prime mast cells to ensure optimal FcεRI-dependent activation (Figure 4A) and attenuates pulmonary hypertension by reducing the expression of pro-fibrotic factors (Figure 4B) in autocrine and paracrine manners, respectively (18, 19).
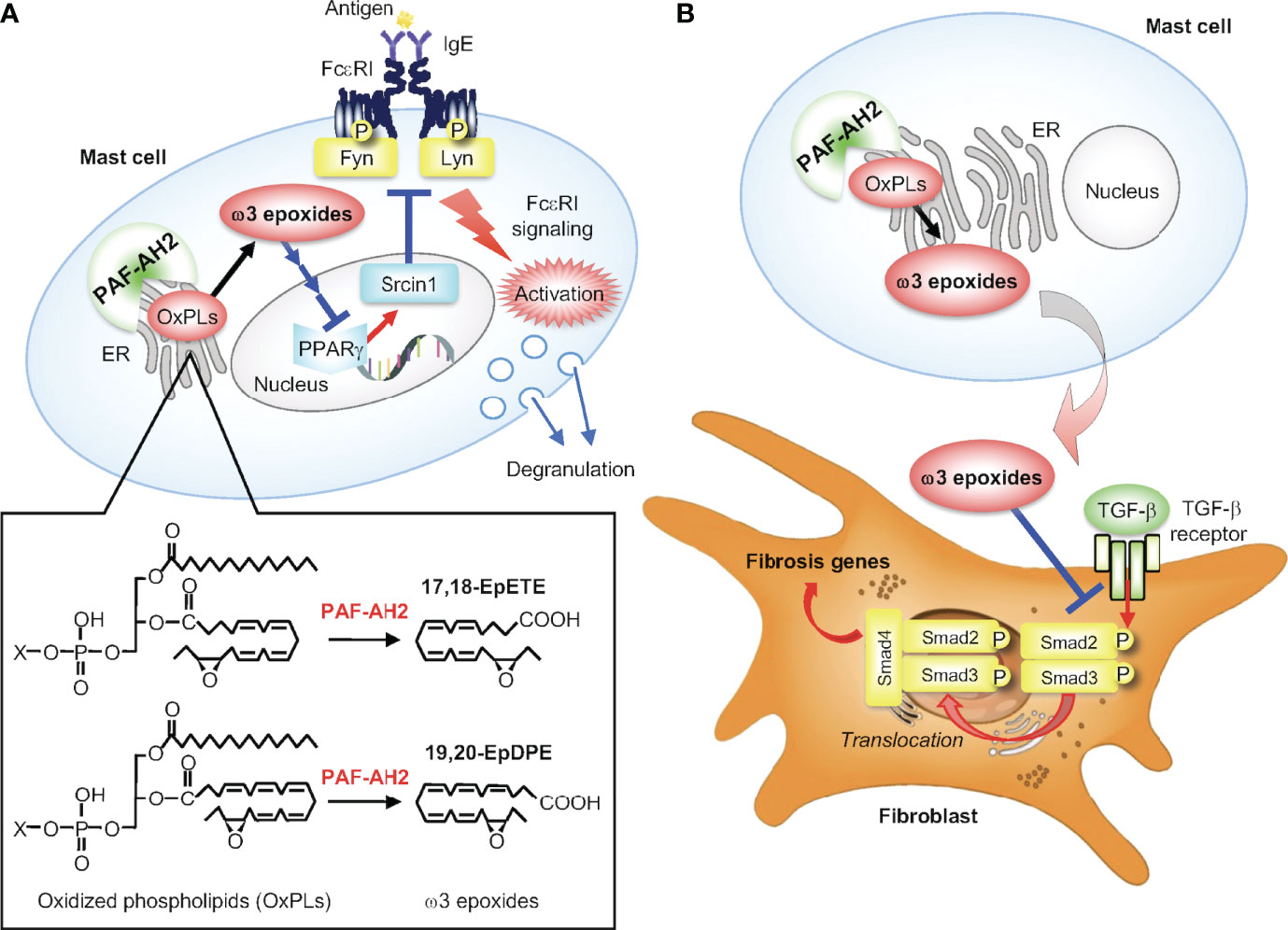
Figure 4 The PAF-AH2-ω3 epoxide axis. (A) PAF-AH2-driven constitutive generation of ω3 epoxides in mast cells and their cell-autonomous role in augmentation of FcεRI signaling. PAF-AH2 directly hydrolyzes phospholipids with oxidized fatty acids (ω3 epoxides in this case) to release free ω3 epoxides (17,18-EpETE and 19,20-EpDPE), which act on the nuclear receptor PPARγ (probably indirectly) to suppress the expression of Srcin1, a Src-inhibitory protein. This Srcin1 downregulation eventually leads to increased activation of the FcεRI-proximal Src family kinases Fyn and Lyn, thereby ensuring optimal FcεRI signaling. (B) Suppression of pulmonary hypertension by PAF-AH2-derived ω3 epoxides. The ω3 epoxides released from lung mast cells acts on neighboring fibroblasts in a paracrine manner to prevent TGF-β-driven Smad2 phosphorylation, thereby attenuating perivascular fibrosis leading to amelioration of pulmonary hypertension.
Comprehensive lipidomics profiling of BMMCs revealed that unique ω3 epoxides, namely 17,18-epoxyeicosatetraenoic acid (17,18-EpETE) and 19,20-epoxydocosapentaenoic acid (19,20-EpDPE) derived respectively from EPA and DHA, are constitutively released from the cells (18). Unlike the canonical route for eicosanoid biosynthesis, in which the AA released by cPLA2α from membrane phospholipids is metabolized by downstream enzymes to eicosanoids (see above), these ω3 epoxides are liberated directly by PAF-AH2 from ω3 epoxide-esterified phospholipids pre-existing in cell membranes (Figure 4A). Genetic or pharmacological inactivation of PAF-AH2 shuts off the constitutive release of ω3 epoxides from mast cell membranes, resulting in reduced FcεRI signaling and anaphylaxis. Supplementation of Pafah2–/– BMMCs with ω3 epoxides fully restores the defective FcεRI-dependent, but not -independent, activation. Mechanistically, ω3 epoxides augment FcεRI-driven activation of mast cells by downregulating Srcin1, a Src-inhibitory protein that counteracts the FcεRI-proximal Src-family tyrosine kinases Fyn and Lyn and thereby dampens FcϵRI signaling (18). Transcriptome analysis revealed that genes regulated by ω3 epoxides largely overlap with those regulated by a PPARγ antagonist in Pafah2–/– BMMCs, implying that the action of ω3 epoxides may involve the inactivation of PPARγ, a lipid-sensing nuclear receptor that induces Srcin1 expression (18).
In general, ω3 fatty acids including EPA and DHA play beneficial roles in various biological events. These ω3 fatty acids act by themselves on the fatty acid receptors GPR40 or GPR120 (86, 87), or are converted by LOX or cytochrome P450 (CYP450) enzymes to SPMs with potent anti-inflammatory or pro-resolving functions. Indeed, several CYP450 isoforms have the capacity to catalyze the epoxidation of EPA and DHA to yield ω3 epoxides (88). Individual SPMs act on their specific receptors, such as ERV1/ChemR23 for resolvin E1 (RvE1), DRV1/GPR32 for RvD1, DRV2/GPR18 for RvD2, GPR37 for protectin D1 (PD1), and LGR6 for maresin 1 (MaR1) (89–93), and can limit leukocyte recruitment, induce granulocyte apoptosis, enhance efferocytosis by phagocytes, facilitate the switch from M1 to M2 macrophages, promote the return of non-apoptotic cells to lymphatics and blood vessels, and help tissue repair (11, 94, 95). In this context, it seems strange that ω3 epoxides potentiate (rather than attenuate) mast cell activation and thereby exacerbate allergic responses. It should be noted, however, that the immunosuppressive action of SPMs can be disadvantageous to host defense (11, 94, 95). Conceivably, FcϵRI-induced mast cell activation might have evolved originally as a defense system against harmful venom, bacteria, and/or parasites (96, 97), where the ω3 epoxide-mediated optimization of FcϵRI signaling may be important for proper elimination of these unfavorable materials from the body and thereby maintenance of a healthy state. In a modern hygienic environment, however, this protective response has been shifting into deleterious outcomes against exposure to environmental allergens, manifesting as allergic diseases.
In contrast to their augmentative role in FcεRI-dependent mast cell activation and anaphylaxis, ω3 epoxides produced by PAF-AH2 in mast cells have a protective role in pulmonary hypertension, a fatal rare disease that causes right heart failure by elevated pulmonary arterial resistance (19). Global or mast cell-specific deletion of PAF-AH2 in mice accelerates vascular remodeling with perivascular fibrosis, resulting in exacerbation of hypoxic pulmonary hypertension. Mechanistically, 17,18-EpETE and 19,20-EpDPE produced by PAF-AH2 in lung mast cells act on stromal fibroblasts in a paracrine manner to suppress their activation by inhibiting TGF-β-driven Smad2 signaling (Figure 4B). Administration of ω3 epoxides into mice improves pulmonary hypertension by reducing advanced vascular remodeling harboring perivascular fibrosis in several models. Furthermore, the whole-exome sequencing of patients with pulmonary arterial hypertension identifies two candidate pathogenic variants of the PAFAH2 gene. Thus, the PAF-AH2–ω3 epoxide axis could be a promising therapeutic target for pulmonary hypertension. In addition, 17,18-EpETE suppresses CHS by inhibiting neutrophil migration through GPR40 (98) Thus, the actions of ω3 epoxides are context-dependent, having detrimental or beneficial effects depending on the diseases.
Plasma-type PAF-AH (PLA2G7/Lp-PLA2) is abundantly expressed in BMMCs, is secreted after FcεRI-dependent activation, and may participate in the degradation of PAF produced by these cells in an autocrine fashion (99). Interestingly, a comprehensive transcriptome analysis of various mast cell populations demonstrated that the expression level of the Pla2g7 gene in skin mast cells is much lower than that in tongue, tracheal, esophageal and peritoneal mast cells (100). Although the physiological significance of the low expression of this enzyme in skin mast cells is unclear, it might avoid rapid degradation of PAF produced by these cells or neighboring cells in a local skin niche. In the aforementioned pulmonary hypertension model, Pla2g7–/– mice do not display any noticeable phenotype (19), implying the segregated role of the two (plasma-type and intracellular) PAF-AH isoforms in this disease.
Regulatory Roles of Extracellular PLA2s in Mast Cell Biology
General View of sPLA2s
The sPLA2 family comprises Ca2+-dependent, low-molecular-mass enzymes with a conserved His-Asp catalytic dyad. There are 11 mammalian sPLA2s (catalytically active IB, IIA, IIC, IID, IIE, IIF, III, V, X and XIIA and inactive XIIB), which are structurally subdivided into group I/II/V/X, group III, and group XII branches (101). Individual sPLA2s exhibit distinct tissue distributions and exert their specific functions in lipid mediator-dependent or -independent fashions (102–105). In extracellular milieus, sPLA2s act on the plasma membrane of activated, damaged, or dying cells (rather than that of resting cells) and also on non-cellular phospholipid components, such as dietary food, lipoproteins, lung surfactant, EVs, and membranes of invading microbes, as hydrolytic targets. Although the expression and potential functions of sPLA2s in mast cells had been reported in previous studies (106–113), the results should be interpreted with caution since many of them relied on the strategies employing overexpression or exogenous addition of super-physiologic levels of sPLA2s, which might not precisely reflect pathophysiologic situations in vivo. Beyond this caveat, gene targeting studies have provided unequivocal evidence for the involvement of several sPLA2s in the regulation of mast cells in cell-autonomous and non-autonomous ways. Our comprehensive phenotypic screening of various sPLA2 knockout strains, with mast cell-dependent PCA reaction in vivo and BMMC functions ex vivo as readouts, has revealed that mice null for sPLA2-III and -IIA, but not those for sPLA2-IB, -IID, -IIE, -IIF, -V and -X, displayed notable alterations in the maturation and/or functions of mast cells (20), as described below.
Regulation of Mast Cell Maturation by sPLA2-III Through the Paracrine PGD2 Pathway
Bee venom group III PLA2 (bvPLA2), when injected into mouse skin, directly activates mast cells likely through hydrolysis of membrane phospholipids to give rise to lysophospholipids, whose massive accumulation can cause lysis of all the cells (including mast cells) in the milieu due to their detergent-like nature leading to release of the alarmin IL-33 and thereby activation of ILC2s and then Th2 cells (114). The aggravated Th2 response by bvPLA2 can be considered as a protective mechanism against future exposure to this noxious venom component. Administration of human sPLA2-III, the sole mammalian homolog of bvPLA2, into mouse skin also elicits mast cell activation (20). Endogenous sPLA2-III is stored in and released from secretory granules of mouse and human mast cells. Importantly, mast cell-dependent passive and active anaphylactic responses are markedly reduced in mice lacking sPLA2-III (Pla2g3–/–) and conversely augmented in mice with transgenic overexpression of human sPLA2-III (PLA2G3TG) (20). Notably, mast cells in Pla2g3–/– mice are numerically normal but morphologically and functionally immature, indicating that sPLA2-III does not merely act as a mast cell activator, but also facilitates mast cell maturation. In fact, histamine and protease contents in secretory granules, expression of mast cell maturation markers (e.g., histidine decarboxylase (histamine synthase), mast cell proteases, and H-PGDS), and FcεRI-dependent and even -independent activation are considerably lower in Pla2g3–/– mast cells than in Pla2g3+/+ mast cells. These phenotypes are mast cell-autonomous, since BMMCs from Pla2g3–/– mice fail to reconstitute the anaphylactic response after their transfer into mast cell-deficient KitW-sh/W-sh mice, and since mast cell-specific deletion of sPLA2-III also leads to similar defects in mast cell maturation and anaphylaxis (20, 21). Importantly, the perturbed mast cell maturation and anaphylaxis in Pla2g3–/– mice are recapitulated in mice lacking lipocalin-type PGD2 synthase (L-PGDS), which is expressed in stromal fibroblasts, or those lacking the PGD2 receptor DP1, which is induced in maturing mast cells. Thus, sPLA2-III secreted from mast cells hydrolyzes phospholipids in adjacent fibroblasts to release AA, which is then converted by fibroblastic L-PGDS to PGD2 that acts on DP1 on mast cells to promote their proper maturation (Figure 5A). This sPLA2-III-L-PGDS-DP1 paracrine circuit highlights a new aspect of PGD2-DP1 signaling in the regulation of mast cell maturation and thereby allergy and provides evidence for the long-standing proposal that sPLA2 acts as a paracrine coordinator of eicosanoid production in tissue microenvironments (20). Since EVs serve as a better hydrolytic target of sPLA2s (115, 116), it is also possible that sPLA2-III may act on mast cell-secreted EVs to release AA, which is incorporated into fibroblasts and utilized for L-PGDS-driven PGD2 generation.
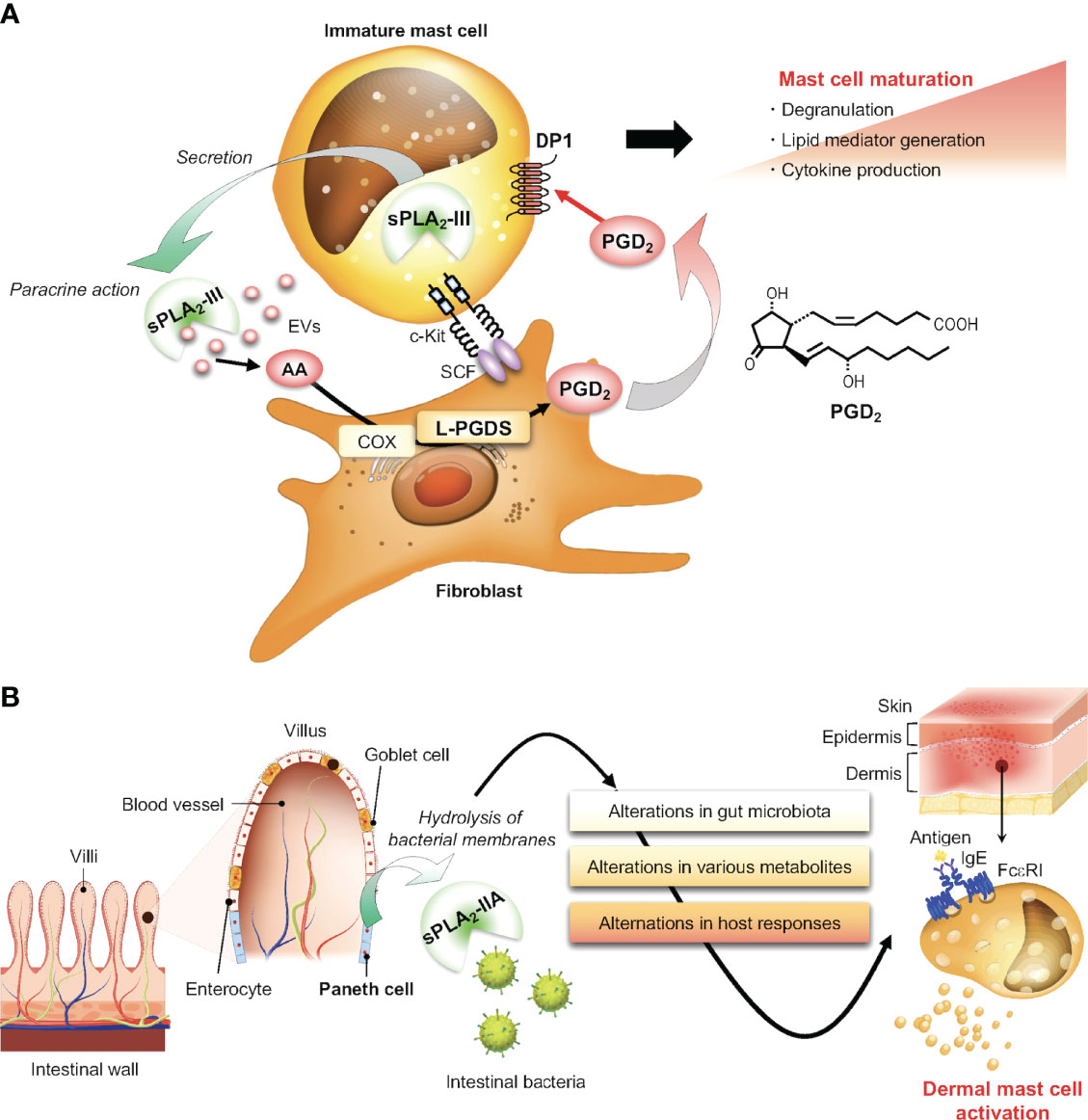
Figure 5 Regulatory roles of sPLA2s in mast cell biology. (A) The sPLA2-III-driven paracrine PGD2 circuit for proper mast cell maturation. sPLA2-III secreted from immature mast cells hydrolyzes phospholipids in adjacent fibroblast membranes or possibly in mast cell-derived EVs to release AA. This AA is metabolized by fibroblastic COX/L-PGDS to PGD2, which in turn acts on DP1 on mast cells to promote mast cell maturation. (B) Non-canonical action of sPLA2-IIA on mast cells via shaping of the gut microbiota. sPLA2-IIA, a potent bactericidal protein, is secreted from intestinal Paneth cells and modulates the gut microbiota. This event has systemic effects on immunity and metabolism, thereby secondarily affecting mast cell activation in distal tissues.
Furthermore, mast cell-specific Pla2g3-deficient mice, as well as mast cell-deficient KitW-sh mice reconstituted with mast cells prepared from global Pla2g3–/– mice, display a significant reduction of irritant contact dermatitis (ICD) and an aggravation of Th1-dependent CHS (21). The increased CHS response by sPLA2-III deficiency in mast cells depends, at least in part, on the reduced expression of H-PGDS and thereby reduced production of PGD2 due to immaturity of mast cells. During severe CHS responses, mast cells represent a source of IL-2 and IL-10, which amplify recruitment, maintenance, and function of Treg cells that limit ear swelling and epidermal hyperplasia (117–120). H-PGDS deficiency in mast cells also abrogates the suppressive effect of mast cells on CHS, indicating that, in addition to the cytokines IL-2 and IL-10, the lipid mediator PGD2 serves as another negative regulator of the CHS responses. In support, mice lacking DP1 also display an exacerbation of CHS by affecting the expression of IL-10 in dendritic cells (60, 61). Taken together, sPLA2-III, which is secreted from mast cells, promotes mast cell maturation through the lipid-driven functional interaction with stromal fibroblasts, thereby facilitating acute anaphylactic and ICD reactions and limiting delayed CHS response.
sPLA2-IIA Regulates Mast Cells Through Shaping of the Gut Microbiota
sPLA2-IIA (PLA2G2A) is a prototypic sPLA2 that is highly upregulated in various human tissues during inflammation such as rheumatoid arthritis, sepsis, and COVID-19 infection (121–123). While sPLA2-IIA promotes sterile inflammation by mobilizing pro-inflammatory lipid mediators or DAMPs (danger-associated molecular patterns) from EVs as an “inflammatory sPLA2” (115), it also efficiently degrades bacterial membranes (Gram-positive in particular), thereby playing a protective role against bacterial infection as a “bactericidal sPLA2” (124). In the context of mast cell biology, sPLA2-IIA was partially purified from rat mastocytoma RBL-2H3 cells (125), was detected immunohistochemically in secretory granules of rat serosal mast cells (126), triggered histamine release or PGD2 production by rat serosal mast cells when added exogenously at high concentrations (106, 107), and enhanced FcεRI-induced degranulation when overexpressed in RBL-2H3 cells (110, 111). However, as the Pla2g2a gene is naturally disrupted in C57BL/6 and 129 strains due to a natural frameshift mutation (127, 128), it had been difficult to evaluate the precise in vivo functions of endogenous sPLA2-IIA using a standard knockout strategy. Even in BALB/c mice, a strain that has an intact Pla2g2a gene (127), sPLA2-IIA expression is highly restricted to intestinal Paneth cells (129, 130). Although a trace level of Pla2g2a mRNA is induced in BALB/c-derived BMMCs after stimulation with SCF plus accessory cytokines in vitro (131), there has been no follow-up study showing that sPLA2-IIA is substantially expressed in mouse mast cells in vivo. This expression profile of sPLA2-IIA in BALB/c mice is in marked contrast to that in other animal species including humans and rats, in which sPLA2-IIA is expressed or induced in many tissues. Beyond these limitations, sPLA2-IIA-depleted (Pla2g2a–/–) mice on the BALB/c background are best suited for analyzing the role of endogenous sPLA2-IIA in the intestine. Importantly, this new mouse model has unveiled a previously unrecognized, non-canonical action of intestinal sPLA2-IIA on mast cells via shaping of the gut microbiota.
Despite the restricted expression of sPLA2-IIA in the intestine, its genetic deletion unexpectedly leads to unusual changes in mast cell degranulation in distal skin. In a model of carcinogen-induced skin cancer, tumor development is markedly reduced, accompanied by a reduction of degranulated mast cells in the tumor tissue, in Pla2g2a–/– mice relative to Pla2g2a+/+ mice (22). Surprisingly, cohousing of Pla2g2a–/– and Pla2g2a+/+ mice in the same cages, which results in mixing of the microbiota between the genotypes through coprophagia, abolishes the skin cancer-related phenotypes. Of more interest, IgE/antigen-induced PCA is uniquely altered in Pla2g2a–/– mice depending on housing conditions; when Pla2g2a+/+ and Pla2g2a–/– mice are housed in two different animal facilities, the PCA response in Pla2g2a–/– mice is reduced in one facility, while it is conversely elevated in the other facility, relative to Pla2g2a+/+ mice (23). Furthermore, in both facilities, the opposite PCA phenotypes in Pla2g2a–/– mice are lost when they are cohoused with Pla2g2a+/+ mice. Thus, the PCA phenotypes in Pla2g2a–/– mice are greatly influenced by housing conditions, implying that sPLA2-IIA, primary expressed in intestinal Paneth cells that secrete various antimicrobial peptides, contributes to shaping of the gut microbiota through its bactericidal activity, thereby secondarily affecting mast cell fate and associated allergic reaction in distal skin. Indeed, metagenome analysis of the stool revealed that several bacterial genera such as Gram-positive Lachnospiraceae and Ruminococcaceae and Gram-negative Helicobacteraceae and Prevotellaceae are differently distributed in Pla2g2a+/+ and Pla2g2a–/– mice, and there is a better correlation between distinct PCA responses and Ruminococcaceae and Mucispirillum abundances in the two facilities. The alteration in gut microbiota in Pla2g2a–/– BALB/c mice, as well as in PLA2G2A-transgenic C57BL/6 mice in which human sPLA2-IIA is overexpressed systemically, also impact the severity of arthritis and psoriasis (22, 132). Thus, sPLA2-IIA acts as a host factor that is primarily expressed in the intestine and contributes to shaping of the gut microbiota, whose disturbance by Pla2g2a deletion or overexpression secondarily affects various diseases including those involving mast cells in proximal and distal tissues (Figure 5B). This concept opens a new avenue for the action modes of this classical sPLA2 and might be applicable to other sPLA2 isoforms expressed in the gastrointestinal tract or even in other anatomical sites such as the respiratory tract and skin.
Nonetheless, looking back the classical view that sPLA2-IIA is expressed in mast cells of rat and human, mast cell-autonomous roles of sPLA2-IIA should be reconsidered. Related to the observations that forcible overexpression or exogenous addition of sPLA2-IIA resulted in enhanced mast cell degranulation as described above (106–108, 110, 111), sPLA2 inhibitors suppressed degranulation by rat serosal mast cells (107) or LTC4 production by human lung mast cells (112), although the inhibitors used in those studies were not strictly specific for sPLA2-IIA. Alternatively, sPLA2-IIA released from activated mast cells, like that released from other cells such as platelets, leukocytes and epithelial cells, might contribute to propagation of inflammation by mobilizing lipid mediators from EVs (133), host defense against infection by degrading bacterial membranes (124), or regulation of cellular signaling by acting as a ligand for the sPLA2 receptor PLA2R1 (130, 134).
Miscellaneous Roles of Other PLA2s in Mast Cell Biology
Several studies have demonstrated the potential roles of sPLA2-V (PLA2G5) and sPLA2-X (PLA2G10) in mast cells. Reportedly, sPLA2-V is released from antigen-activated BMMCs and then acts on neighboring fibroblasts to augment COX-1-dependent PGD2 biosynthesis (135–137), is localized to the perinuclear area in BMMCs (131), and augments FcεRI-induced PGD2 and LTC4 production when overexpressed in RBL-2H3 cells (110, 111). TLR2-induced, COX-2-dependent delayed PGD2 generation is partially reduced in BMMCs from sPLA2-V-deficient (Pla2g5–/–) mice, where sPLA2-V may act in cooperation with cPLA2α (138). In addition, sPLA2-X is detected in BMMCs, and IL-13 induction in response to IL-33 is substantially impaired in BMMCs from sPLA2-X-deficient (Pla2g10–/–) mice (139). Importantly, Pla2g5–/– and Pla2g10–/– mice are both protected from airway inflammation induced by allergen challenge through the mechanisms involving ILC2 cells, M2 macrophages, eosinophils, airway epithelial cells, and possibly mast cells (139–145). However, other studies showed that the expression levels of sPLA2-V and -X in BMMCs are rather low and that FcεRI-induced BMMC activation ex vivo and PCA reaction in vivo are not significantly affected in Pla2g5–/– and Pla2g10–/– mice (17, 20, 146). To reconcile these inconsistencies, it is necessary to clarify whether these two sPLA2s are expressed in a certain population of mast cells and play specific roles therein under particular pathophysiological conditions.
Several lines of evidence argue the involvement of mast cells in cardiometabolic diseases (147). Since activated mast cells produce PGD2 and LTB4, which have been implicated in cardiovascular pathology including atherosclerosis (148, 149), it is likely that cPLA2α contributes to the disease by supplying these eicosanoids in atherosclerotic plaques. Indeed, global Pla2g4a–/– mice are protected from the development of atherosclerosis (150). However, the contribution of cPLA2α expressed in mast cells to the disease remains unknown and should be confirmed using mast cell-specific Pla2g4a–/– mice. Modified or oxidized low-density lipoprotein (LDL) has been shown to induce mast cell activation, resulting in cytokine secretion and subsequent leucocyte recruitment, presumably through TLR4 (151). As a component of modified LDL, the lysophospholipid mediator LPA can induce mast cell activation, resulting in the release of tryptase and chemokines (152). Many LPA species could be detected in the atherosclerotic lesion, where mast cells also reside (152, 153). Since several sPLA2s have the capacity to generate modified LDL with a pro-atherogenic potential in vitro (154–157), it is tempting to speculate that LPA produced in LDL by these sPLA2s may contribute to the development of atherosclerosis through activating aortic mast cells. Although several studies using mice overexpressing sPLA2-IIA or those lacking sPLA2-V or sPLA2-X have proposed the potential roles of these sPLA2s in atherosclerosis, none of them has provided evidence that these sPLA2s generate modified, pro-atherogenic LDL in vivo (158–162). Therefore, it is still unclear whether sPLA2s could promote atherosclerosis development by modifying LDL and generating LPA, and if so, which sPLA2 isoform(s) would be truly responsible for this event in the context of mast cell activation.
Group IVD cPLA2 (cPLA2δ or PLA2G4D) was initially identified as a keratinocyte-specific cPLA2 isoform that is highly induced during psoriasis (163). It has recently been shown that cPLA2δ is expressed in mast cells of psoriatic patients and is released extracellularly via mast cell-derived EVs to be transferred into adjacent Langerhans cells (164). The EV-driven cPLA2δ captured by Langerhans cells generates neolipid antigens, which are then presented on CD1a to activate lipid-specific CD1a-reactive T cells, leading to induction of the Th17 cytokines IL-17A and IL-22. Although these results have provided a model whereby cPLA2δ promotes psoriasis pathology, it remains unclear which lipid metabolites produced by cPLA2δ can act as neolipid antigens. Since cPLA2δ exhibits PLA1 activity in preference to PLA2 activity (165), certain lipid metabolites generated by the PLA1 reaction might underlie the function of cPLA2δ. Further, given that cPLA2δ is expressed in epidermal keratinocytes rather than in mast cells and that CD1a is present in humans but not in mice, the regulatory roles of cPLA2δ in psoriasis or other skin diseases such as atopic dermatitis in the context of mast cells need further exploration.
Mast cells also express group VIA Ca2+-independent PLA2 (iPLA2β, also known as PLA2G6 or PNPLA9). Pharmacologic inhibition of iPLA2β by bromoenol lactone (BEL), a well-known iPLA2 inhibitor, attenuates IgE/antigen-stimulated mast cell exocytosis (166). However, mice deficient in iPLA2β (Pla2g6-/-) show a normal PCA response in vivo, and Pla2g6-/- BMMCs exhibit normal degranulation, eicosanoid generation, and cytokine expression in vitro (17), arguing against the contribution of iPLA2β to mast cell development and functions. Thus, caution should be exercised when interpreting the results obtained from studies using BEL or other iPLA2 inhibitors. Alternatively, considering that the iPLA2 family includes nine isoforms, some BEL-sensitive iPLA2 isoform(s) other than iPLA2β, such as group VIB iPLA2γ (PNPLA8) which is expressed in BMMCs more abundantly than iPLA2β (18), might be involved in the regulation of mast cells.
Interestingly, silencing of adipose triglyceride lipase (ATGL, also referred to as PNPLA2 or iPLA2ζ), a member of the iPLA2 family that plays an essential role in lipolysis and thereby energy metabolism by hydrolyzing triglycerides stored in lipid droplets (167), results in the reduction of PGD2 and LTC4 synthesis more efficiently than silencing of cPLA2α in human mast cells derived from blood CD34+ progenitors (168). These results suggest that the AA released from triglycerides by ATGL is coupled with eicosanoid synthesis in mast cells, underscoring a functional link between energy homeostasis and eicosanoid signaling. However, given the essential role of cPLA2α in eicosanoid generation in mast cells as revealed by studies using Pla2g4a-/- mice (see above), the following possibilities should also be taken into consideration: (i) mouse and human mast cells might have distinct dependence on cPLA2α and ATGL; (ii) the AA released by ATGL from lipid droplets might be firstly incorporated into membrane phospholipids by lysophospholipid acyltransferases and then cleaved out by cPLA2α toward eicosanoid synthesis; or (iii) ATGL-mediated energy supply might be required for appropriate cPLA2α-driven eicosanoid generation. Also, it is important to clarify whether ATGL in mast cells is involved in the regulation of eicosanoid synthesis or other effector functions under certain in vivo conditions.
Concluding Remarks
In this article, we have highlighted current understanding of the pathophysiological roles of several PLA2s and associated bioactive lipids in mast cell biology, focusing mainly on the findings obtained from studies using gene-manipulated mice in combination with comprehensive lipidomics. As a future prospect, it is important to translate these findings obtained from experimental animals into humans. It is possible that the functions of mast cells may also be affected by other PLA2s or bioactive lipids not described in this article, by bioenergetics coupled with lipogenesis, lipolysis and fatty acid β-oxidation, and by lipid composition in membrane microdomains that could affect signal potency through FcϵRI or other receptors. A full understanding of lipid networks in relation to mast cells, allergy, and other mast cell-dependent biological events should be further elucidated using advanced techniques such as spatiotemporal lipid imaging, untargeted lipidomics, and novel pharmacological tools to manipulate the activity or expression of particular PLA2 subtypes that would have a potential to sequester allergic or other diseases. Further research will lead to a better understanding of the overall picture of the regulation of mast cells by lipids, hopefully allowing the prophylactic and/or therapeutic application of novel agents that target specific PLA2-driven lipid pathways to human diseases.
Author Contributions
MM and YT wrote the manuscript and prepared Figures. All authors have read and agreed to the published version of the manuscript.
Funding
This work was supported by Grants-in-Aid for Scientific Research JP19K22483 and JP20H05691 (to MM) and JP18K06624 (to YT) from Japan Society for the Promotion of Science, and AMED-CREST JP21gm1210013 from the Japan Agency for Medical Research and Development (to MM).
Conflict of Interest
The authors declare that the research was conducted in the absence of any commercial and financial relationships that could be construed as a potential conflict of interest.
Publisher’s Note
All claims expressed in this article are solely those of the authors and do not necessarily represent those of their affiliated organizations, or those of the publisher, the editors and the reviewers. Any product that may be evaluated in this article, or claim that may be made by its manufacturer, is not guaranteed or endorsed by the publisher.
References
1. Zheng T, Yu J, Oh MH, Zhu Z. The Atopic March: Progression From Atopic Dermatitis to Allergic Rhinitis and Asthma. Allergy Asthma Immunol Res (2011) 3(2):67–73. doi: 10.4168/aair.2011.3.2.67
2. Dainichi T, Kitoh A, Otsuka A, Nakajima S, Nomura T, Kaplan DH, et al. The Epithelial Immune Microenvironment (EIME) in Atopic Dermatitis and Psoriasis. Nat Immunol (2018) 19(12):1286–98. doi: 10.1038/s41590-018-0256-2
3. Devereux G. The Increase in the Prevalence of Asthma and Allergy: Food for Thought. Nat Rev Immunol (2006) 6(11):869–74. doi: 10.1038/nri1958
4. Gurish MF, Austen KF. Developmental Origin and Functional Specialization of Mast Cell Subsets. Immunity (2012) 37(1):25–33. doi: 10.1016/j.immuni.2012.07.003
5. Serhan N, Basso L, Sibilano R, Petitfils C, Meixiong J, Bonnart C, et al. House Dust Mites Activate Nociceptor-Mast Cell Clusters to Drive Type 2 Skin Inflammation. Nat Immunol (2019) 20(11):1435–43. doi: 10.1038/s41590-019-0493-z
6. Galli SJ, Tsai M. IgE and Mast Cells in Allergic Disease. Nat Med (2012) 18(5):693–704. doi: 10.1038/nm.2755
7. Izawa K, Yamanishi Y, Maehara A, Takahashi M, Isobe M, Ito S, et al. The Receptor LMIR3 Negatively Regulates Mast Cell Activation and Allergic Responses by Binding to Extracellular Ceramide. Immunity (2012) 37(5):827–39. doi: 10.1016/j.immuni.2012.08.018
8. Kitaura J, Murakami M. Positive and Negative Roles of Lipids in Mast Cells and Allergic Responses. Curr Opin Immunol (2021) 72:186–95. doi: 10.1016/j.coi.2021.06.001
9. Shimizu T. Lipid Mediators in Health and Disease: Enzymes and Receptors as Therapeutic Targets for the Regulation of Immunity and Inflammation. Annu Rev Pharmacol Toxicol (2009) 49:123–50. doi: 10.1146/annurev.pharmtox.011008.145616
10. Narumiya S, Furuyashiki T. Fever, Inflammation, Pain and Beyond: Prostanoid Receptor Research During These 25 Years. FASEB J (2011) 25(3):813–8. doi: 10.1096/fj.11-0302ufm
11. Serhan CN. Pro-Resolving Lipid Mediators are Leads for Resolution Physiology. Nature (2014) 510(7503):92–101. doi: 10.1038/nature13479
12. Kano K, Aoki J, Hla T. Lysophospholipid Mediators in Health and Disease. Annu Rev Pathol (2022) 17:459–83. doi: 10.1146/annurev-pathol-050420-025929
13. Murakami M. Novel Functions of Phospholipase A2s: Overview. Biochim Biophys Acta Mol Cell Biol Lipids (2019) 1864(6):763–5. doi: 10.1016/j.bbalip.2019.02.005
14. Murakami M. Lipoquality Control by Phospholipase A2 Enzymes. Proc Jpn Acad Ser B Phys Biol Sci (2017) 93(9):677–702. doi: 10.2183/pjab.93.043
15. Murakami M, Sato H, Taketomi Y. Updating Phospholipase A2 Biology. Biomolecules (2020) 10(10):1457. doi: 10.3390/biom10101457
16. Uozumi N, Kume K, Nagase T, Nakatani N, Ishii S, Tashiro F, et al. Role of Cytosolic Phospholipase A2 in Allergic Response and Parturition. Nature (1997) 390(6660):618–22. doi: 10.1038/37622
17. Ueno N, Taketomi Y, Yamamoto K, Hirabayashi T, Kamei D, Kita Y, et al. Analysis of Two Major Intracellular Phospholipases A2 (PLA2) in Mast Cells Reveals Crucial Contribution of Cytosolic PLA2α, Not CA2+-Independent PLA2β, to Lipid Mobilization in Proximal Mast Cells and Distal Fibroblasts. J Biol Chem (2011) 286(43):37249–63. doi: 10.1074/jbc.M111.290312
18. Shimanaka Y, Kono N, Taketomi Y, Arita M, Okayama Y, Tanaka Y, et al. Omega-3 Fatty Acid Epoxides are Autocrine Mediators That Control the Magnitude of IgE-Mediated Mast Cell Activation. Nat Med (2017) 23(11):1287–97. doi: 10.1038/nm.4417
19. Moriyama H, Endo J, Kataoka M, Shimanaka Y, Kono N, Sugiura Y, et al. Omega-3 Fatty Acid Epoxides Produced by PAF-AH2 in Mast Cells Regulate Pulmonary Vascular Remodeling. Nat Commun (2022) 13(1):3013. doi: 10.1038/s41467-022-30621-z
20. Taketomi Y, Ueno N, Kojima T, Sato H, Murase R, Yamamoto K, et al. Mast Cell Maturation Is Driven via a Group III Phospholipase A2-Prostaglandin D2-DP1 Receptor Paracrine Axis. Nat Immunol (2013) 14(6):554–63. doi: 10.1038/ni.2586
21. Taketomi Y, Endo Y, Higashi T, Murase R, Ono T, Taya C, et al. Mast Cell-Specific Deletion of Group III Secreted Phospholipase A2 Impairs Mast Cell Maturation and Functions. Cells (2021) 10(7):1691. doi: 10.3390/cells10071691
22. Miki Y, Taketomi Y, Kidoguchi Y, Yamamoto K, Muramatsu K, Nishito Y, et al. Group IIA Secreted Phospholipase A2 Controls Skin Carcinogenesis and Psoriasis by Shaping the Gut Microbiota. JCI Insight (2022) 7(2):e152611. doi: 10.1172/jci.insight.152611
23. Taketomi Y, Miki Y, Murakami M. Old But New: Group IIA Phospholipase A2 as a Modulator of Gut Microbiota. Metabolites (2022) 12(4):352. doi: 10.3390/metabo12040352
24. Clark JD, Lin LL, Kriz RW, Ramesha CS, Sultzman LA, Lin AY, et al. A Novel Arachidonic Acid-Selective Cytosolic PLA2 Contains a Ca2+-Dependent Translocation Domain With Homology to PKC and GAP. Cell (1991) 65(6):1043–51. doi: 10.1016/0092-8674(91)90556-e
25. Lin LL, Wartmann M, Lin AY, Knopf JL, Seth A, Davis RJ. cPLA2 is Phosphorylated and Activated by MAP Kinase. Cell (1993) 72(2):269–78. doi: 10.1016/0092-8674(93)90666-e
26. Casas J, Gijon MA, Vigo AG, Crespo MS, Balsinde J, Balboa MA. Phosphatidylinositol 4,5-Bisphosphate Anchors Cytosolic Group IVA Phospholipase A2 to Perinuclear Membranes and Decreases its Calcium Requirement for Translocation in Live Cells. Mol Biol Cell (2006) 17(1):155–62. doi: 10.1091/mbc.e05-06-0545
27. Stahelin RV, Subramanian P, Vora M, Cho W, Chalfant CE. Ceramide-1-Phosphate Binds Group IVA Cytosolic Phospholipase A2via a Novel Site in the C2 Domain. J Biol Chem (2007) 282(28):20467–74. doi: 10.1074/jbc.M701396200
28. Murakami M, Austen KF, Arm JP. The Immediate Phase of C-Kit Ligand Stimulation of Mouse Bone Marrow-Derived Mast Cells Elicits Rapid Leukotriene C4 Generation Through Posttranslational Activation of Cytosolic Phospholipase A2 and 5-Lipoxygenase. J Exp Med (1995) 182(1):197–206. doi: 10.1084/jem.182.1.197
29. Murakami M, Matsumoto R, Urade Y, Austen KF, Arm JP. C-Kit Ligand Mediates Increased Expression of Cytosolic Phospholipase A2, Prostaglandin Endoperoxide Synthase-1, and Hematopoietic Prostaglandin D2 Synthase and Increased IgE-Dependent Prostaglandin D2 Generation in Immature Mouse Mast Cells. J Biol Chem (1995) 270(7):3239–46. doi: 10.1074/jbc.270.7.3239
30. Murakami M, Austen KF, Bingham CO 3rd, Friend DS, Penrose JF, Arm JP. Interleukin-3 Regulates Development of the 5-Lipoxygenase/Leukotriene C4 Synthase Pathway in Mouse Mast Cells. J Biol Chem (1995) 270(39):22653–6. doi: 10.1074/jbc.270.39.22653
31. Kanaoka Y, Boyce JA. Cysteinyl Leukotrienes and Their Receptors: Cellular Distribution and Function in Immune and Inflammatory Responses. J Immunol (2004) 173(3):1503–10. doi: 10.4049/jimmunol.173.3.1503
32. Murakami M, Matsumoto R, Austen KF, Arm JP. Prostaglandin Endoperoxide Synthase-1 and -2 Couple to Different Transmembrane Stimuli to Generate Prostaglandin D2 in Mouse Bone Marrow-Derived Mast Cells. J Biol Chem (1994) 269(35):22269–75. doi: 10.1016/S0021-9258(17)31786-6
33. Murakami M, Bingham CO 3rd, Matsumoto R, Austen KF, Arm JP. IgE-Dependent Activation of Cytokine-Primed Mouse Cultured Mast Cells Induces a Delayed Phase of Prostaglandin D2 Generation via Prostaglandin Endoperoxide Synthase-2. J Immunol (1995) 155(9):4445–53.
34. Ogasawara T, Murakami M, Suzuki-Nishimura T, Uchida MK, Kudo I. Mouse Bone Marrow-Derived Mast Cells Undergo Exocytosis, Prostanoid Generation, and Cytokine Expression in Response to G Protein-Activating Polybasic Compounds After Coculture With Fibroblasts in the Presence of c-Kit Ligand. J Immunol (1997) 158(1):393–404.
35. Murakami M, Taketomi Y. Secreted Phospholipase A2 and Mast Cells. Allergol Int (2015) 64(1):4–10. doi: 10.1016/j.alit.2014.07.005
36. Fujishima H, Sanchez Mejia RO, Bingham CO 3rd, Lam BK, Sapirstein A, Bonventre JV, et al. Cytosolic Phospholipase A2 is Essential for Both the Immediate and the Delayed Phases of Eicosanoid Generation in Mouse Bone Marrow-Derived Mast Cells. Proc Natl Acad Sci USA (1999) 96(9):4803–7. doi: 10.1073/pnas.96.9.4803
37. Nakatani N, Uozumi N, Kume K, Murakami M, Kudo I, Shimizu T. Role of Cytosolic Phospholipase A2 in the Production of Lipid Mediators and Histamine Release in Mouse Bone-Marrow-Derived Mast Cells. Biochem J (2000) 352(Pt 2):311–7. doi: 10.1042/bj3520311
38. Hewson CA, Patel S, Calzetta L, Campwala H, Havard S, Luscombe E, et al. Preclinical Evaluation of an Inhibitor of Cytosolic Phospholipase A2α for the Treatment of Asthma. J Pharmacol Exp Ther (2012) 340(3):656–65. doi: 10.1124/jpet.111.186379
39. Irvin CG, Tu YP, Sheller JR, Funk CD. 5-Lipoxygenase Products are Necessary for Ovalbumin-Induced Airway Responsiveness in Mice. Am J Physiol (1997) 272(6 Pt 1):L1053–8. doi: 10.1152/ajplung.1997.272.6.L1053
40. Tager AM, Bromley SK, Medoff BD, Islam SA, Bercury SD, Friedrich EB, et al. Leukotriene B4 Receptor BLT1 Mediates Early Effector T Cell Recruitment. Nat Immunol (2003) 4(10):982–90. doi: 10.1038/ni970
41. Kim DC, Hsu FI, Barrett NA, Friend DS, Grenningloh R, Ho IC, et al. Cysteinyl Leukotrienes Regulate Th2 Cell-Dependent Pulmonary Inflammation. J Immunol (2006) 176(7):4440–8. doi: 10.4049/jimmunol.176.7.4440
42. Barrett NA, Rahman OM, Fernandez JM, Parsons MW, Xing W, Austen KF, et al. Dectin-2 Mediates Th2 Immunity Through the Generation of Cysteinyl Leukotrienes. J Exp Med (2011) 208(3):593–604. doi: 10.1084/jem.20100793
43. Matsuoka T, Hirata M, Tanaka H, Takahashi Y, Murata T, Kabashima K, et al. Prostaglandin D2 as a Mediator of Allergic Asthma. Science (2000) 287(5460):2013–7. doi: 10.1126/science.287.5460.2013
44. Shiraishi Y, Asano K, Niimi K, Fukunaga K, Wakaki M, Kagyo J, et al. Cyclooxygenase-2/Prostaglandin D2/CRTH2 Pathway Mediates Double-Stranded RNA-Induced Enhancement of Allergic Airway Inflammation. J Immunol (2008) 180(1):541–9. doi: 10.4049/jimmunol.180.1.541
45. Ishii S, Nagase T, Shindou H, Takizawa H, Ouchi Y, Shimizu T. Platelet-Activating Factor Receptor Develops Airway Hyperresponsiveness Independently of Airway Inflammation in a Murine Asthma Model. J Immunol (2004) 172(11):7095–102. doi: 10.4049/jimmunol.172.11.7095
46. Paruchuri S, Jiang Y, Feng C, Francis SA, Plutzky J, Boyce JA. Leukotriene E4 Activates Peroxisome Proliferator-Activated Receptor γ and Induces Prostaglandin D2 Generation by Human Mast Cells. J Biol Chem (2008) 283(24):16477–87. doi: 10.1074/jbc.M705822200
47. Lazarinis N, Bood J, Gomez C, Kolmert J, Lantz AS, Gyllfors P, et al. Leukotriene E4 Induces Airflow Obstruction and Mast Cell Activation Through the Cysteinyl Leukotriene Type 1 Receptor. J Allergy Clin Immunol (2018) 142(4):1080–9. doi: 10.1016/j.jaci.2018.02.024
48. Henderson WR Jr., Tang LO, Chu SJ, Tsao SM, Chiang GK, Jones F, et al. A Role for Cysteinyl Leukotrienes in Airway Remodeling in a Mouse Asthma Model. Am J Respir Crit Care Med (2002) 165(1):108–16. doi: 10.1164/ajrccm.165.1.2105051
49. Jiang Y, Kanaoka Y, Feng C, Nocka K, Rao S, Boyce JA. Cutting Edge: Interleukin 4-Dependent Mast Cell Proliferation Requires Autocrine/Intracrine Cysteinyl Leukotriene-Induced Signaling. J Immunol (2006) 177(5):2755–9. doi: 10.4049/jimmunol.177.5.2755
50. Jiang Y, Borrelli LA, Kanaoka Y, Bacskai BJ, Boyce JA. CysLT2 Receptors Interact With CysLT1 Receptors and Down-Modulate Cysteinyl Leukotriene Dependent Mitogenic Responses of Mast Cells. Blood (2007) 110(9):3263–70. doi: 10.1182/blood-2007-07-100453
51. Doherty TA, Khorram N, Lund S, Mehta AK, Croft M, Broide DH. Lung Type 2 Innate Lymphoid Cells Express Cysteinyl Leukotriene Receptor 1, Which Regulates TH2 Cytokine Production. J Allergy Clin Immunol (2013) 132(1):205–13. doi: 10.1016/j.jaci.2013.03.048
52. Salimi M, Stoger L, Liu W, Go S, Pavord I, Klenerman P, et al. Cysteinyl Leukotriene E4 Activates Human Group 2 Innate Lymphoid Cells and Enhances the Effect of Prostaglandin D2 and Epithelial Cytokines. J Allergy Clin Immunol (2017) 140(4):1090–100.e11. doi: 10.1016/j.jaci.2016.12.958
53. von Moltke J, O'Leary CE, Barrett NA, Kanaoka Y, Austen KF, Locksley RM. Leukotrienes Provide an NFAT-Dependent Signal That Synergizes With IL-33 to Activate ILC2s. J Exp Med (2017) 214(1):27–37. doi: 10.1084/jem.20161274
54. Liu T, Barrett NA, Kanaoka Y, Yoshimoto E, Garofalo D, Cirka H, et al. Type 2 Cysteinyl Leukotriene Receptors Drive IL-33-Dependent Type 2 Immunopathology and Aspirin Sensitivity. J Immunol (2018) 200(3):915–27. doi: 10.4049/jimmunol.1700603
55. Bankova LG, Dwyer DF, Yoshimoto E, Ualiyeva S, McGinty JW, Raff H, et al. The Cysteinyl Leukotriene 3 Receptor Regulates Expansion of IL-25-Producing Airway Brush Cells Leading to Type 2 Inflammation. Sci Immunol (2018) 3(28):eaat9453. doi: 10.1126/sciimmunol.aat9453
56. McGinty JW, Ting HA, Billipp TE, Nadjsombati MS, Khan DM, Barrett NA, et al. Tuft-Cell-Derived Leukotrienes Drive Rapid Anti-Helminth Immunity in the Small Intestine But are Dispensable for Anti-Protist Immunity. Immunity (2020) 52(3):528–41.e7. doi: 10.1016/j.immuni.2020.02.005
57. Leyva-Castillo JM, Galand C, Kam C, Burton O, Gurish M, Musser MA, et al. Mechanical Skin Injury Promotes Food Anaphylaxis by Driving Intestinal Mast Cell Expansion. Immunity (2019) 50(5):1262–75.E4. doi: 10.1016/j.immuni.2019.03.023
58. Voisin T, Perner C, Messou MA, Shiers S, Ualiyeva S, Kanaoka Y, et al. The CysLT2R Receptor Mediates Leukotriene C4-Driven Acute and Chronic Itch. Proc Natl Acad Sci USA (2021) 118(13):E2022087118. doi: 10.1073/pnas.2022087118
59. Wang F, Trier AM, Li F, Kim S, Chen Z, Chai JN, et al. A Basophil-Neuronal Axis Promotes Itch. Cell (2021) 184(2):422–40.e17. doi: 10.1016/j.cell.2020.12.033
60. Hammad H, de Heer HJ, Soullie T, Hoogsteden HC, Trottein F, Lambrecht BN. Prostaglandin D2 Inhibits Airway Dendritic Cell Migration and Function in Steady State Conditions by Selective Activation of the D Prostanoid Receptor 1. J Immunol (2003) 171(8):3936–40. doi: 10.4049/jimmunol.171.8.3936
61. Yamamoto Y, Otani S, Hirai H, Nagata K, Aritake K, Urade Y, et al. Dual Functions of Prostaglandin D2 in Murine Contact Hypersensitivity via DP and CRTH2. Am J Pathol (2011) 179(1):302–14. doi: 10.1016/j.ajpath.2011.03.047
62. Nakamura T, Fujiwara Y, Yamada R, Fujii W, Hamabata T, Lee MY, et al. Mast Cell-Derived Prostaglandin D2 Attenuates Anaphylactic Reactions in Mice. J Allergy Clin Immunol (2017) 140(2):630–2.e9. doi: 10.1016/j.jaci.2017.02.030
63. Nakamura T, Maeda S, Horiguchi K, Maehara T, Aritake K, Choi BI, et al. PGD2 Deficiency Exacerbates Food Antigen-Induced Mast Cell Hyperplasia. Nat Commun (2015) 6:7514. doi: 10.1038/ncomms8514
64. Trivedi SG, Newson J, Rajakariar R, Jacques TS, Hannon R, Kanaoka Y, et al. Essential Role for Hematopoietic Prostaglandin D2 Synthase in the Control of Delayed Type Hypersensitivity. Proc Natl Acad Sci U.S.A. (2006) 103(13):5179–84. doi: 10.1073/pnas.0507175103
65. Xue L, Salimi M, Panse I, Mjosberg JM, McKenzie AN, Spits H, et al. Prostaglandin D2 Activates Group 2 Innate Lymphoid Cells Through Chemoattractant Receptor-Homologous Molecule Expressed on TH2 Cells. J Allergy Clin Immunol (2014) 133(4):1184–94. doi: 10.1016/j.jaci.2013.10.056
66. Wojno ED, Monticelli LA, Tran SV, Alenghat T, Osborne LC, Thome JJ, et al. The Prostaglandin D2 Receptor CRTH2 Regulates Accumulation of Group 2 Innate Lymphoid Cells in the Inflamed Lung. Mucosal Immunol (2015) 8(6):1313–23. doi: 10.1038/mi.2015.21
67. Xue L, Barrow A, Fleming VM, Hunter MG, Ogg G, Klenerman P, et al. Leukotriene E4 Activates Human Th2 Cells for Exaggerated Proinflammatory Cytokine Production in Response to Prostaglandin D2. J Immunol (2012) 188(2):694–702. doi: 10.4049/jimmunol.1102474
68. Xiong Z, Zhu X, Geng J, Xu Y, Wu R, Li C, et al. Intestinal Tuft-2 Cells Exert Antimicrobial Immunity via Sensing Bacterial Metabolite N-Undecanoylglycine. Immunity (2022) 55(4):686–700.e7. doi: 10.1016/j.immuni.2022.03.001
69. Kunikata T, Yamane H, Segi E, Matsuoka T, Sugimoto Y, Tanaka S, et al. Suppression of Allergic Inflammation by the Prostaglandin E Receptor Subtype EP3. Nat Immunol (2005) 6(5):524–31. doi: 10.1038/ni1188
70. Honda T, Matsuoka T, Ueta M, Kabashima K, Miyachi Y, Narumiya S. Prostaglandin E2-EP3 Signaling Suppresses Skin Inflammation in Murine Contact Hypersensitivity. J Allergy Clin Immunol (2009) 124(4):809–18.E2. doi: 10.1016/j.jaci.2009.04.029
71. Morimoto K, Shirata N, Taketomi Y, Tsuchiya S, Segi-Nishida E, Inazumi T, et al. Prostaglandin E2-EP3 Signaling Induces Inflammatory Swelling by Mast Cell Activation. J Immunol (2014) 192(3):1130–7. doi: 10.4049/jimmunol.1300290
72. Liu T, Laidlaw TM, Katz HR, Boyce JA. Prostaglandin E2 Deficiency Causes a Phenotype of Aspirin Sensitivity That Depends on Platelets and Cysteinyl Leukotrienes. Proc Natl Acad Sci USA (2013) 110(42):16987–92. doi: 10.1073/pnas.1313185110
73. Liu T, Garofalo D, Feng C, Lai J, Katz H, Laidlaw TM, et al. Platelet-Driven Leukotriene C4-Mediated Airway Inflammation in Mice is Aspirin-Sensitive and Depends on T Prostanoid Receptors. J Immunol (2015) 194(11):5061–8. doi: 10.4049/jimmunol.1402959
74. Feng C, Beller EM, Bagga S, Boyce JA. Human Mast Cells Express Multiple EP Receptors for Prostaglandin E2 That Differentially Modulate Activation Responses. Blood (2006) 107(8):3243–50. doi: 10.1182/blood-2005-07-2772
75. Safholm J, Manson ML, Bood J, Delin I, Orre AC, Bergman P, et al. Prostaglandin E2 Inhibits Mast Cell-Dependent Bronchoconstriction in Human Small Airways Through the E Prostanoid Subtype 2 Receptor. J Allergy Clin Immunol (2015) 136(5):1232–9.e1. doi: 10.1016/j.jaci.2015.04.002
76. Rastogi S, Willmes DM, Nassiri M, Babina M, Worm M. PGE2 Deficiency Predisposes to Anaphylaxis by Causing Mast Cell Hyperresponsiveness. J Allergy Clin Immunol (2020) 146(6):1387–96.e13. doi: 10.1016/j.jaci.2020.03.046
77. Lundequist A, Nallamshetty SN, Xing W, Feng C, Laidlaw TM, Uematsu S, et al. Prostaglandin E2 Exerts Homeostatic Regulation of Pulmonary Vascular Remodeling in Allergic Airway Inflammation. J Immunol (2010) 184(1):433–41. doi: 10.4049/jimmunol.0902835
78. Sturm EM, Schratl P, Schuligoi R, Konya V, Sturm GJ, Lippe IT, et al. Prostaglandin E2 Inhibits Eosinophil Trafficking Through E-Prostanoid 2 Receptors. J Immunol (2008) 181(10):7273–83. doi: 10.4049/jimmunol.181.10.7273
79. Okano M, Fujiwara T, Haruna T, Kariya S, Makihara S, Higaki T, et al. Prostaglandin E2 Suppresses Staphylococcal Enterotoxin-Induced Eosinophilia-Associated Cellular Responses Dominantly Through an E-Prostanoid 2-Mediated Pathway in Nasal Polyps. J Allergy Clin Immunol (2009) 123(4):868–74.e13. doi: 10.1016/j.jaci.2009.01.047
80. Maric J, Ravindran A, Mazzurana L, Bjorklund AK, Van Acker A, Rao A, et al. Prostaglandin E2 Suppresses Human Group 2 Innate Lymphoid Cell Function. J Allergy Clin Immunol (2018) 141(5):1761–73.e6. doi: 10.1016/j.jaci.2017.09.050
81. Okano M, Sugata Y, Fujiwara T, Matsumoto R, Nishibori M, Shimizu K, et al. E Prostanoid 2 (EP2)/EP4-Mediated Suppression of Antigen-Specific Human T-Cell Responses by Prostaglandin E2. Immunology (2006) 118(3):343–52. doi: 10.1111/j.1365-2567.2006.02376.x
82. Sawada Y, Honda T, Nakamizo S, Nakajima S, Nonomura Y, Otsuka A, et al. Prostaglandin E2 (PGE2)-EP2 Signaling Negatively Regulates Murine Atopic Dermatitis-Like Skin Inflammation by Suppressing Thymic Stromal Lymphopoietin Expression. J Allergy Clin Immunol (2019) 144(5):1265–73.e9. doi: 10.1016/j.jaci.2019.06.036
83. Hattori M, Arai H. Intracellular PAF-Acetylhydrolase Type I. Enzymes (2015) 38:23–36. doi: 10.1016/bs.enz.2015.09.007
84. Kono N, Arai H. Intracellular Platelet-Activating Factor Acetylhydrolase, Type II: A Unique Cellular Phospholipase A2 That Hydrolyzes Oxidatively Modified Phospholipids. Enzymes (2015) 38:43–54. doi: 10.1016/bs.enz.2015.09.008
85. Tjoelker LW, Wilder C, Eberhardt C, Stafforini DM, Dietsch G, Schimpf B, et al. Anti-Inflammatory Properties of a Platelet-Activating Factor Acetylhydrolase. Nature (1995) 374(6522):549–53. doi: 10.1038/374549a0
86. Oh DY, Talukdar S, Bae EJ, Imamura T, Morinaga H, Fan W, et al. GPR120 is an Omega-3 Fatty Acid Receptor Mediating Potent Anti-Inflammatory and Insulin-Sensitizing Effects. Cell (2010) 142(5):687–98. doi: 10.1016/j.cell.2010.07.041
87. Oh DY, Walenta E, Akiyama TE, Lagakos WS, Lackey D, Pessentheiner AR, et al. A Gpr120-Selective Agonist Improves Insulin Resistance and Chronic Inflammation in Obese Mice. Nat Med (2014) 20(8):942–7. doi: 10.1038/nm.3614
88. Isobe Y, Itagaki M, Ito Y, Naoe S, Kojima K, Ikeguchi M, et al. Comprehensive Analysis of the Mouse Cytochrome P450 Family Responsible for Omega-3 Epoxidation of Eicosapentaenoic Acid. Sci Rep (2018) 8(1):7954. doi: 10.1038/s41598-018-26325-4
89. Luangsay S, Wittamer V, Bondue B, De Henau O, Rouger L, Brait M, et al. Mouse ChemR23 is Expressed in Dendritic Cell Subsets and Macrophages, and Mediates an Anti-Inflammatory Activity of Chemerin in a Lung Disease Model. J Immunol (2009) 183(10):6489–99. doi: 10.4049/jimmunol.0901037
90. Krishnamoorthy S, Recchiuti A, Chiang N, Yacoubian S, Lee CH, Yang R, et al. Resolvin D1 Binds Human Phagocytes With Evidence for Proresolving Receptors. Proc Natl Acad Sci USA (2010) 107(4):1660–5. doi: 10.1073/pnas.0907342107
91. Chiang N, Dalli J, Colas RA, Serhan CN. Identification of Resolvin D2 Receptor Mediating Resolution of Infections and Organ Protection. J Exp Med (2015) 212(8):1203–17. doi: 10.1084/jem.20150225
92. Bang S, Xie YK, Zhang ZJ, Wang Z, Xu ZZ, Ji RR. GPR37 Regulates Macrophage Phagocytosis and Resolution of Inflammatory Pain. J Clin Invest (2018) 128(8):3568–82. doi: 10.1172/JCI99888
93. Chiang N, Libreros S, Norris PC, de la Rosa X, Serhan CN. Maresin 1 Activates LGR6 Receptor Promoting Phagocyte Immunoresolvent Functions. J Clin Invest (2019) 129(12):5294–311. doi: 10.1172/JCI129448
94. Basil MC, Levy BD. Specialized Pro-Resolving Mediators: Endogenous Regulators of Infection and Inflammation. Nat Rev Immunol (2016) 16(1):51–67. doi: 10.1038/nri.2015.4
95. Serhan CN, Levy BD. Resolvins in Inflammation: Emergence of the Pro-Resolving Superfamily of Mediators. J Clin Invest (2018) 128(7):2657–69. doi: 10.1172/JCI97943
96. Metz M, Piliponsky AM, Chen CC, Lammel V, Abrink M, Pejler G, et al. Mast Cells can Enhance Resistance to Snake and Honeybee Venoms. Science (2006) 313(5786):526–30. doi: 10.1126/science.1128877
97. Abraham SN, St John AL. Mast Cell-Orchestrated Immunity to Pathogens. Nat Rev Immunol (2010) 10(6):440–52. doi: 10.1038/nri2782
98. Nagatake T, Shiogama Y, Inoue A, Kikuta J, Honda T, Tiwari P, et al. The 17,18-Epoxyeicosatetraenoic Acid-G Protein-Coupled Receptor 40 Axis Ameliorates Contact Hypersensitivity by Inhibiting Neutrophil Mobility in Mice and Cynomolgus Macaques. J Allergy Clin Immunol (2018) 142(2):470–84.e12. doi: 10.1016/j.jaci.2017.09.053
99. Nakajima K, Murakami M, Yanoshita R, Samejima Y, Karasawa K, Setaka M, et al. Activated Mast Cells Release Extracellular Type Platelet-Activating Factor Acetylhydrolase That Contributes to Autocrine Inactivation of Platelet-Activating Factor. J Biol Chem (1997) 272(32):19708–13. doi: 10.1074/jbc.272.32.19708
100. Dwyer DF, Barrett NA, Austen KF, Immunological Genome Project C. Expression Profiling of Constitutive Mast Cells Reveals a Unique Identity Within the Immune System. Nat Immunol (2016) 17(7):878–87. doi: 10.1038/ni.3445
101. Lambeau G, Gelb MH. Biochemistry and Physiology of Mammalian Secreted Phospholipases A2. Annu Rev Biochem (2008) 77:495–520. doi: 10.1146/annurev.biochem.76.062405.154007
102. Murakami M, Taketomi Y, Miki Y, Sato H, Hirabayashi T, Yamamoto K. Recent Progress in Phospholipase A2 Research: From Cells to Animals to Humans. Prog Lipid Res (2011) 50(2):152–92. doi: 10.1016/j.plipres.2010.12.001
103. Murakami M, Sato H, Miki Y, Yamamoto K, Taketomi Y. A New Era of Secreted Phospholipase A2. J Lipid Res (2015) 56(7):1248–61. doi: 10.1194/jlr.R058123
104. Murakami M, Yamamoto K, Miki Y, Murase R, Sato H, Taketomi Y. The Roles of the Secreted Phospholipase A2 Gene Family in Immunology. Adv Immunol (2016) 132:91–134. doi: 10.1016/bs.ai.2016.05.001
105. Murakami M, Miki Y, Sato H, Murase R, Taketomi Y, Yamamoto K. Group IID, IIE, IIF and III Secreted Phospholipase A2s. Biochim Biophys Acta Mol Cell Biol Lipids (2019) 1864(6):803–18. doi: 10.1016/j.bbalip.2018.08.014
106. Murakami M, Kudo I, Inoue K. Eicosanoid Generation From Antigen-Primed Mast Cells by Extracellular Mammalian 14-kDa Group II Phospholipase A2. FEBS Lett (1991) 294(3):247–51. doi: 10.1016/0014-5793(91)81440-j
107. Murakami M, Kudo I, Suwa Y, Inoue K. Release of 14-kDa Group-II Phospholipase A2 From Activated Mast Cells and its Possible Involvement in the Regulation of the Degranulation Process. Eur J Biochem (1992) 209(1):257–65. doi: 10.1111/j.1432-1033.1992.tb17284.x
108. Murakami M, Hara N, Kudo I, Inoue K. Triggering of Degranulation in Mast Cells by Exogenous Type II Phospholipase A2. J Immunol (1993) 151(10):5675–84.
109. Bingham CO 3rd, Fijneman RJ, Friend DS, Goddeau RP, Rogers RA, Austen KF, et al. Low Molecular Weight Group IIA and Group V Phospholipase A2 Enzymes Have Different Intracellular Locations in Mouse Bone Marrow-Derived Mast Cells. J Biol Chem (1999) 274(44):31476–84. doi: 10.1074/jbc.274.44.31476
110. Enomoto A, Murakami M, Valentin E, Lambeau G, Gelb MH, Kudo I. Redundant and Segregated Functions of Granule-Associated Heparin-Binding Group II Subfamily of Secretory Phospholipases A2 in the Regulation of Degranulation and Prostaglandin D2 Synthesis in Mast Cells. J Immunol (2000) 165(7):4007–14. doi: 10.4049/jimmunol.165.7.4007
111. Murakami M, Koduri RS, Enomoto A, Shimbara S, Seki M, Yoshihara K, et al. Distinct Arachidonate-Releasing Functions of Mammalian Secreted Phospholipase A2s in Human Embryonic Kidney 293 and Rat Mastocytoma RBL-2H3 Cells Through Heparan Sulfate Shuttling and External Plasma Membrane Mechanisms. J Biol Chem (2001) 276(13):10083–96. doi: 10.1074/jbc.M007877200
112. Triggiani M, Giannattasio G, Calabrese C, Loffredo S, Granata F, Fiorello A, et al. Lung Mast Cells Are a Source of Secreted Phospholipases A2. J Allergy Clin Immunol (2009) 124(3):558–65, 65.e1-3. doi: 10.1016/j.jaci.2009.04.035
113. Granata F, Staiano RI, Loffredo S, Petraroli A, Genovese A, Marone G, et al. The Role of Mast Cell-Derived Secreted Phospholipases A2 in Respiratory Allergy. Biochimie (2010) 92(6):588–93. doi: 10.1016/j.biochi.2010.02.030
114. Palm NW, Rosenstein RK, Yu S, Schenten DD, Florsheim E, Medzhitov R. Bee Venom Phospholipase A2 Induces a Primary Type 2 Response That Is Dependent on the Receptor ST2 and Confers Protective Immunity. Immunity (2013) 39(5):976–85. doi: 10.1016/j.immuni.2013.10.006
115. Boudreau LH, Duchez AC, Cloutier N, Soulet D, Martin N, Bollinger J, et al. Platelets Release Mitochondria Serving as Substrate for Bactericidal Group IIA-Secreted Phospholipase A2 to Promote Inflammation. Blood (2014) 124(14):2173–83. doi: 10.1182/blood-2014-05-573543
116. Kudo K, Miki Y, Carreras J, Nakayama S, Nakamoto Y, Ito M, et al. Secreted Phospholipase A2 Modifies Extracellular Vesicles and Accelerates B Cell Lymphoma. Cell Metab (2022) 34(4):615–33.e8. doi: 10.1016/j.cmet.2022.02.011
117. Grimbaldeston MA, Nakae S, Kalesnikoff J, Tsai M, Galli SJ. Mast Cell-Derived Interleukin 10 Limits Skin Pathology in Contact Dermatitis and Chronic Irradiation With Ultraviolet B. Nat Immunol (2007) 8(10):1095–104. doi: 10.1038/ni1503
118. Hershko AY, Suzuki R, Charles N, Alvarez-Errico D, Sargent JL, Laurence A, et al. Mast Cell Interleukin-2 Production Contributes to Suppression of Chronic Allergic Dermatitis. Immunity (2011) 35(4):562–71. doi: 10.1016/j.immuni.2011.07.013
119. Dudeck A, Dudeck J, Scholten J, Petzold A, Surianarayanan S, Kohler A, et al. Mast Cells are Key Promoters of Contact Allergy That Mediate the Adjuvant Effects of Haptens. Immunity (2011) 34(6):973–84. doi: 10.1016/j.immuni.2011.03.028
120. Reber LL, Sibilano R, Starkl P, Roers A, Grimbaldeston MA, Tsai M, et al. Imaging Protective Mast Cells in Living Mice During Severe Contact Hypersensitivity. JCI Insight (2017) 2(9):e92900. doi: 10.1172/jci.insight.92900
121. Boilard E, Lai Y, Larabee K, Balestrieri B, Ghomashchi F, Fujioka D, et al. A Novel Anti-Inflammatory Role for Secretory Phospholipase A2 in Immune Complex-Mediated Arthritis. EMBO Mol Med (2010) 2(5):172–87. doi: 10.1002/emmm.201000072
122. Hamaguchi K, Kuwata H, Yoshihara K, Masuda S, Shimbara S, Oh-ishi S, et al. Induction of Distinct Sets of Secretory Phospholipase A2 in Rodents During Inflammation. Biochim Biophys Acta (2003) 1635(1):37–47. doi: 10.1016/j.bbalip.2003.10.004
123. Snider JM, You JK, Wang X, Snider AJ, Hallmark B, Zec MM, et al. Group IIA Secreted Phospholipase A2 is Associated With the Pathobiology Leading to COVID-19 Mortality. J Clin Invest (2021) 131(19):e149236. doi: 10.1172/JCI149236
124. Weinrauch Y, Abad C, Liang NS, Lowry SF, Weiss J. Mobilization of Potent Plasma Bactericidal Activity During Systemic Bacterial Challenge. Role of Group IIA Phospholipase A2. J Clin Invest (1998) 102(3):633–8. doi: 10.1172/JCI3121
125. Murakami M, Kudo I, Umeda M, Matsuzawa A, Takeda M, Komada M, et al. Detection of Three Distinct Phospholipases A2 in Cultured Mast Cells. J Biochem (1992) 111(2):175–81. doi: 10.1093/oxfordjournals.jbchem.a123733
126. Chock SP, Schmauder-Chock EA, Cordella-Miele E, Miele L, Mukherjee AB. The Localization of Phospholipase A2 in the Secretory Granule. Biochem J (1994) 300( Pt 3):619–22. doi: 10.1042/bj3000619
127. Kennedy BP, Payette P, Mudgett J, Vadas P, Pruzanski W, Kwan M, et al. A Natural Disruption of the Secretory Group II Phospholipase A2 Gene in Inbred Mouse Strains. J Biol Chem (1995) 270(38):22378–85. doi: 10.1074/jbc.270.38.22378
128. MacPhee M, Chepenik KP, Liddell RA, Nelson KK, Siracusa LD, Buchberg AM. The Secretory Phospholipase A2 Gene is a Candidate for the Mom1 Locus, a Major Modifier of ApcMin-Induced Intestinal Neoplasia. Cell (1995) 81(6):957–66. doi: 10.1016/0092-8674(95)90015-2
129. Mulherkar R, Rao RS, Wagle AS, Patki V, Deo MG. Enhancing Factor, a Paneth Cell Specific Protein From Mouse Small Intestines: Predicted Amino Acid Sequence From RT-PCR Amplified cDNA and its Expression. Biochem Biophys Res Commun (1993) 195(3):1254–63. doi: 10.1006/bbrc.1993.2179
130. Schewe M, Franken PF, Sacchetti A, Schmitt M, Joosten R, Bottcher R, et al. Secreted Phospholipases A2 Are Intestinal Stem Cell Niche Factors With Distinct Roles in Homeostasis, Inflammation, and Cancer. Cell Stem Cell (2016) 19(1):38–51. doi: 10.1016/j.stem.2016.05.023
131. Bingham CO 3rd, Murakami M, Fujishima H, Hunt JE, Austen KF, Arm JP. A Heparin-Sensitive Phospholipase A2 and Prostaglandin Endoperoxide Synthase-2 Are Functionally Linked in the Delayed Phase of Prostaglandin D2 Generation in Mouse Bone Marrow-Derived Mast Cells. J Biol Chem (1996) 271(42):25936–44. doi: 10.1074/jbc.271.42.25936
132. Dore E, Joly-Beauparlant C, Morozumi S, Mathieu A, Levesque T, Allaeys I, et al. The Interaction of Secreted Phospholipase A2-IIA With the Microbiota Alters its Lipidome and Promotes Inflammation. JCI Insight (2022) 7(2):e152638. doi: 10.1172/jci.insight.152638
133. Duchez AC, Boudreau LH, Naika GS, Bollinger J, Belleannee C, Cloutier N, et al. Platelet Microparticles are Internalized in Neutrophils via the Concerted Activity of 12-Lipoxygenase and Secreted Phospholipase A2-IIA. Proc Natl Acad Sci USA (2015) 112(27):E3564–73. doi: 10.1073/pnas.1507905112
134. Rouault M, Le Calvez C, Boilard E, Surrel F, Singer A, Ghomashchi F, et al. Recombinant Production and Properties of Binding of the Full Set of Mouse Secreted Phospholipases A2 to the Mouse M-Type Receptor. Biochemistry (2007) 46(6):1647–62. doi: 10.1021/bi062119b
135. Reddy ST, Herschman HR. Transcellular Prostaglandin Production Following Mast Cell Activation is Mediated by Proximal Secretory Phospholipase A2 and Distal Prostaglandin Synthase 1. J Biol Chem (1996) 271(1):186–91. doi: 10.1074/jbc.271.1.186
136. Reddy ST, Herschman HR. Prostaglandin Synthase-1 and Prostaglandin Synthase-2 are Coupled to Distinct Phospholipases for the Generation of Prostaglandin D2 in Activated Mast Cells. J Biol Chem (1997) 272(6):3231–7. doi: 10.1074/jbc.272.6.3231
137. Reddy ST, Winstead MV, Tischfield JA, Herschman HR. Analysis of the Secretory Phospholipase A2 That Mediates Prostaglandin Production in Mast Cells. J Biol Chem (1997) 272(21):13591–6. doi: 10.1074/jbc.272.21.13591
138. Kikawada E, Bonventre JV, Arm JP. Group V Secretory PLA2 Regulates TLR2-Dependent Eicosanoid Generation in Mouse Mast Cells Through Amplification of ERK and cPLA2α Activation. Blood (2007) 110(2):561–7. doi: 10.1182/blood-2006-10-052258
139. Nolin JD, Lai Y, Ogden HL, Manicone AM, Murphy RC, An D, et al. Secreted PLA2 Group X Orchestrates Innate and Adaptive Immune Responses to Inhaled Allergen. JCI Insight (2017) 2(21):e94929. doi: 10.1172/jci.insight.94929
140. Munoz NM, Meliton AY, Arm JP, Bonventre JV, Cho W, Leff AR. Deletion of Secretory Group V Phospholipase A2 Attenuates Cell Migration and Airway Hyperresponsiveness in Immunosensitized Mice. J Immunol (2007) 179(7):4800–7. doi: 10.4049/jimmunol.179.7.4800
141. Giannattasio G, Fujioka D, Xing W, Katz HR, Boyce JA, Balestrieri B. Group V Secretory Phospholipase A2 Reveals its Role in House Dust Mite-Induced Allergic Pulmonary Inflammation by Regulation of Dendritic Cell Function. J Immunol (2010) 185(7):4430–8. doi: 10.4049/jimmunol.1001384
142. Ohta S, Imamura M, Xing W, Boyce JA, Balestrieri B. Group V Secretory Phospholipase A2 is Involved in Macrophage Activation and is Sufficient for Macrophage Effector Functions in Allergic Pulmonary Inflammation. J Immunol (2013) 190(12):5927–38. doi: 10.4049/jimmunol.1203202
143. Yamaguchi M, Samuchiwal SK, Quehenberger O, Boyce JA, Balestrieri B. Macrophages Regulate Lung ILC2 Activation via PlA2g5-Dependent Mechanisms. Mucosal Immunol (2018) 11(3):615–26. doi: 10.1038/mi.2017.99
144. Henderson WR Jr., Chi EY, Bollinger JG, Tien YT, Ye X, Castelli L, et al. Importance of Group X-Secreted Phospholipase A2 in Allergen-Induced Airway Inflammation and Remodeling in a Mouse Asthma Model. J Exp Med (2007) 204(4):865–77. doi: 10.1084/jem.20070029
145. Henderson WR Jr., Ye X, Lai Y, Ni Z, Bollinger JG, Tien YT, et al. Key Role of Group V Secreted Phospholipase A2 in Th2 Cytokine and Dendritic Cell-Driven Airway Hyperresponsiveness and Remodeling. PloS One (2013) 8(2):e56172. doi: 10.1371/journal.pone.0056172
146. Diaz BL, Satake Y, Kikawada E, Balestrieri B, Arm JP. Group V Secretory Phospholipase A2 Amplifies the Induction of Cyclooxygenase 2 and Delayed Prostaglandin D2 Generation in Mouse Bone Marrow Culture-Derived Mast Cells in a Strain-Dependent Manner. Biochim Biophys Acta (2006) 1761(12):1489–97. doi: 10.1016/j.bbalip.2006.09.009
147. Shi GP, Bot I, Kovanen PT. Mast Cells in Human and Experimental Cardiometabolic Diseases. Nat Rev Cardiol (2015) 12(11):643–58. doi: 10.1038/nrcardio.2015.117
148. Song WL, Stubbe J, Ricciotti E, Alamuddin N, Ibrahim S, Crichton I, et al. Niacin and Biosynthesis of PGD2 by Platelet COX-1 in Mice and Humans. J Clin Invest (2012) 122(4):1459–68. doi: 10.1172/JCI59262
149. Heller EA, Liu E, Tager AM, Sinha S, Roberts JD, Koehn SL, et al. Inhibition of Atherogenesis in BLT1-Deficient Mice Reveals a Role for LTB4 and BLT1 in Smooth Muscle Cell Recruitment. Circulation (2005) 112(4):578–86. doi: 10.1161/CIRCULATIONAHA.105.545616
150. Ii H, Hontani N, Toshida I, Oka M, Sato T, Akiba S. Group IVA Phospholipase A2-Associated Production of MMP-9 in Macrophages and Formation of Atherosclerotic Lesions. Biol Pharm Bull (2008) 31(3):363–8. doi: 10.1248/bpb.31.363
151. Meng Z, Yan C, Deng Q, Dong X, Duan ZM, Gao DF, et al. Oxidized Low-Density Lipoprotein Induces Inflammatory Responses in Cultured Human Mast Cells via Toll-Like Receptor 4. Cell Physiol Biochem (2013) 31(6):842–53. doi: 10.1159/000350102
152. Bot M, de Jager SC, MacAleese L, Lagraauw HM, van Berkel TJ, Quax PH, et al. Lysophosphatidic Acid Triggers Mast Cell-Driven Atherosclerotic Plaque Destabilization by Increasing Vascular Inflammation. J Lipid Res (2013) 54(5):1265–74. doi: 10.1194/jlr.M032862
153. Bot M, Bot I, Lopez-Vales R, van de Lest CH, Saulnier-Blache JS, Helms JB, et al. Atherosclerotic Lesion Progression Changes Lysophosphatidic Acid Homeostasis to Favor its Accumulation. Am J Pathol (2010) 176(6):3073–84. doi: 10.2353/ajpath.2010.090009
154. Hanasaki K, Yamada K, Yamamoto S, Ishimoto Y, Saiga A, Ono T, et al. Potent Modification of Low Density Lipoprotein by Group X Secretory Phospholipase A2 is Linked to Macrophage Foam Cell Formation. J Biol Chem (2002) 277(32):29116–24. doi: 10.1074/jbc.M202867200
155. Wooton-Kee CR, Boyanovsky BB, Nasser MS, de Villiers WJ, Webb NR. Group V Spla2 Hydrolysis of Low-Density Lipoprotein Results in Spontaneous Particle Aggregation and Promotes Macrophage Foam Cell Formation. Arterioscler Thromb Vasc Biol (2004) 24(4):762–7. doi: 10.1161/01.ATV.0000122363.02961.c1
156. Pruzanski W, Lambeau L, Lazdunsky M, Cho W, Kopilov J, Kuksis A. Differential Hydrolysis of Molecular Species of Lipoprotein Phosphatidylcholine by Groups IIA, V and X Secretory Phospholipases A2. Biochim Biophys Acta (2005) 1736(1):38–50. doi: 10.1016/j.bbalip.2005.07.005
157. Sato H, Kato R, Isogai Y, Saka G, Ohtsuki M, Taketomi Y, et al. Analyses of Group III Secreted Phospholipase A2 Transgenic Mice Reveal Potential Participation of This Enzyme in Plasma Lipoprotein Modification, Macrophage Foam Cell Formation, and Atherosclerosis. J Biol Chem (2008) 283(48):33483–97. doi: 10.1074/jbc.M804628200
158. Ivandic B, Castellani LW, Wang XP, Qiao JH, Mehrabian M, Navab M, et al. Role of Group II Secretory Phospholipase A2 in Atherosclerosis: 1. Increased Atherogenesis and Altered Lipoproteins in Transgenic Mice Expressing Group IIa Phospholipase A2. Arterioscler Thromb Vasc Biol (1999) 19(5):1284–90. doi: 10.1161/01.atv.19.5.1284
159. Bostrom MA, Boyanovsky BB, Jordan CT, Wadsworth MP, Taatjes DJ, de Beer FC, et al. Group V Secretory Phospholipase A2 Promotes Atherosclerosis: Evidence From Genetically Altered Mice. Arterioscler Thromb Vasc Biol (2007) 27(3):600–6. doi: 10.1161/01.ATV.0000257133.60884.44
160. Boyanovsky B, Zack M, Forrest K, Webb NR. The Capacity of Group V Spla2 to Increase Atherogenicity of ApoE-/- and LDLR-/- Mouse LDL In Vitro Predicts its Atherogenic Role In Vivo. Arterioscler Thromb Vasc Biol (2009) 29(4):532–8. doi: 10.1161/ATVBAHA.108.183038
161. Zack M, Boyanovsky BB, Shridas P, Bailey W, Forrest K, Howatt DA, et al. Group X Secretory Phospholipase A2 Augments Angiotensin II-Induced Inflammatory Responses and Abdominal Aortic Aneurysm Formation in apoE-Deficient Mice. Atherosclerosis (2011) 214(1):58–64. doi: 10.1016/j.atherosclerosis.2010.08.054
162. Ait-Oufella H, Herbin O, Lahoute C, Coatrieux C, Loyer X, Joffre J, et al. Group X Secreted Phospholipase A2 Limits the Development of Atherosclerosis in LDL Receptor-Null Mice. Arterioscler Thromb Vasc Biol (2013) 33(3):466–73. doi: 10.1161/ATVBAHA.112.300309
163. Chiba H, Michibata H, Wakimoto K, Seishima M, Kawasaki S, Okubo K, et al. Cloning of a Gene for a Novel Epithelium-Specific Cytosolic Phospholipase A2, cPLA2δ, Induced in Psoriatic Skin. J Biol Chem (2004) 279(13):12890–7. doi: 10.1074/jbc.M305801200
164. Cheung KL, Jarrett R, Subramaniam S, Salimi M, Gutowska-Owsiak D, Chen YL, et al. Psoriatic T Cells Recognize Neolipid Antigens Generated by Mast Cell Phospholipase Delivered by Exosomes and Presented by CD1a. J Exp Med (2016) 213(11):2399–412. doi: 10.1084/jem.20160258
165. Ohto T, Uozumi N, Hirabayashi T, Shimizu T. Identification of Novel Cytosolic Phospholipase A2s, Murine cPLA2δ, ϵ, and ζ, Which Form a Gene Cluster With cPLA2β. J Biol Chem (2005) 280(26):24576–83. doi: 10.1074/jbc.M413711200
166. Fensome-Green A, Stannard N, Li M, Bolsover S, Cockcroft S. Bromoenol Lactone, an Inhibitor of Group V1A Calcium-Independent Phospholipase A2 Inhibits Antigen-Stimulated Mast Cell Exocytosis Without Blocking CA2+ Influx. Cell Calcium (2007) 41(2):145–53. doi: 10.1016/j.ceca.2006.06.002
167. Zimmermann R, Strauss JG, Haemmerle G, Schoiswohl G, Birner-Gruenberger R, Riederer M, et al. Fat Mobilization in Adipose Tissue is Promoted by Adipose Triglyceride Lipase. Science (2004) 306(5700):1383–6. doi: 10.1126/science.1100747
Keywords: phospholipid, phospholipase A2, lipid mediator, mast cells, allergy, type 2 immunity
Citation: Taketomi Y and Murakami M (2022) Regulatory Roles of Phospholipase A2 Enzymes and Bioactive Lipids in Mast Cell Biology. Front. Immunol. 13:923265. doi: 10.3389/fimmu.2022.923265
Received: 19 April 2022; Accepted: 30 May 2022;
Published: 27 June 2022.
Edited by:
Frans J. Van Overveld, University College Roosevelt, NetherlandsReviewed by:
Vineesh Vimala Raveendran, King Faisal Specialist Hospital & Research Centre, Saudi ArabiaViktor Bugajev, Institute of Molecular Genetics (ASCR), Czechia
Copyright © 2022 Taketomi and Murakami. This is an open-access article distributed under the terms of the Creative Commons Attribution License (CC BY). The use, distribution or reproduction in other forums is permitted, provided the original author(s) and the copyright owner(s) are credited and that the original publication in this journal is cited, in accordance with accepted academic practice. No use, distribution or reproduction is permitted which does not comply with these terms.
*Correspondence: Makoto Murakami, makmurak@m.u-tokyo.ac.jp