- 1Department of Biology and Biotechnology Charles Darwin, Sapienza University, Rome, Italy
- 2Laboratory Affiliated to Istituto Pasteur Italia-Fondazione Cenci Bolognetti, Sapienza University, Rome, Italy
Multiple Sclerosis (MS) is a neurodegenerative autoimmune disorder of the central nervous system (CNS) characterized by the recruitment of self-reactive T lymphocytes, mainly inflammatory T helper (Th) cell subsets. Once recruited within the CNS, inflammatory Th cells produce several inflammatory cytokines and chemokines that activate resident glial cells, thus contributing to the breakdown of blood-brain barrier (BBB), demyelination and axonal loss. Astrocytes are recognized as key players of MS immunopathology, which respond to Th cell-defining cytokines by acquiring a reactive phenotype that amplify neuroinflammation into the CNS and contribute to MS progression. In this review, we summarize current knowledge of the astrocytic changes and behaviour in both MS and experimental autoimmune encephalomyelitis (EAE), and the contribution of pathogenic Th1, Th17 and Th1-like Th17 cell subsets, and CD8+ T cells to the morphological and functional modifications occurring in astrocytes and their pathological outcomes.
Introduction
Multiple sclerosis (MS) is a chronic inflammatory demyelinating disease of the central nervous system (CNS) affecting more than 2.5 million people worldwide, with a 2020 global prevalence of 35.9 per 100000 people (1). In the majority of patients, MS begins with a single clinically isolated syndrome (CIS) of neurological dysfunction that resolves over time. After the initial CIS, most patients have a second relapse and develop the relapsing-remitting MS (RRMS) form (2). Relapses are characterized by CNS inflammation and confluent area of demyelination in the white and grey matter of the brain and spinal cord caused by the loss of oligodendrocytes and myelin sheaths (3). One to two decades post-diagnosis, 15-30% of RRMS patients develop secondary progressive MS (SPMS) that is characterised by gradual neuroaxonal loss and brain atrophy, thus leading to patient disability and neurodegeneration (4). About 15% of patients develop an irreversible primary progressive form (PPMS) from the onset characterized by chronic demyelinated lesions in the white matter, axonal loss, diffuse and focal demyelination of the grey matter and neurodegeneration (5).
Despite the exact causes of MS remain still unknown, the disease is known to arise in genetically susceptible individuals (6, 7) by a complex interplay between environmental factors (8) and dysregulated immune responses (9). Several studies performed in murine models of experimental autoimmune encephalomyelitis (EAE) to explain MS pathophysiology, validated the hypothesis that MS is an autoimmune disorder characterized by the infiltration within the CNS of adaptive self-reactive immune cells, which cause demyelination and remyelination events, thus leading to the loss of sensory and motor functions (10). It is still an open question whether the initial MS triggering insult occurs within the CNS (intrinsic model), presumably affecting the oligodendrocytes and favouring the release of CNS antigens to the periphery, or whether it takes place outside the CNS (extrinsic model) leading to the activation of aberrant adaptive immune responses targeting CNS antigens (9). Independently of the place where the triggering events occur, peripheral innate and adaptive immune cells, especially autoreactive inflammatory T helper (Th) cells, cross the blood-brain barrier (BBB) and release inflammatory mediators in the brain that affect the function of resident glial cells, leading to astrogliosis, oligodendrocyte loss and axonal degeneration (11, 12).
Astrocytes are star-shaped glial cells that play a pivotal role in maintaining CNS homeostasis (13). Through highly ramified processes, astrocytes contact several cells within the CNS contributing to the formation, activity and plasticity of neuronal synapses (14), providing neurotrophic factors and metabolic support to neurons and oligodendrocytes (15–18) and ensuring the formation and maintenance of BBB integrity (19). At a resting state and in different brain regions, astrocytes are highly heterogeneous in their morphology and functional properties (20). Protoplasmic astrocytes, mainly located in the grey matter, at the hippocampus and cerebral cortex, are characterised by extremely ramified cell bodies, thus allowing them to contact synapses and perform neuromodulation. Fibrous astrocytes, mainly located in the white matter, are smaller with longer and narrower protrusions, which interact with axons at the level of the nodes of Ranvier (21). Besides these two main astrocyte subpopulations, nine more distinct astrocyte-like subtypes have been described on the basis of their morphological features including radial, marginal and perivascular glia located in the cortex of human brain (20). Moreover, the advent of single-cell RNA sequencing (scRNA-seq) and single-cell spatial transcriptomics evidenced further diversity and specialization of astrocytes depending on their differential brain localization (22–24).
In pathological conditions, such as MS, astrocytes undergo profound morphological and functional modifications (25, 26), which lead to a strong reduction of their metabolic and homeostatic functions (13, 27, 28). Moreover, astrocytes acquire a reactive phenotype characterized by the up-regulation of specific molecular markers such as glial fibrillary acidic protein (GFAP), vimentin, S100B, superoxide dismutase 1 (SOD1), complement component C3, tropomyosin receptor kinase B (TrkB) and IL-17R (29). In both MS and EAE animal models, inflammatory Th cells, once recruited to the CNS, produce cytokines such as TNF-α, IL-17, GM-CSF and IFN-γ (11) that activate astrocytes, which in turn acquire a reactive phenotype, proliferate, form glia scar (29, 30) and produce several cytokines and chemokines favouring the recruitment of leucocytes and inflammatory Th cells into the CNS parenchyma (25, 26, 31–34).
In this review, we describe the main astrocytic changes occurring in MS and the role of the crosstalk between inflammatory T cells and astrocytes in amplifying CNS inflammation and MS progression (Figure 1).
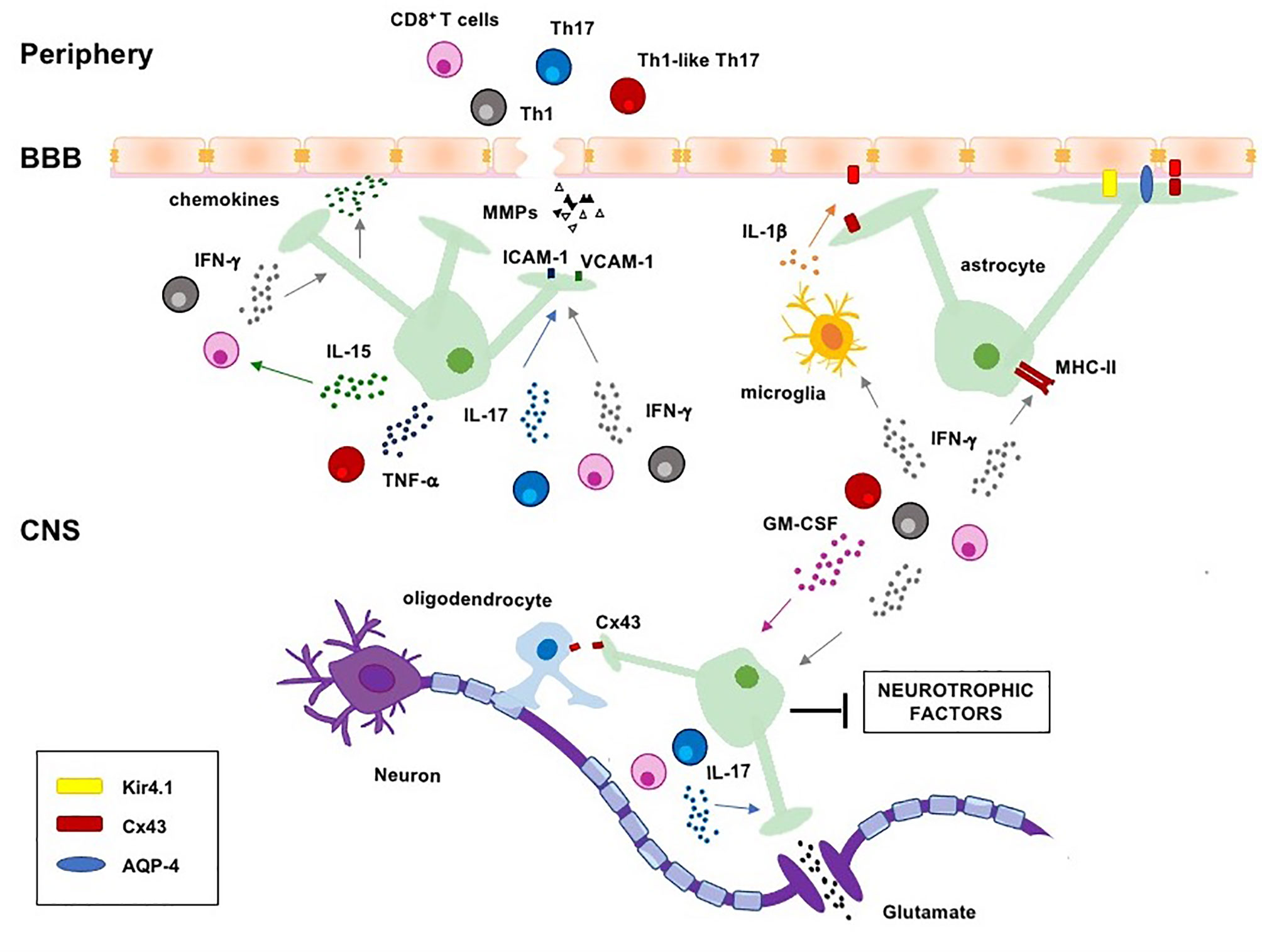
Figure 1 Regulation of astrocyte functions by pathogenic T cells and lineage-defining cytokines in MS. Pathogenic Th1, Th17, Th1-like Th17 and CD8+ T cells migrate into the CNS where they are reactivated and produce lineage-defining cytokines, which induce astrogliosis and negatively regulate several homeostatic functions of astrocytes, such as the maintenance of BBB integrity, clearance of excessive ions and glutamate from the synaptic cleft, energy support to neurons and oligodendrocytes. CNS, central nervous system; BBB, blood-brain-barrier; MHC-II, major histocompatibility complex II; ICAM-1, intercellular adhesion molecule-1; VCAM-1, vascular adhesion molecule-1; AQP-4, aquaporin 4; Cx43, connexin 43; Kir4.1, inward rectifying potassium channel 4.1.
Astrocyte Dysregulation in MS
In both MS and EAE, the activation of astrocytes occurs at an early stage and persists into the acute and chronic stages of the disease. Several changes in both morphology and spatial localization of astrocytes have been observed in different stages of the disease (28). Studies in acute EAE showed the presence of hypertrophic reactive astrocytes at a very early stage of the symptomatic phase, even before immune cells cross the BBB and enter into the CNS parenchyma (35, 36). In acute MS lesions from post-mortem brain biopsies, hypertrophic reactive astrocytes with damaged end-feet processes were detected in active plaques as well as in the adjacent normal white and grey matter, thus suggesting their pivotal role in both the development and sustainment of the lesions (28, 37, 38). Astrocytes with a very swollen cytoplasm due to the accumulation of GFAP+ filaments were also found in active-acute lesions (28). Furthermore, the phenotypic characterization of reactive astrocytes in active demyelinating MS lesions evidenced that they lose most of their homeostatic functions and acquire a highly inflammatory and neurotoxic phenotype, thus inducing the death of both neurons and mature oligodendrocytes (39, 40). As recently evidenced by magnetic resonance imaging (MRI)-informed scRNAseq, astrocytes still remain active in chronic active demyelinated lesions and form an astroglial scar as soon as chronic lesions became inactive (41). Consistently, scRNAseq analysis of CNS samples from EAE mice identified a dominant cluster of high proinflammatory and neurotoxic astrocytes, characterized by increased GM-CSF signalling, NF-κB activation and iNOS expression. The presence of this reactive astrocyte subpopulation was also confirmed in post-mortem brain tissues from MS patients who underwent euthanasia followed by rapid autopsy (42).
BBB Breakdown and Leukocyte Recruitment
The breakdown of the BBB is one crucial hallmark of MS (43, 44) that precedes the infiltration of peripheral leukocytes and autoreactive T lymphocytes that, once entered into the CNS, contribute to the development and expansion of MS lesions by damaging various cellular components of the BBB (45–50). The BBB is a continuous endothelial barrier between the CNS and peripheral blood that provides oxygen and critical nutrients to the CNS and limits the entry of toxic substances and immune cells. The integrity and functionality of the BBB are ensured by the physiological properties of highly specialized endothelial cells (EC) that, by interacting with pericytes, perivascular astrocytes and neurons, form a neurovascular unit (NVU) that limits both paracellular and transcellular movement of cells and solutes (51, 52). Perivascular astrocytes are crucial structural and functional components of the BBB that through their end-feet interact with ECs and ensheathe the brain vasculature (53). Disruption of the astroglia/NVU communication has been linked to BBB breakdown in both EAE and MS (37, 54). At the NVU, astrocytes produce several vasoactive molecules such as nitric oxide (NO), prostaglandins and arachidonic acid that regulate cerebral blood flow (55). Moreover, the specialized water-channel aquaporin-4 (AQP-4), the inward rectifying potassium channel (Kir) Kir4.1 and the gap junction-forming protein connexin 43 (Cx43) expressed by the end-feet at the level of glia limitans confer to astrocytes the ability to regulate the exchange of water and ions across the BBB (56). Extensive loss of Cx43 with concomitant patchy loss of AQP-4 was observed in actively demyelinating and chronic active lesions of progressive MS patients with high relapse rates (57), thus contributing to the weakened of BBB and to the vasogenic oedema due to increased hydrostatic vascular pressure and shear stress (Figure 1) (58).
The integrity of the BBB is also maintained by the tight junctions (TJs), large multiple transmembrane proteins containing occludins, claudins and junctional adhesion molecules (JAMs), which mediate tight adhesion between adjacent ECs. Claudins are the major components of BBB TJs and are essential for the maintenance of BBB integrity (19). The downregulation of claudin-5 and claudin-11 at the BBB has been associated with the impairment of barrier function (59, 60). In both MS and EAE lesions, reactive astrocytes upregulate thymidine phosphorylase (TYMP) and vascular endothelial growth factor A (VEGFA), which mediate the downregulation of occludin and claudin-5, thus contributing to BBB breakdown (61, 62). Furthermore, reactive astrocytes also produce CC-chemokine ligand 2 (CCL2), which contributes to the disassembly of TJs (63) and to the downregulation of both occludin and claudin-5 (64).
In addition to produce soluble factors that increase the permeability of the BBB, reactive astrocytes secrete several chemokines that favour the recruitment of circulating leukocytes into the CNS (Figure 1). CCL2 upregulation was observed in astrocytes from the white matter lesions of both MS post-mortem brains and EAE mice, where it plays a critical role in both macrophage and T cell infiltration into the white matter of spinal cord (31, 65, 66). In EAE and MS lesions, reactive astrocytes have been also identified as the major source of CCL20 (32, 33, 67), a chemokine that mediates the recruitment of pathogenic CCR6+ Th17 and Th1-like Th17 cells into the inflamed CNS (68). Furthermore, high levels of CXCL10 production by astrocytes correlated with the accumulation of CXCR3+ Th1 and Th1-like Th17 cells into the inflamed spinal cord and demyelinated lesions in EAE (25) (Figure 1).
Impaired Astrocyte-Neuron Communication in MS
In the CNS, astrocytes are closely associated with neurons, by tightly enwrapping neuronal cell bodies, axons and synapses (Figure 1). The association between astrocytes and synapses is important to maintain the brain homeostasis and to regulate neuronal synaptic transmission (69). Astrocytes regulate synaptic functions by tuning glutamate concentration in the synaptic cleft (70). Glutamate is the major excitatory neurotransmitter in CNS that, if accumulates in the synaptic and extra-synaptic space, may lead to the hyperexcitation of neurons and neuronal death through a process known as glutamate excitotoxicity (71). After release from presynaptic neurons, glutamate is taken up from post-synaptic receptors such as mGluRs (metabotropic glutamate receptors), NMDARs (N-methyl-D-aspartate receptors) and AMPARs (α-amino-3-hydroxy-5-methyl-4-isoxazolepropionic acid receptors), which in turn transmit the excitatory impulse (72). Excessive and prolonged stimulation of glutamate receptors leads to the depolarization of postsynaptic membranes and mitochondrial Ca2+ overload that triggers excessive production of reactive oxygen species (ROS) and nitric oxide (NO) and the opening of the mitochondrial transition pore, thus favouring the release of pro-apoptotic proteins and neuronal cell death (73). Astrocytes play a crucial role in removing the excess of glutamate by using specific glutamate transporters such as glutamate-aspartate transporter (GLAST) and glutamate transporter-1 (GLT-1), which uptake more than 80% glutamate released in the synaptic cleft. In astrocytes, glutamate is then metabolized by glutamine synthase (GS) into glutamine that in turn is released, taken up by neurons and used for the synthesis of glutamate and gamma-aminobutyric acid (GABA) (71). Increased levels of glutamate were observed in the CSF of RRMS patients with active lesions during relapse as well as in SPMS patients (74). Moreover, in both MS and EAE, impaired glutamate homeostasis has been related to both decreased glutamine synthetase and increased glutaminase, the enzyme responsible for glutamate synthesis, as well as to the downregulation of the glutamate transporters GLT-1 and GLAST (75–79). In addition to impaired glutamate uptake, reactive astrocytes were also described to release glutamate at the peak stage of EAE by up-regulating the glutamate carboxypeptidase II, a metalloprotease that converts the neuropeptide N-acetylaspartylglutamate into N-acetyl aspartate and glutamate (80).
Astrocyte end-feet express several channels and ion transporters, which regulate ion homeostasis and neuronal excitability. In particular, Kir4.1 K+ channel, co-expressed together with AQP4 in the astrocytes end-feet, faces neuronal synapses and allows a rapid clearance of K+ ions from the extracellular space, thus facilitating the repolarization of neuronal membranes and neuronal firing (81). Reduced levels of Kir4.1 were observed in perivascular astrocytes in both acute and chronic active demyelinated MS lesions (82). The reduction of astroglial Kir4.1 channel was associated to increased serum levels of complement-fixing IgG subclasses, suggesting a role of anti-Kir4.1 autoantibody in amplifying inflammation and tissue damage in MS (82, 83).
Another important function of astrocytes is to regulate the brain energy supply required for the transmission of synaptic impulses and neuron functions (18). Astrocytic end-feet rapidly uptake glucose from the brain capillaries and metabolize it in the glycolytic route to generate lactate. Lactate is then transferred through monocarboxylate transporters (MCT) to neurons where it is converted to pyruvate and oxidized for energy production in mitochondria (84). Interestingly, a significant reduction in the expression of genes encoding for both the astrocyte-neuro lactate shuttle (ANLS), including MCT1, and the glutamate–glutamine cycle (GGC), including glutamine synthase and GLT-1, has been observed in the grey matter from post-mortem brain tissues of chronic SPMS and PPMS patients (85). The downregulation of both ANLS and GGC genes observed in MS was also associated with the simultaneous up-regulation of inflammatory cytokines suggesting a role of immune-related signalling in the impairment of astrocyte metabolic functions (85). Consistently, Ponath et al. found that astrocytes derived from pluripotent stem cells of MS patients carrying the risk allele variant rs7665090G produced large amounts of proinflammatory factors and displayed a significant reduced ability to release lactate and reuptake glutamate after stimulation (86). The further analysis of reactive astrocytes in white matter lesions from post-mortem tissues suggested the presence of harmful hypertrophic astrocytes in MS patients carrying the risk allele variant (86). The impaired metabolic functions of astrocytes observed in MS also involve the synthesis of cholesterol that is required for myelin sheath formation, the maintenance of axonal membrane and synapses integrity (87). Astrocytes from spinal cord, cerebellum and optic nerve of chronic EAE showed a reduction in the expression of several genes involved in cholesterol synthesis. Similar results were observed by the gene expression analyses of astrocytes from optic chiasm autopsy tissues from MS patients (88).
In order to support the correct neuronal effector responses, astrocytes also secrete neurotrophic factors including nerve growth factor (NGF), brain-derived neurotropic factors (BDNF), fibroblast growth factor (FGF) and ciliary neurotrophic factor (CNTF), which are required for the optimal survival, growth and differentiation of neurons and for preventing neurodegeneration (89). Impaired production of neurotrophic factors was observed in astrocytes exposed to T cell-derived inflammatory cytokines in EAE (90). Moreover, in both EAE and chronic MS lesions, astrocytes up-regulated the BDNF receptor TrkB that upon stimulation with BDNF induced a strong release of NO, thus contributing to oxidative stress and neuronal damage (91).
Impaired Astrocyte-Oligodendrocyte Communication in MS
Reactive and hypertrophic astrocytes, accumulating in MS demyelinating lesions, also contribute to oligodendrocyte loss and demyelination by favouring lesion development and progression (38, 92). In healthy brains, astrocytes regulate the homeostasis of myelin sheaths by releasing several neurotrophic factors that promote the proliferation of oligodendrocyte progenitor cells (OPCs) their migration and differentiation to oligodendrocytes (93). The platelet-derived growth factor (PDGF) and BDNF secreted by astrocytes promote OPCs proliferation, migration and maturation to myelinating oligodendrocytes (94). During neuroinflammation, astrocytes also exert neuroprotective effects on oligodendrocytes by producing CXCL1 and CNTF, which favour OPCs recruitment to axons and their differentiation into mature myelinating oligodendrocytes, respectively (95). Consistently, reactive astrocytes in acute MS lesions secrete several remyelinating factors (96) and increased BDNF release by reactive astrocytes was found to induce remyelination in a cuprizone-induced demyelination model (97). However, with the progression of MS to a chronic stage, reactive astrocytes form a dense glial scar around the axons and secrete hyaluronan and proteoglycans, thus preventing OPCs recruitment and maturation into the demyelinated areas (97, 98). Moreover, astrocytes also supply lipids, especially cholesterol, to oligodendrocytes necessary for myelin synthesis (16, 99). This astrocyte-oligodendrocyte network is finely regulated by a physical interaction through connexins Cx30/Cx32 and Cx43/Cx47, which are fundamental for the exchange of potassium ions and metabolic factors required for myelin maintenance (16, 100, 101). A strong reduction of Cx47 in both cell bodies and proximal oligodendrocyte processes as well as of the astrocyte binding partner Cx43 was observed in EAE lesions. A concomitant loss of Cx32 was also detected within and around the lesions that persisted throughout the disease course (102). Similar results were obtained by immunohistochemical analysis of post-mortem brain tissues from MS patients, where a strong reduction of both oligodendrocyte Cx32 and Cx47 was observed in and around chronic lesions as well as in the normal-appearing white matter (NAWM) (103). On the contrary, the expression of Cx30 and Cx43 on astrocytes was increased in both lesions and NAWM and correlated with astrogliosis and the acquisition by astrocytes of an inflammatory phenotype, while higher Cx32 expression was associated with a longer disease duration (104). So, the loss of connection between oligodendrocytes and reactive astrocytes during chronic inflammation may accelerate MS progression by contributing to demyelination and axonal damage. Consistently, Cx43 loss was associated with a rapidly progressive MS course, oligodendrogliopathy and active demyelinating lesions (57).
Dysregulation of Astrocyte Functions by Inflammatory T Cells in MS
The immunopathogenesis of MS relies on the recruitment of specific autoreactive Th cell subsets and CD8+ T cells within CNS where they are reactivated and secrete cytokines and chemokines that modulate the activity of several glial cells, including astrocytes (11, 105). Among Th cells, Th1, Th17 and Th1-like Th17 cells have been identified as key players of MS pathogenesis, by producing one or more lineage-defining cytokines, which affect several astrocyte functions as discussed below (Figure 1).
Th1-Mediated Regulation of Astrocytes in MS
Th1 cells are a subset of CD4+ T lymphocytes characterized by the expression of the CXC chemokine receptor type 3 (CXCR3), interleukin (IL)-12 receptor (IL-12R) chains β1/β2, the master transcription factor T-bet and by the production of the lineage-signature cytokine IFN-γ together with GM-CSF and TNF-α (106). The neuropathological functions of Th1 cells in MS have been extensively studied in EAE animal models (11) and associated to their ability to trigger the activation of resident microglia and their differentiation into a high inflammatory and neurotoxic phenotype (107, 108). More recent studies evidenced that Th1 cells and their effector cytokines may also affect the phenotype and functions of astrocytes in MS (109). Human astrocytes, indeed, were found to express IFN-γ receptor (IFNGR) that was also up-regulated in the cortex of post-mortem MS brain tissues and associated with the acquisition of a neurotoxic phenotype (110). Silencing of IFN-γ signalling in murine astrocytes, suppressed EAE by inhibiting inflammatory chemokine production and the infiltration of Th1 and Th17 cells into the CNS (111, 112). Moreover, IFN-γ-treated astrocytes up-regulated the expression of chemokines such as CCL20, CXCL10 and CXCL12 involved in the recruitment of both Th1 and Th17 cells into the CNS, and CCL2 (90, 113) that contributes to BBB breakdown by inducing both disassembly and downregulation of TJs (63, 64). In addition to favour the infiltration of inflammatory T cells within CNS, IFN-γ-activated astrocytes were also described to promote the proliferation of myelin-specific T cells during EAE by up-regulating major histocompatibility complex class II (MHC-II) molecules and contributing to the reactivation of pathogenic T lymphocytes as antigen-presenting cells (APC) (114). Interestingly, astrocytes in chronic active lesions from post-mortem MS brain tissues were found to express MHC-II together with B7.1 and B7.2 (115, 116), two important costimulatory molecules that are required for optimal APC functions and up-regulated by IFN-γ (117, 118). However, IFN-γ was also described to mediate protective effects on astrocytes during chronic EAE. Smith et al. observed an exacerbation of chronic EAE, an increase of the lesion size and enhanced oxidative stress, in mice with IFNGR-deficient astrocytes (119). Similar results were obtained in EAE transgenic mice expressing a signalling deficient dominant negative IFNGR1 on astrocytes (120).
Th1-derived cytokines have been also implicated in polarizing astrocytes to a neurotoxic phenotype. In astrocytes from EAE mice, Th1-derived cytokines such as IFN-γ and GM-CSF impaired the expression of neurotrophic factors such as NGF, CNTF and BDNF, and up-regulated the expression of NO synthase (90). Moreover, the loss of Cx43 in astrocytes from acute demyelinating MS lesions has been recently associated to Th1-derived IFN-γ via microglia-dependent production of IL-1β, thus contributing to the disruption of astrocyte intercellular communications and MS progression (121).
Th17-Mediated Regulation of Astrocytes in MS
Th17 cells are characterized by the expression of CCR6, CCR4, CD161, IL-23R, IL-1R, the master transcription factor retinoic acid receptor-related orphan nuclear receptor γt (RORγt) and the production of the lineage-signature cytokines IL-17A-F and IL-21 together with IL-22 (122). The pathogenic functions of Th17 cells in MS have been associated to BBB breakdown and CNS inflammation (123, 124) by targeting both resident microglia and astrocytes (90, 107). Astrocytes, indeed, express a functional IL-17R and are responsive to IL-17 by polarizing towards a reactive phenotype and by producing several inflammatory cytokines and chemokines during EAE (67, 125–127). The impairment of IL-17-mediated signalling in astrocytes through the selective ablation of key signalling mediators was shown to ameliorate EAE by inhibiting the production of inflammatory chemokines, the infiltration of inflammatory cells (128, 129) and the percentage of Th17 cells within CNS (130). For instance, in astrocytes, IL-17 induces the expression and production of IL-6 that, by acting in a positive feedback loop, may amplify Th17 cell differentiation (131–133). IL-17 also enhances the production of CCL20 in astrocytes (67, 128), thus facilitating the recruitment of Th17 cells within CNS (90). Moreover, by up-regulating the expression of vascular adhesion molecule-1 (VCAM-1) on brain stem astrocytes, Th17-associated cytokines may further enhance the recruitment of both Th1 and Th17 cells within the CNS (134).
IL-17-mediated signalling in astrocytes also promotes the secretion of matrix metalloproteinases such as MMP-3 and MMP-9 that further compromise the integrity of the BBB favouring the recruitment of encephalitogenic T cells into the CNS (129, 130).
Th17 cell-associated cytokines were also shown to affect the homeostatic functions of astrocytes. Kostic et al. reported that low doses of IL-17A impaired the ability of astrocytes to uptake glutamate from the extracellular space by reducing the expression of GLT-1 and GLAST transporters as well as of glutamine synthetase. In addition to reduce glutamate uptake, exposure of astrocytes to IL-17A also caused a Ca2+-dependent glutamate release, thus favouring excitotoxic damage (Figure 1) (135).
Role of Th1-Like Th17 Cells on Astrocyte Functions in MS
Despite most of the studies carried out to investigate the crosstalk between inflammatory Th cells and astrocytes focused on Th1 and Th17 cells, the recent identification of highly pathogenic Th1-like Th17 cells in both EAE (49, 136) and MS (136–140) suggests their contribution in promoting the morphological and functional changes occurring during astrogliosis. Th1-like Th17 produce TNF-α, GM-CSF, IL-17A, although at lower levels than Th17 cells, high levels of IFN-γ, co-express CXCR3 and T-bet together with CCR6 and RORγt, and express IL-23R (122, 141, 142).
Most of the cytokines produced by Th1-like Th17 cells may exhibit synergistic detrimental effects on astrocytes. For instance, IFN-γ and TNF-α cooperate with IL-17A by inducing the production of inflammatory chemokines in astrocytes and enhancing the recruitment of encephalitogenic T cells into the CNS (90, 128, 132, 143). Moreover, IFN-γ and IL-17A produced by Th1-like Th17 cells induce the up-regulation of intercellular adhesion molecule-1 (ICAM-1) and VCAM-1 in cortical astrocytes within CNS lesions during EAE (144). Finally, a detrimental contribution of Th1-like Th17 cells on astrocytes is strongly supported by the above cited effects of IFN-γ in combination with GM-CSF. Indeed, these cytokines cooperate in impairing the production of neurotrophic factors as well as in promoting oxidative stress in astrocytes (90) and enhance astrocyte-dependent glutamate excitotoxicity induced by IL-17A (Figure 1) (135). Nevertheless, further studies are required to elucidate this issue.
Crosstalk Between CD8+ T Cells and Astrocytes in MS
Although inflammatory Th cell subsets have long been regarded as the main effectors of MS pathogenesis, an important pathophysiological role of CD8+ T cells has also recently been recognized. Histopathological studies of immune cell infiltrates in post-mortem brain tissues from MS patients showed a prevalence of CD8+ T cells compared to CD4+ T cells (105, 145). Moreover, sRNA-seq analyses revealed a prominent oligoclonal expansion of CD8+ T cells in the peripheral blood and CSF of MS patients (146, 147).
Activated CD8+ T cells may contribute to BBB breakdown by inducing the activation of astrocytes and the downregulation of both occludin and claudin-5 in perforin-dependent and non-apoptotic manner (148). Once entered into the CNS, CD8+ T cells may be reactivated by MHC class I-expressing resident glial cells, including reactive astrocytes (145), thus contributing to tissue damage and neuroinflammation (149). In EAE, CD8+ T cells also produce IL-17 thus supporting Th17-mediated dysregulation of astrocyte functions (150). Astrocytes in turn may enhance the cytotoxic activity of CNS-infiltrating CD8+ T cells by producing IL-15 (151). Immunohistochemistry analysis of post-mortem brain tissues from MS patients revealed the expression of IL-15 in reactive GFAP+ astrocytes located in both acute and subacute MS lesions as well as near blood vessels. The exposure of CD8+ T cells to astrocyte-derived IL-15 enhanced antigen-specific cytotoxicity as well as the expression of lytic enzymes (granzyme B and perforin) and natural killer group 2 member D (NKG2D) (151). NKG2D is a transmembrane receptor constitutively expressed on human CD8+ T cells that recognizes stress-induced ligands on target cells and enhances TCR-mediated cytotoxicity (152). Notably, ULBP4, a NKG2D ligand, was highly expressed on astrocytes in active and chronic active MS lesions from post-mortem brain tissues. Furthermore, the addition of soluble ULPB4 to CD8+ T cells from MS patients co-cultured with astrocytes enhanced the production of inflammatory cytokines and increased T cell motility, thus suggesting an important contribution of the crosstalk between CD8+ T cells and astrocytes in synergy with CD4+ Th cells to CNS damage in MS (153).
Impact of T Cell Targeting MS Therapeutic Drugs on Astrocytes
The crosstalk between inflammatory T cells and astrocytes in sustaining neuroinflammation and neurodegeneration in MS is supported by recent evidences that some of the disease-modifying drugs target both inflammatory T cells (11) and astrocytes (154).
Glatiramer acetate (GA), an acetate salts composed of a mixture of four synthetic polypeptides (glutamate, lysine, alanine, and tyrosine), suppresses inflammatory Th1 cells by promoting their shift to an anti-inflammatory Th2 cell phenotype (155–157) and by increasing the frequency of suppressive regulatory T cells (Treg) (158). In EAE mice, GA-specific Th2 cells produced high levels of BDNF, IL-10 and TGF-β following their adoptive transfer within CNS (159). Moreover, the production of immunosuppressive cytokines and neurotrophic factors by GA-specific T cells primed astrocytes to produce IL-10 and TGF-β (159), thus promoting their transition from a neurotoxic to a neuroprotective phenotype (160). More recent data from Eilam et al. also evidenced that GA treatment of EAE mice partially abrogated BBB disruption by increasing the expression of claudin-5 on astrocytes and by restoring their end-feet connections with the NVU (54).
Dimethyl fumarate (DMF) acts on T cells by reducing the total number of circulating T cells (161, 162), in particular of IFN-γ- and IL-17-producing Th cell subsets and CD8+ T cells (163, 164), and by increasing the percentage of Th2 and Treg cells (165, 166). DMF treatment of primary reactive astrocytes derived from both human and murine brains evidenced the ability of this drug to reduce the secretion of inflammatory cytokines and chemokines as well as to prevent the production of ROS (167) by promoting anti-oxidant gene expression (168). Similar effects were observed by treating human astrocytes with a novel fumarate, isosorbide-DMF (IDMF). Genome-wide expression analysis of human astrocytes treated with DMF and IDMF evidenced the ability of both compounds to downregulate the expression of several genes associated with neurotoxic reactive astrocytes, including MMP9, CCL2 and ICAM1 (169).
Fingolimod (FTY720) and siponimod (BAF312) are two antagonists of the sphingosine-1-phosphate receptor (S1PR) approved for RRMS and SPMS, respectively (11, 170). Both drugs bind S1PR and induce its internalization, thus sequestering T cells in lymphoid organs and reducing circulating inflammatory CD4+ and CD8+ T cells (11, 170–172). In both human and murine astrocytes, fingolimod treatment was shown to impair the production of inflammatory and neurotoxic factors (173) and to promote the secretion of neurotrophic mediators (174). Moreover, Trkov Bobnar et al. showed that fingolimod reduced the expression of MHC-II on the surface of IFN-γ-treated astrocytes, thus preventing their activity as APCs (175). As fingolimod, siponimod was reported to impair inflammatory cytokine expression in human astrocytes and to restore astrocyte-endothelial cell connections by up-regulating the expression of claudin-5 and ZO-1 (176, 177). Finally, in human astrocytes, both fingolimod and siponimod prevented glutamate neurotoxicity by restoring the expression of GLAST and GLT1 on astrocytes and glutamate uptake (177, 178).
Laquinimod (LQ) is a quinoline-3-carboxamide derivate under clinical trial evaluation for the treatment of RRMS (179). In EAE, LQ treatment ameliorated disease progression by reducing the polarization and recruitment of Th17 cells as well as the production of inflammatory cytokines (180, 181). Treatment of human astrocytes with LQ inhibited IL-1β-induced downregulation of glutamate transporters GLAST and GLT1 and restored astrocyte glutamate uptake (178). Consistently, LQ treatment ameliorated EAE by suppressing, in astrocytes, the expression of inflammatory mediators such as IL-6 and ROS and by inducing a transcriptional program associated to homeostatic chemokines, neurotrophin, axonal guidance and transendothelial migration (182).
Conclusions
The contribution of Th1, Th17, Th1-like Th17 inflammatory cytokines in activating astrocytes to gain a neurotoxic phenotype is beginning to be studied in MS. However, astrocytes may also counteract inflammation by producing several factors, which reduce BBB breakdown, leucocyte infiltration and promote remyelination, axonal regeneration and neurogenesis (21). Therefore, therapeutic strategies aimed at counteracting the infiltration of inflammatory Th cell subsets into the CNS and at polarizing reactive astrocytes towards a neuroprotective phenotype may be beneficial to arrest disease progression as well as to stimulate repair processes. A deeper understanding of the cross-talk between T cells and astrocytes in MS will be seminal for the development of more efficient therapies dampening inflammatory T cells and stimulating neuroprotective astrocytes.
Author Contributions
MK, CA, and VT wrote the original draft. MF contributed to reviewing and editing the manuscript. LT contributed to writing and editing the manuscript. All authors contributed to the article and approved the submitted version.
Funding
This work was supported by the Italian Foundation for Multiple Sclerosis (FISM 2020-R-Single/01), “Progetto Ateneo” (Sapienza University of Rome, Italy) and Istituto Pasteur Italia-Fondazione Cenci Bolognetti (Sapienza University of Rome, Italy) to LT. Fondazione Ceschina (Lugano, Switzerland) to MF.
Conflict of Interest
The authors declare that the research was conducted in the absence of any commercial or financial relationships that could be construed as a potential conflict of interest.
The handling editor declared a past co-authorship with the authors MK, CA, and LT.
Publisher’s Note
All claims expressed in this article are solely those of the authors and do not necessarily represent those of their affiliated organizations, or those of the publisher, the editors and the reviewers. Any product that may be evaluated in this article, or claim that may be made by its manufacturer, is not guaranteed or endorsed by the publisher.
References
1. Walton C, King R, Rechtman L, Kaye W, Leray E, Marrie RA, et al. Rising Prevalence of Multiple Sclerosis Worldwide: Insights From the Atlas of MS, Third Edition. Mult Scler (2020) 26:1816–21. doi: 10.1177/1352458520970841
2. Thompson AJ, Banwell BL, Barkhof F, Carroll WM, Coetzee T, Comi G, et al. Diagnosis of Multiple Sclerosis: 2017 Revisions of the McDonald Criteria. Lancet Neurol (2018) 17:162–73. doi: 10.1016/S1474-4422(17)30470-2
3. Popescu BF, Lucchinetti CF. Pathology of Demyelinating Diseases. Annu Rev Pathol (2012) 7:185–217. doi: 10.1146/annurev-pathol-011811-132443
4. Lorscheider J, Buzzard K, Jokubaitis V, Spelman T, Havrdova E, Horakova D, et al. Defining Secondary Progressive Multiple Sclerosis. Brain (2016) 139:2395–405. doi: 10.1093/brain/aww173
5. Correale J, Gaitan MI, Ysrraelit MC, Fiol MP. Progressive Multiple Sclerosis: From Pathogenic Mechanisms to Treatment. Brain (2017) 140:527–46. doi: 10.1093/brain/aww258
6. International Multiple Sclerosis Genetics, Beecham AH, Patsopoulos NA, Xifara DK, Davis MF, Kemppinen A, et al. Analysis of Immune-Related Loci Identifies 48 New Susceptibility Variants for Multiple Sclerosis. Nat Genet (2013) 45:1353–60. doi: 10.1038/ng.2770
7. Cotsapas C, Mitrovic M. Genome-Wide Association Studies of Multiple Sclerosis. Clin Transl Immunol (2018) 7:e1018. doi: 10.1002/cti2.1018
8. Belbasis L, Bellou V, Evangelou E, Ioannidis JP, Tzoulaki I. Environmental Risk Factors and Multiple Sclerosis: An Umbrella Review of Systematic Reviews and Meta-Analyses. Lancet Neurol (2015) 14:263–73. doi: 10.1016/S1474-4422(14)70267-4
9. Dendrou CA, Fugger L, Friese MA. Immunopathology of Multiple Sclerosis. Nat Rev Immunol (2015) 15:545–58. doi: 10.1038/nri3871
10. Thompson AJ, Baranzini SE, Geurts J, Hemmer B, Ciccarelli O. Multiple Sclerosis. Lancet (2018) 391:1622–36. doi: 10.1016/S0140-6736(18)30481-1
11. Kunkl M, Frascolla S, Amormino C, Volpe E, Tuosto L. T Helper Cells: The Modulators of Inflammation in Multiple Sclerosis. Cells (2020) 9:482–502. doi: 10.3390/cells9020482
12. Hemmer B, Kerschensteiner M, Korn T. Role of the Innate and Adaptive Immune Responses in the Course of Multiple Sclerosis. Lancet Neurol (2015) 14:406–19. doi: 10.1016/S1474-4422(14)70305-9
13. Brambilla R. The Contribution of Astrocytes to the Neuroinflammatory Response in Multiple Sclerosis and Experimental Autoimmune Encephalomyelitis. Acta Neuropathol (2019) 137:757–83. doi: 10.1007/s00401-019-01980-7
14. Allen NJ, Eroglu C. Cell Biology of Astrocyte-Synapse Interactions. Neuron (2017) 96:697–708. doi: 10.1016/j.neuron.2017.09.056
15. Orthmann-Murphy JL, Abrams CK, Scherer SS. Gap Junctions Couple Astrocytes and Oligodendrocytes. J Mol Neurosci (2008) 35:101–16. doi: 10.1007/s12031-007-9027-5
16. Camargo N, Goudriaan A, van Deijk AF, Otte WM, Brouwers JF, Lodder H, et al. Oligodendroglial Myelination Requires Astrocyte-Derived Lipids. PloS Biol (2017) 15:e1002605. doi: 10.1371/journal.pbio.1002605
17. Nortley R, Attwell D. Control of Brain Energy Supply by Astrocytes. Curr Opin Neurobiol (2017) 47:80–5. doi: 10.1016/j.conb.2017.09.012
18. Deitmer JW, Theparambil SM, Ruminot I, Noor SI, Becker HM. Energy Dynamics in the Brain: Contributions of Astrocytes to Metabolism and pH Homeostasis. Front Neurosci (2019) 13:1301. doi: 10.3389/fnins.2019.01301
19. Castro Dias M, Mapunda JA, Vladymyrov M, Engelhardt B. Structure and Junctional Complexes of Endothelial, Epithelial and Glial Brain Barriers. Int J Mol Sci (2019) 20:5372–99. doi: 10.3390/ijms20215372
20. Matyash V, Kettenmann H. Heterogeneity in Astrocyte Morphology and Physiology. Brain Res Rev (2010) 63:2–10. doi: 10.1016/j.brainresrev.2009.12.001
21. Kohler S, Winkler U, Hirrlinger J. Heterogeneity of Astrocytes in Grey and White Matter. Neurochem Res (2021) 46:3–14. doi: 10.1007/s11064-019-02926-x
22. Lo CH, Skarica M, Mansoor M, Bhandarkar S, Toro S, Pitt D. Astrocyte Heterogeneity in Multiple Sclerosis: Current Understanding and Technical Challenges. Front Cell Neurosci (2021) 15:726479. doi: 10.3389/fncel.2021.726479
23. Bayraktar OA, Bartels T, Holmqvist S, Kleshchevnikov V, Martirosyan A, Polioudakis D, et al. Astrocyte Layers in the Mammalian Cerebral Cortex Revealed by a Single-Cell in Situ Transcriptomic Map. Nat Neurosci (2020) 23:500–9. doi: 10.1038/s41593-020-0602-1
24. Batiuk MY, Martirosyan A, Wahis J, de Vin F, Marneffe C, Kusserow C, et al. Identification of Region-Specific Astrocyte Subtypes at Single Cell Resolution. Nat Commun (2020) 11:1220. doi: 10.1038/s41467-019-14198-8
25. Mills Ko E, Ma JH, Guo F, Miers L, Lee E, Bannerman P, et al. Deletion of Astroglial CXCL10 Delays Clinical Onset But Does Not Affect Progressive Axon Loss in a Murine Autoimmune Multiple Sclerosis Model. J Neuroinflamm (2014) 11:105. doi: 10.1186/1742-2094-11-105
26. Choi SS, Lee HJ, Lim I, Satoh J, Kim SU. Human Astrocytes: Secretome Profiles of Cytokines and Chemokines. PloS One (2014) 9:e92325. doi: 10.1371/journal.pone.0092325
27. Aharoni R, Eilam R, Arnon R. Astrocytes in Multiple Sclerosis-Essential Constituents With Diverse Multifaceted Functions. Int J Mol Sci (2021) 22:5904–25. doi: 10.3390/ijms22115904
28. Ludwin SK, Rao V, Moore CS, Antel JP. Astrocytes in Multiple Sclerosis. Mult Scler (2016) 22:1114–24. doi: 10.1177/1352458516643396
29. Escartin C, Galea E, Lakatos A, O'Callaghan JP, Petzold GC, Serrano-Pozo A, et al. Reactive Astrocyte Nomenclature, Definitions, and Future Directions. Nat Neurosci (2021) 24:312–25. doi: 10.1038/s41593-020-00783-4
30. Sofroniew MV. Multiple Roles for Astrocytes as Effectors of Cytokines and Inflammatory Mediators. Neuroscientist (2014) 20:160–72. doi: 10.1177/1073858413504466
31. Kim RY, Hoffman AS, Itoh N, Ao Y, Spence R, Sofroniew MV, et al. Astrocyte CCL2 Sustains Immune Cell Infiltration in Chronic Experimental Autoimmune Encephalomyelitis. J Neuroimmunol (2014) 274:53–61. doi: 10.1016/j.jneuroim.2014.06.009
32. Ambrosini E, Remoli ME, Giacomini E, Rosicarelli B, Serafini B, Lande R, et al. Astrocytes Produce Dendritic Cell-Attracting Chemokines In Vitro and in Multiple Sclerosis Lesions. J Neuropathol Exp Neurol (2005) 64:706–15. doi: 10.1097/01.jnen.0000173893.01929.fc
33. Ambrosini E, Columba-Cabezas S, Serafini B, Muscella A, Aloisi F. Astrocytes Are the Major Intracerebral Source of Macrophage Inflammatory Protein-3alpha/CCL20 in Relapsing Experimental Autoimmune Encephalomyelitis and In Vitro. Glia (2003) 41:290–300. doi: 10.1002/glia.10193
34. Sorensen TL, Tani M, Jensen J, Pierce V, Lucchinetti C, Folcik VA, et al. Expression of Specific Chemokines and Chemokine Receptors in the Central Nervous System of Multiple Sclerosis Patients. J Clin Invest (1999) 103:807–15. doi: 10.1172/JCI5150
35. Chanaday NL, Roth GA. Microglia and Astrocyte Activation in the Frontal Cortex of Rats With Experimental Autoimmune Encephalomyelitis. Neuroscience (2016) 314:160–9. doi: 10.1016/j.neuroscience.2015.11.060
36. Grygorowicz T, Welniak-Kaminska M, Struzynska L. Early P2X7R-Related Astrogliosis in Autoimmune Encephalomyelitis. Mol Cell Neurosci (2016) 74:1–9. doi: 10.1016/j.mcn.2016.02.003
37. Brosnan CF, Raine CS. The Astrocyte in Multiple Sclerosis Revisited. Glia (2013) 61:453–65. doi: 10.1002/glia.22443
38. Ponath G, Ramanan S, Mubarak M, Housley W, Lee S, Sahinkaya FR, et al. Myelin Phagocytosis by Astrocytes After Myelin Damage Promotes Lesion Pathology. Brain (2017) 140:399–413. doi: 10.1093/brain/aww298
39. Liddelow SA, Guttenplan KA, Clarke LE, Bennett FC, Bohlen CJ, Schirmer L, et al. Neurotoxic Reactive Astrocytes Are Induced by Activated Microglia. Nature (2017) 541:481–7. doi: 10.1038/nature21029
40. Liddelow SA, Barres BA. Reactive Astrocytes: Production, Function, and Therapeutic Potential. Immunity (2017) 46:957–67. doi: 10.1016/j.immuni.2017.06.006
41. Absinta M, Maric D, Gharagozloo M, Garton T, Smith MD, Jin J, et al. A Lymphocyte-Microglia-Astrocyte Axis in Chronic Active Multiple Sclerosis. Nature (2021) 597:709–14. doi: 10.1038/s41586-021-03892-7
42. Wheeler MA, Clark IC, Tjon EC, Li Z, Zandee SEJ, Couturier CP, et al. MAFG-Driven Astrocytes Promote CNS Inflammation. Nature (2020) 578:593–9. doi: 10.1038/s41586-020-1999-0
43. Ortiz GG, Pacheco-Moises FP, Macias-Islas MA, Flores-Alvarado LJ, Mireles-Ramirez MA, Gonzalez-Renovato ED, et al. Role of the Blood-Brain Barrier in Multiple Sclerosis. Arch Med Res (2014) 45:687–97. doi: 10.1016/j.arcmed.2014.11.013
44. Alvarez JI, Cayrol R, Prat A. Disruption of Central Nervous System Barriers in Multiple Sclerosis. Biochim Biophys Acta (2011) 1812:252–64. doi: 10.1016/j.bbadis.2010.06.017
45. Sonar SA, Lal G. Differentiation and Transmigration of CD4 T Cells in Neuroinflammation and Autoimmunity. Front Immunol (2017) 8:1695. doi: 10.3389/fimmu.2017.01695
46. Setiadi AF, Abbas AR, Jeet S, Wong K, Bischof A, Peng I, et al. IL-17A Is Associated With the Breakdown of the Blood-Brain Barrier in Relapsing-Remitting Multiple Sclerosis. J Neuroimmunol (2019) 332:147–54. doi: 10.1016/j.jneuroim.2019.04.011
47. Kim HJ, Biernacki K, Prat A, Antel JP, Bar-Or A. Inflammatory Potential and Migratory Capacities Across Human Brain Endothelial Cells of Distinct Glatiramer Acetate-Reactive T Cells Generated in Treated Multiple Sclerosis Patients. Clin Immunol (2004) 111:38–46. doi: 10.1016/j.clim.2004.01.004
48. Kebir H, Kreymborg K, Ifergan I, Dodelet-Devillers A, Cayrol R, Bernard M, et al. Human TH17 Lymphocytes Promote Blood-Brain Barrier Disruption and Central Nervous System Inflammation. Nat Med (2007) 13:1173–5. doi: 10.1038/nm1651
49. Kebir H, Ifergan I, Alvarez JI, Bernard M, Poirier J, Arbour N, et al. Preferential Recruitment of Interferon-Gamma-Expressing TH17 Cells in Multiple Sclerosis. Ann Neurol (2009) 66:390–402. doi: 10.1002/ana.21748
50. Huppert J, Closhen D, Croxford A, White R, Kulig P, Pietrowski E, et al. Cellular Mechanisms of IL-17-Induced Blood-Brain Barrier Disruption. FASEB J (2010) 24:1023–34. doi: 10.1096/fj.09-141978
51. Profaci CP, Munji RN, Pulido RS, Daneman R. The Blood-Brain Barrier in Health and Disease: Important Unanswered Questions. J Exp Med (2020) 217:e20190062. doi: 10.1084/jem.20190062
52. Mastorakos P, McGavern D. The Anatomy and Immunology of Vasculature in the Central Nervous System. Sci Immunol (2019) 4:eaav0492. doi: 10.1126/sciimmunol.aav0492
53. Michinaga S, Koyama Y. Dual Roles of Astrocyte-Derived Factors in Regulation of Blood-Brain Barrier Function After Brain Damage. Int J Mol Sci (2019) 20:571–93. doi: 10.3390/ijms20030571
54. Eilam R, Segal M, Malach R, Sela M, Arnon R, Aharoni R. Astrocyte Disruption of Neurovascular Communication Is Linked to Cortical Damage in an Animal Model of Multiple Sclerosis. Glia (2018) 66:1098–117. doi: 10.1002/glia.23304
55. Koehler RC, Roman RJ, Harder DR. Astrocytes and the Regulation of Cerebral Blood Flow. Trends Neurosci (2009) 32:160–9. doi: 10.1016/j.tins.2008.11.005
56. das Neves SP, Sousa JC, Sousa N, Cerqueira JJ, Marques F. Altered Astrocytic Function in Experimental Neuroinflammation and Multiple Sclerosis. Glia (2021) 69:1341–68. doi: 10.1002/glia.23940
57. Masaki K, Suzuki SO, Matsushita T, Matsuoka T, Imamura S, Yamasaki R, et al. Connexin 43 Astrocytopathy Linked to Rapidly Progressive Multiple Sclerosis and Neuromyelitis Optica. PloS One (2013) 8:e72919. doi: 10.1371/journal.pone.0072919
58. Ezan P, Andre P, Cisternino S, Saubamea B, Boulay AC, Doutremer S, et al. Deletion of Astroglial Connexins Weakens the Blood-Brain Barrier. J Cereb Blood Flow Metab (2012) 32:1457–67. doi: 10.1038/jcbfm.2012.45
59. Errede M, Girolamo F, Ferrara G, Strippoli M, Morando S, Boldrin V, et al. Blood-Brain Barrier Alterations in the Cerebral Cortex in Experimental Autoimmune Encephalomyelitis. J Neuropathol Exp Neurol (2012) 71:840–54. doi: 10.1097/NEN.0b013e31826ac110
60. Uchida Y, Sumiya T, Tachikawa M, Yamakawa T, Murata S, Yagi Y, et al. Involvement of Claudin-11 in Disruption of Blood-Brain, -Spinal Cord, and -Arachnoid Barriers in Multiple Sclerosis. Mol Neurobiol (2019) 56:2039–56. doi: 10.1007/s12035-018-1207-5
61. Chapouly C, Tadesse Argaw A, Horng S, Castro K, Zhang J, Asp L, et al. Astrocytic TYMP and VEGFA Drive Blood-Brain Barrier Opening in Inflammatory Central Nervous System Lesions. Brain (2015) 138:1548–67. doi: 10.1093/brain/awv077
62. Argaw AT, Asp L, Zhang J, Navrazhina K, Pham T, Mariani JN, et al. Astrocyte-Derived VEGF-A Drives Blood-Brain Barrier Disruption in CNS Inflammatory Disease. J Clin Invest (2012) 122:2454–68. doi: 10.1172/JCI60842
63. Roberts TK, Eugenin EA, Lopez L, Romero IA, Weksler BB, Couraud PO, et al. CCL2 Disrupts the Adherens Junction: Implications for Neuroinflammation. Lab Invest (2012) 92:1213–33. doi: 10.1038/labinvest.2012.80
64. Stamatovic SM, Keep RF, Wang MM, Jankovic I, Andjelkovic AV. Caveolae-Mediated Internalization of Occludin and Claudin-5 During CCL2-Induced Tight Junction Remodeling in Brain Endothelial Cells. J Biol Chem (2009) 284:19053–66. doi: 10.1074/jbc.M109.000521
65. Prins M, Dutta R, Baselmans B, Breve JJ, Bol JG, Deckard SA, et al. Discrepancy in CCL2 and CCR2 Expression in White Versus Grey Matter Hippocampal Lesions of Multiple Sclerosis Patients. Acta Neuropathol Commun (2014) 2:98. doi: 10.1186/s40478-014-0098-6
66. Moreno M, Bannerman P, Ma J, Guo F, Miers L, Soulika AM, et al. Conditional Ablation of Astroglial CCL2 Suppresses CNS Accumulation of M1 Macrophages and Preserves Axons in Mice With MOG Peptide EAE. J Neurosci (2014) 34:8175–85. doi: 10.1523/JNEUROSCI.1137-14.2014
67. Meares GP, Ma X, Qin H, Benveniste EN. Regulation of CCL20 Expression in Astrocytes by IL-6 and IL-17. Glia (2012) 60:771–81. doi: 10.1002/glia.22307
68. Reboldi A, Coisne C, Baumjohann D, Benvenuto F, Bottinelli D, Lira S, et al. C-C Chemokine Receptor 6-Regulated Entry of TH-17 Cells Into the CNS Through the Choroid Plexus is Required for the Initiation of EAE. Nat Immunol (2009) 10:514–23. doi: 10.1038/ni.1716
69. Mederos S, Gonzalez-Arias C, Perea G. Astrocyte-Neuron Networks: A Multilane Highway of Signaling for Homeostatic Brain Function. Front Synaptic Neurosci (2018) 10:45. doi: 10.3389/fnsyn.2018.00045
70. Chung WS, Allen NJ, Eroglu C. Astrocytes Control Synapse Formation, Function, and Elimination. Cold Spring Harb Perspect Biol (2015) 7:a020370. doi: 10.1101/cshperspect.a020370
71. Mahmoud S, Gharagozloo M, Simard C, Gris D. Astrocytes Maintain Glutamate Homeostasis in the CNS by Controlling the Balance Between Glutamate Uptake and Release. Cells (2019) 8:184–211. doi: 10.3390/cells8020184
72. Lau A, Tymianski M. Glutamate Receptors, Neurotoxicity and Neurodegeneration. Pflugers Arch (2010) 460:525–42. doi: 10.1007/s00424-010-0809-1
73. Wang Y, Qin ZH. Molecular and Cellular Mechanisms of Excitotoxic Neuronal Death. Apoptosis (2010) 15:1382–402. doi: 10.1007/s10495-010-0481-0
74. Sarchielli P, Greco L, Floridi A, Floridi A, Gallai V. Excitatory Amino Acids and Multiple Sclerosis: Evidence From Cerebrospinal Fluid. Arch Neurol (2003) 60:1082–8. doi: 10.1001/archneur.60.8.1082
75. Werner P, Pitt D, Raine CS. Multiple Sclerosis: Altered Glutamate Homeostasis in Lesions Correlates With Oligodendrocyte and Axonal Damage. Ann Neurol (2001) 50:169–80. doi: 10.1002/ana.1077
76. Korn T, Magnus T, Jung S. Autoantigen Specific T Cells Inhibit Glutamate Uptake in Astrocytes by Decreasing Expression of Astrocytic Glutamate Transporter GLAST: A Mechanism Mediated by Tumor Necrosis Factor-Alpha. FASEB J (2005) 19:1878–80. doi: 10.1096/fj.05-3748fje
77. Sulkowski G, Dabrowska-Bouta B, Salinska E, Struzynska L. Modulation of Glutamate Transport and Receptor Binding by Glutamate Receptor Antagonists in EAE Rat Brain. PloS One (2014) 9:e113954. doi: 10.1371/journal.pone.0113954
78. Ohgoh M, Hanada T, Smith T, Hashimoto T, Ueno M, Yamanishi Y, et al. Altered Expression of Glutamate Transporters in Experimental Autoimmune Encephalomyelitis. J Neuroimmunol (2002) 125:170–8. doi: 10.1016/s0165-5728(02)00029-2
79. Mitosek-Szewczyk K, Sulkowski G, Stelmasiak Z, Struzynska L. Expression of Glutamate Transporters GLT-1 and GLAST in Different Regions of Rat Brain During the Course of Experimental Autoimmune Encephalomyelitis. Neuroscience (2008) 155:45–52. doi: 10.1016/j.neuroscience.2008.05.025
80. Ha D, Bing SJ, Ahn G, Kim J, Cho J, Kim A, et al. Blocking Glutamate Carboxypeptidase II Inhibits Glutamate Excitotoxicity and Regulates Immune Responses in Experimental Autoimmune Encephalomyelitis. FEBS J (2016) 283:3438–56. doi: 10.1111/febs.13816
81. Seifert G, Henneberger C, Steinhauser C. Diversity of Astrocyte Potassium Channels: An Update. Brain Res Bull (2018) 136:26–36. doi: 10.1016/j.brainresbull.2016.12.002
82. Schirmer L, Srivastava R, Kalluri SR, Bottinger S, Herwerth M, Carassiti D, et al. Differential Loss of KIR4.1 Immunoreactivity in Multiple Sclerosis Lesions. Ann Neurol (2014) 75:810–28. doi: 10.1002/ana.24168
83. Srivastava R, Aslam M, Kalluri SR, Schirmer L, Buck D, Tackenberg B, et al. Potassium Channel KIR4.1 as an Immune Target in Multiple Sclerosis. N Engl J Med (2012) 367:115–23. doi: 10.1056/NEJMoa1110740
84. Magistretti PJ, Allaman I. Lactate in the Brain: From Metabolic End-Product to Signalling Molecule. Nat Rev Neurosci (2018) 19:235–49. doi: 10.1038/nrn.2018.19
85. Zeis T, Allaman I, Gentner M, Schroder K, Tschopp J, Magistretti PJ, et al. Metabolic Gene Expression Changes in Astrocytes in Multiple Sclerosis Cerebral Cortex Are Indicative of Immune-Mediated Signaling. Brain Behav Immun (2015) 48:313–25. doi: 10.1016/j.bbi.2015.04.013
86. Ponath G, Lincoln MR, Levine-Ritterman M, Park C, Dahlawi S, Mubarak M, et al. Enhanced Astrocyte Responses Are Driven by a Genetic Risk Allele Associated With Multiple Sclerosis. Nat Commun (2018) 9:5337. doi: 10.1038/s41467-018-07785-8
87. Chandra A, Xu YM. Cholesterol: A Necessary Evil From a Multiple Sclerosis Perspective. Clin Exp Neuroimmunology (2016) 7:145–57. doi: 10.1111/cen3.12289
88. Itoh N, Itoh Y, Tassoni A, Ren E, Kaito M, Ohno A, et al. Cell-Specific and Region-Specific Transcriptomics in the Multiple Sclerosis Model: Focus on Astrocytes. Proc Natl Acad Sci USA (2018) 115:E302–9. doi: 10.1073/pnas.1716032115
89. Chiareli RA, Carvalho GA, Marques BL, Mota LS, Oliveira-Lima OC, Gomes RM, et al. The Role of Astrocytes in the Neurorepair Process. Front Cell Dev Biol (2021) 9:665795. doi: 10.3389/fcell.2021.665795
90. Prajeeth CK, Kronisch J, Khorooshi R, Knier B, Toft-Hansen H, Gudi V, et al. Effectors of Th1 and Th17 Cells Act on Astrocytes and Augment Their Neuroinflammatory Properties. J Neuroinflamm (2017) 14:204. doi: 10.1186/s12974-017-0978-3
91. Colombo E, Cordiglieri C, Melli G, Newcombe J, Krumbholz M, Parada LF, et al. Stimulation of the Neurotrophin Receptor TrkB on Astrocytes Drives Nitric Oxide Production and Neurodegeneration. J Exp Med (2012) 209:521–35. doi: 10.1084/jem.20110698
92. Ponath G, Park C, Pitt D. The Role of Astrocytes in Multiple Sclerosis. Front Immunol (2018) 9:217. doi: 10.3389/fimmu.2018.00217
93. Domingues HS, Portugal CC, Socodato R, Relvas JB. Oligodendrocyte, Astrocyte, and Microglia Crosstalk in Myelin Development, Damage, and Repair. Front Cell Dev Biol (2016) 4:71. doi: 10.3389/fcell.2016.00071
94. Traiffort E, Kassoussi A, Zahaf A, Laouarem Y. Astrocytes and Microglia as Major Players of Myelin Production in Normal and Pathological Conditions. Front Cell Neurosci (2020) 14:79. doi: 10.3389/fncel.2020.00079
95. Linnerbauer M, Rothhammer V. Protective Functions of Reactive Astrocytes Following Central Nervous System Insult. Front Immunol (2020) 11:573256. doi: 10.3389/fimmu.2020.573256
96. Rawji KS, Gonzalez Martinez GA, Sharma A, Franklin RJM. The Role of Astrocytes in Remyelination. Trends Neurosci (2020) 43:596–607. doi: 10.1016/j.tins.2020.05.006
97. Fulmer CG, VonDran MW, Stillman AA, Huang Y, Hempstead BL, Dreyfus CF. Astrocyte-Derived BDNF Supports Myelin Protein Synthesis After Cuprizone-Induced Demyelination. J Neurosci (2014) 34:8186–96. doi: 10.1523/JNEUROSCI.4267-13.2014
98. Back SA, Tuohy TM, Chen H, Wallingford N, Craig A, Struve J, et al. Hyaluronan Accumulates in Demyelinated Lesions and Inhibits Oligodendrocyte Progenitor Maturation. Nat Med (2005) 11:966–72. doi: 10.1038/nm1279
99. Zhang J, Xu X, Liu H, Jin L, Shen X, Xie C, et al. Astrocytic YAP Prevents the Demyelination Through Promoting Expression of Cholesterol Synthesis Genes in Experimental Autoimmune Encephalomyelitis. Cell Death Dis (2021) 12:907. doi: 10.1038/s41419-021-04203-8
100. Tress O, Maglione M, May D, Pivneva T, Richter N, Seyfarth J, et al. Panglial Gap Junctional Communication Is Essential for Maintenance of Myelin in the CNS. J Neurosci (2012) 32:7499–518. doi: 10.1523/JNEUROSCI.0392-12.2012
101. Philippot C, Griemsmann S, Jabs R, Seifert G, Kettenmann H, Steinhauser C. Astrocytes and Oligodendrocytes in the Thalamus Jointly Maintain Synaptic Activity by Supplying Metabolites. Cell Rep (2021) 34:108642. doi: 10.1016/j.celrep.2020.108642
102. Markoullis K, Sargiannidou I, Gardner C, Hadjisavvas A, Reynolds R, Kleopa KA. Disruption of Oligodendrocyte Gap Junctions in Experimental Autoimmune Encephalomyelitis. Glia (2012) 60:1053–66. doi: 10.1002/glia.22334
103. Markoullis K, Sargiannidou I, Schiza N, Hadjisavvas A, Roncaroli F, Reynolds R, et al. Gap Junction Pathology in Multiple Sclerosis Lesions and Normal-Appearing White Matter. Acta Neuropathol (2012) 123:873–86. doi: 10.1007/s00401-012-0978-4
104. Markoullis K, Sargiannidou I, Schiza N, Roncaroli F, Reynolds R, Kleopa KA. Oligodendrocyte Gap Junction Loss and Disconnection From Reactive Astrocytes in Multiple Sclerosis Gray Matter. J Neuropathol Exp Neurol (2014) 73:865–79. doi: 10.1097/NEN.0000000000000106
105. Mockus TE, Munie A, Atkinson JR, Segal BM. Encephalitogenic and Regulatory CD8 T Cells in Multiple Sclerosis and Its Animal Models. J Immunol (2021) 206:3–10. doi: 10.4049/jimmunol.2000797
106. Annunziato F, Cosmi L, Liotta F, Maggi E, Romagnani S. Human Th1 Dichotomy: Origin, Phenotype and Biologic Activities. Immunology (2014) 144:343–51. doi: 10.1111/imm.12399
107. Murphy AC, Lalor SJ, Lynch MA, Mills KH. Infiltration of Th1 and Th17 Cells and Activation of Microglia in the CNS During the Course of Experimental Autoimmune Encephalomyelitis. Brain Behav Immun (2010) 24:641–51. doi: 10.1016/j.bbi.2010.01.014
108. Prajeeth CK, Lohr K, Floess S, Zimmermann J, Ulrich R, Gudi V, et al. Effector Molecules Released by Th1 But Not Th17 Cells Drive an M1 Response in Microglia. Brain Behav Immun (2014) 37:248–59. doi: 10.1016/j.bbi.2014.01.001
109. Prajeeth CK, Huehn J, Stangel M. Regulation of Neuroinflammatory Properties of Glial Cells by T Cell Effector Molecules. Neural Regener Res (2018) 13:234–6. doi: 10.4103/1673-5374.226385
110. Hashioka S, Klegeris A, Schwab C, McGeer PL. Interferon-Gamma-Dependent Cytotoxic Activation of Human Astrocytes and Astrocytoma Cells. Neurobiol Aging (2009) 30:1924–35. doi: 10.1016/j.neurobiolaging.2008.02.019
111. Ding X, Yan Y, Li X, Li K, Ciric B, Yang J, et al. Silencing IFN-Gamma Binding/Signaling in Astrocytes Versus Microglia Leads to Opposite Effects on Central Nervous System Autoimmunity. J Immunol (2015) 194:4251–64. doi: 10.4049/jimmunol.1303321
112. Wang X, Mulas F, Yi W, Brunn A, Nishanth G, Just S, et al. OTUB1 Inhibits CNS Autoimmunity by Preventing IFN-Gamma-Induced Hyperactivation of Astrocytes. EMBO J (2019) 38:e100947. doi: 10.15252/embj.2018100947
113. Prajeeth CK, Dittrich-Breiholz O, Talbot SR, Robert PA, Huehn J, Stangel M. IFN-Gamma Producing Th1 Cells Induce Different Transcriptional Profiles in Microglia and Astrocytes. Front Cell Neurosci (2018) 12:352. doi: 10.3389/fncel.2018.00352
114. Yang JF, Tao HQ, Liu YM, Zhan XX, Liu Y, Wang XY, et al. Characterization of the Interaction Between Astrocytes and Encephalitogenic Lymphocytes During the Development of Experimental Autoimmune Encephalitomyelitis (EAE) in Mice. Clin Exp Immunol (2012) 170:254–65. doi: 10.1111/j.1365-2249.2012.04661.x
115. Zeinstra E, Wilczak N, Streefland C, De Keyser J. Astrocytes in Chronic Active Multiple Sclerosis Plaques Express MHC Class II Molecules. Neuroreport (2000) 11:89–91. doi: 10.1097/00001756-200001170-00018
116. Zeinstra E, Wilczak N, De Keyser J. Reactive Astrocytes in Chronic Active Lesions of Multiple Sclerosis Express Co-Stimulatory Molecules B7-1 and B7-2. J Neuroimmunol (2003) 135:166–71. doi: 10.1016/s0165-5728(02)00462-9
117. Tan L, Gordon KB, Mueller JP, Matis LA, Miller SD. Presentation of Proteolipid Protein Epitopes and B7-1-Dependent Activation of Encephalitogenic T Cells by IFN-Gamma-Activated SJL/J Astrocytes. J Immunol (1998) 160:4271–9.
118. Nikcevich KM, Gordon KB, Tan L, Hurst SD, Kroepfl JF, Gardinier M, et al. IFN-Gamma-Activated Primary Murine Astrocytes Express B7 Costimulatory Molecules and Prime Naive Antigen-Specific T Cells. J Immunol (1997) 158:614–21.
119. Smith BC, Sinyuk M, Jenkins JE 3rd, Psenicka MW, Williams JL. The Impact of Regional Astrocyte Interferon-Gamma Signaling During Chronic Autoimmunity: A Novel Role for the Immunoproteasome. J Neuroinflamm (2020) 17:184. doi: 10.1186/s12974-020-01861-x
120. Hindinger C, Bergmann CC, Hinton DR, Phares TW, Parra GI, Hussain S, et al. IFN-Gamma Signaling to Astrocytes Protects From Autoimmune Mediated Neurological Disability. PloS One (2012) 7:e42088. doi: 10.1371/journal.pone.0042088
121. Watanabe M, Masaki K, Yamasaki R, Kawanokuchi J, Takeuchi H, Matsushita T, et al. Th1 Cells Downregulate Connexin 43 Gap Junctions in Astrocytes via Microglial Activation. Sci Rep (2016) 6:38387. doi: 10.1038/srep38387
122. Annunziato F, Cosmi L, Liotta F, Maggi E, Romagnani S. Defining the Human T Helper 17 Cell Phenotype. Trends Immunol (2012) 33:505–12. doi: 10.1016/j.it.2012.05.004
123. Volpe E, Battistini L, Borsellino G. Advances in T Helper 17 Cell Biology: Pathogenic Role and Potential Therapy in Multiple Sclerosis. Mediators Inflamm (2015) 2015:475158. doi: 10.1155/2015/475158
124. Tahmasebinia F, Pourgholaminejad A. The Role of Th17 Cells in Auto-Inflammatory Neurological Disorders. Prog Neuropsychopharmacol Biol Psychiatry (2017) 79:408–16. doi: 10.1016/j.pnpbp.2017.07.023
125. Das Sarma J, Ciric B, Marek R, Sadhukhan S, Caruso ML, Shafagh J, et al. Functional Interleukin-17 Receptor A is Expressed in Central Nervous System Glia and Upregulated in Experimental Autoimmune Encephalomyelitis. J Neuroinflamm (2009) 6:14. doi: 10.1186/1742-2094-6-14
126. Yi H, Bai Y, Zhu X, Lin L, Zhao L, Wu X, et al. IL-17A Induces MIP-1alpha Expression in Primary Astrocytes via Src/MAPK/PI3K/NF-kB Pathways: Implications for Multiple Sclerosis. J Neuroimmune Pharmacol (2014) 9:629–41. doi: 10.1007/s11481-014-9553-1
127. Liu X, Zhou F, Yang Y, Wang W, Niu L, Zuo D, et al. MiR-409-3p and MiR-1896 Co-Operatively Participate in IL-17-Induced Inflammatory Cytokine Production in Astrocytes and Pathogenesis of EAE Mice via Targeting SOCS3/STAT3 Signaling. Glia (2019) 67:101–12. doi: 10.1002/glia.23530
128. Kang Z, Altuntas CZ, Gulen MF, Liu C, Giltiay N, Qin H, et al. Astrocyte-Restricted Ablation of Interleukin-17-Induced Act1-Mediated Signaling Ameliorates Autoimmune Encephalomyelitis. Immunity (2010) 32:414–25. doi: 10.1016/j.immuni.2010.03.004
129. Huang G, Wang Y, Vogel P, Chi H. Control of IL-17 Receptor Signaling and Tissue Inflammation by the P38alpha-MKP-1 Signaling Axis in a Mouse Model of Multiple Sclerosis. Sci Signal (2015) 8:ra24. doi: 10.1126/scisignal.aaa2147
130. Yan Y, Ding X, Li K, Ciric B, Wu S, Xu H, et al. CNS-Specific Therapy for Ongoing EAE by Silencing IL-17 Pathway in Astrocytes. Mol Ther (2012) 20:1338–48. doi: 10.1038/mt.2012.12
131. Ma X, Reynolds SL, Baker BJ, Li X, Benveniste EN, Qin H. IL-17 Enhancement of the IL-6 Signaling Cascade in Astrocytes. J Immunol (2010) 184:4898–906. doi: 10.4049/jimmunol.1000142
132. Elain G, Jeanneau K, Rutkowska A, Mir AK, Dev KK. The Selective Anti-IL17A Monoclonal Antibody Secukinumab (AIN457) Attenuates IL17A-Induced Levels of IL6 in Human Astrocytes. Glia (2014) 62:725–35. doi: 10.1002/glia.22637
133. Shan K, Pang R, Zhao C, Liu X, Gao W, Zhang J, et al. IL-17-Triggered Downregulation of miR-497 Results in High HIF-1alpha Expression and Consequent IL-1beta and IL-6 Production by Astrocytes in EAE Mice. Cell Mol Immunol (2017) 14:909–23. doi: 10.1038/cmi.2017.12
134. Williams JL, Manivasagam S, Smith BC, Sim J, Vollmer LL, Daniels BP, et al. Astrocyte-T Cell Crosstalk Regulates Region-Specific Neuroinflammation. Glia (2020) 68:1361–74. doi: 10.1002/glia.23783
135. Kostic M, Zivkovic N, Cvetanovic A, Stojanovic I, Colic M. IL-17 Signalling in Astrocytes Promotes Glutamate Excitotoxicity: Indications for the Link Between Inflammatory and Neurodegenerative Events in Multiple Sclerosis. Mult Scler Relat Disord (2017) 11:12–7. doi: 10.1016/j.msard.2016.11.006
136. Becher B, Segal BM. T(H)17 Cytokines in Autoimmune Neuro-Inflammation. Curr Opin Immunol (2011) 23:707–12. doi: 10.1016/j.coi.2011.08.005
137. Edwards LJ, Robins RA, Constantinescu CS. Th17/Th1 Phenotype in Demyelinating Disease. Cytokine (2010) 50:19–23. doi: 10.1016/j.cyto.2009.12.003
138. Cao Y, Goods BA, Raddassi K, Nepom GT, Kwok WW, Love JC, et al. Functional Inflammatory Profiles Distinguish Myelin-Reactive T Cells From Patients With Multiple Sclerosis. Sci Transl Med (2015) 7:287ra274. doi: 10.1126/scitranslmed.aaa8038
139. Paroni M, Maltese V, De Simone M, Ranzani V, Larghi P, Fenoglio C, et al. Recognition of Viral and Self-Antigens by TH1 and TH1/TH17 Central Memory Cells in Patients With Multiple Sclerosis Reveals Distinct Roles in Immune Surveillance and Relapses. J Allergy Clin Immunol (2017) 140:797–808. doi: 10.1016/j.jaci.2016.11.045
140. van Langelaar J, van der Vuurst de Vries RM, Janssen M, Wierenga-Wolf AF, Spilt IM, Siepman TA, et al. T Helper 17.1 Cells Associate With Multiple Sclerosis Disease Activity: Perspectives for Early Intervention. Brain (2018) 141:1334–49. doi: 10.1093/brain/awy069
141. Lee Y, Awasthi A, Yosef N, Quintana FJ, Xiao S, Peters A, et al. Induction and Molecular Signature of Pathogenic TH17 Cells. Nat Immunol (2012) 13:991–9. doi: 10.1038/ni.2416
142. Hu D, Notarbartolo S, Croonenborghs T, Patel B, Cialic R, Yang TH, et al. Transcriptional Signature of Human Pro-Inflammatory TH17 Cells Identifies Reduced IL10 Gene Expression in Multiple Sclerosis. Nat Commun (2017) 8:1600. doi: 10.1038/s41467-017-01571-8
143. Yang W, Bai Y, Xiong Y, Zhang J, Chen S, Zheng X, et al. Potentiating the Antitumour Response of CD8(+) T Cells by Modulating Cholesterol Metabolism. Nature (2016) 531:651–5. doi: 10.1038/nature17412
144. Loos J, Schmaul S, Noll TM, Paterka M, Schillner M, Loffel JT, et al. Functional Characteristics of Th1, Th17, and Ex-Th17 Cells in EAE Revealed by Intravital Two-Photon Microscopy. J Neuroinflamm (2020) 17:357. doi: 10.1186/s12974-020-02021-x
145. Salou M, Nicol B, Garcia A, Laplaud DA. Involvement of CD8(+) T Cells in Multiple Sclerosis. Front Immunol (2015) 6:604. doi: 10.3389/fimmu.2015.00604
146. Saligrama N, Zhao F, Sikora MJ, Serratelli WS, Fernandes RA, Louis DM, et al. Opposing T Cell Responses in Experimental Autoimmune Encephalomyelitis. Nature (2019) 572:481–7. doi: 10.1038/s41586-019-1467-x
147. Beltran E, Gerdes LA, Hansen J, Flierl-Hecht A, Krebs S, Blum H, et al. Early Adaptive Immune Activation Detected in Monozygotic Twins With Prodromal Multiple Sclerosis. J Clin Invest (2019) 129:4758–68. doi: 10.1172/JCI128475
148. Suidan GL, McDole JR, Chen Y, Pirko I, Johnson AJ. Induction of Blood Brain Barrier Tight Junction Protein Alterations by CD8 T Cells. PloS One (2008) 3:e3037. doi: 10.1371/journal.pone.0003037
149. Huseby ES, Kamimura D, Arima Y, Parello CS, Sasaki K, Murakami M. Role of T Cell-Glial Cell Interactions in Creating and Amplifying Central Nervous System Inflammation and Multiple Sclerosis Disease Symptoms. Front Cell Neurosci (2015) 9:295. doi: 10.3389/fncel.2015.00295
150. Huber M, Heink S, Pagenstecher A, Reinhard K, Ritter J, Visekruna A, et al. IL-17A Secretion by CD8+ T Cells Supports Th17-Mediated Autoimmune Encephalomyelitis. J Clin Invest (2013) 123:247–60. doi: 10.1172/JCI63681
151. Saikali P, Antel JP, Pittet CL, Newcombe J, Arbour N. Contribution of Astrocyte-Derived IL-15 to CD8 T Cell Effector Functions in Multiple Sclerosis. J Immunol (2010) 185:5693–703. doi: 10.4049/jimmunol.1002188
152. Prajapati K, Perez C, Rojas LBP, Burke B, Guevara-Patino JA. Functions of NKG2D in CD8(+) T Cells: An Opportunity for Immunotherapy. Cell Mol Immunol (2018) 15:470–9. doi: 10.1038/cmi.2017.161
153. Carmena Moratalla A, Carpentier Solorio Y, Lemaitre F, Farzam-Kia N, Levert A, Zandee SEJ, et al. Stress Signal ULBP4, an NKG2D Ligand, Is Upregulated in Multiple Sclerosis and Shapes CD8(+) T-Cell Behaviors. Neurol Neuroimmunol Neuroinflamm (2022) 9:e1119. doi: 10.1212/NXI.0000000000001119
154. De Kleijn KMA, Martens GJM. Molecular Effects of FDA-Approved Multiple Sclerosis Drugs on Glial Cells and Neurons of the Central Nervous System. Int J Mol Sci (2020) 21:4229–78. doi: 10.3390/ijms21124229
155. Neuhaus O, Farina C, Yassouridis A, Wiendl H, Then Bergh F, Dose T, et al. Multiple Sclerosis: Comparison of Copolymer-1- Reactive T Cell Lines From Treated and Untreated Subjects Reveals Cytokine Shift From T Helper 1 to T Helper 2 Cells. Proc Natl Acad Sci USA (2000) 97:7452–7. doi: 10.1073/pnas.97.13.7452
156. Valenzuela RM, Costello K, Chen M, Said A, Johnson KP, Dhib-Jalbut S. Clinical Response to Glatiramer Acetate Correlates With Modulation of IFN-Gamma and IL-4 Expression in Multiple Sclerosis. Mult Scler (2007) 13:754–62. doi: 10.1177/1352458506074510
157. Chen M, Gran B, Costello K, Johnson K, Martin R, Dhib-Jalbut S. Glatiramer Acetate Induces a Th2-Biased Response and Crossreactivity With Myelin Basic Protein in Patients With MS. Mult Scler (2001) 7:209–19. doi: 10.1177/135245850100700401
158. Hong J, Li N, Zhang X, Zheng B, Zhang JZ. Induction of CD4+CD25+ Regulatory T Cells by Copolymer-I Through Activation of Transcription Factor Foxp3. Proc Natl Acad Sci USA (2005) 102:6449–54. doi: 10.1073/pnas.0502187102
159. Aharoni R, Kayhan B, Eilam R, Sela M, Arnon R. Glatiramer Acetate-Specific T Cells in the Brain Express T Helper 2/3 Cytokines and Brain-Derived Neurotrophic Factor in Situ. Proc Natl Acad Sci USA (2003) 100:14157–62. doi: 10.1073/pnas.2336171100
160. Fan YY, Huo J. A1/A2 Astrocytes in Central Nervous System Injuries and Diseases: Angels or Devils? Neurochem Int (2021) 148:105080. doi: 10.1016/j.neuint.2021.105080
161. Longbrake EE, Ramsbottom MJ, Cantoni C, Ghezzi L, Cross AH, Piccio L. Dimethyl Fumarate Selectively Reduces Memory T Cells in Multiple Sclerosis Patients. Mult Scler (2016) 22:1061–70. doi: 10.1177/1352458515608961
162. Ghadiri M, Rezk A, Li R, Evans A, Luessi F, Zipp F, et al. Dimethyl Fumarate-Induced Lymphopenia in MS Due to Differential T-Cell Subset Apoptosis. Neurol Neuroimmunol Neuroinflamm (2017) 4:e340. doi: 10.1212/NXI.0000000000000340
163. Gross CC, Schulte-Mecklenbeck A, Klinsing S, Posevitz-Fejfar A, Wiendl H, Klotz L. Dimethyl Fumarate Treatment Alters Circulating T Helper Cell Subsets in Multiple Sclerosis. Neurol Neuroimmunol Neuroinflamm (2016) 3:e183. doi: 10.1212/NXI.0000000000000183
164. Buckle G, Bandari D, Greenstein J, Gudesblatt M, Khatri B, Kita M, et al. Effect of Dimethyl Fumarate on Lymphocyte Subsets in Patients With Relapsing Multiple Sclerosis. Mult Scler J Exp Transl Clin (2020) 6:2055217320918619. doi: 10.1177/2055217320918619
165. Wu Q, Wang Q, Mao G, Dowling CA, Lundy SK, Mao-Draayer Y. Dimethyl Fumarate Selectively Reduces Memory T Cells and Shifts the Balance Between Th1/Th17 and Th2 in Multiple Sclerosis Patients. J Immunol (2017) 198:3069–80. doi: 10.4049/jimmunol.1601532
166. Medina S, Villarrubia N, Sainz de la Maza S, Lifante J, Costa-Frossard L, Roldan E, et al. Optimal Response to Dimethyl Fumarate Associates in MS With a Shift From an Inflammatory to a Tolerogenic Blood Cell Profile. Mult Scler (2018) 24:1317–27. doi: 10.1177/1352458517717088
167. Galloway DA, Williams JB, Moore CS. Effects of Fumarates on Inflammatory Human Astrocyte Responses and Oligodendrocyte Differentiation. Ann Clin Transl Neurol (2017) 4:381–91. doi: 10.1002/acn3.414
168. Brennan MS, Matos MF, Richter KE, Li B, Scannevin RH. The NRF2 Transcriptional Target, OSGIN1, Contributes to Monomethyl Fumarate-Mediated Cytoprotection in Human Astrocytes. Sci Rep (2017) 7:42054. doi: 10.1038/srep42054
169. Swindell WR, Bojanowski K, Chaudhuri RK. A Novel Fumarate, Isosorbide Di-(Methyl Fumarate) (IDMF), Replicates Astrocyte Transcriptome Responses to Dimethyl Fumarate (DMF) But Specifically Down-Regulates Genes Linked to a Reactive Phenotype. Biochem Biophys Res Commun (2020) 532:475–81. doi: 10.1016/j.bbrc.2020.08.079
170. Kipp M. Does Siponimod Exert Direct Effects in the Central Nervous System? Cells (2020) 9:1771. doi: 10.3390/cells9081771
171. Ghadiri M, Rezk A, Li R, Evans A, Giacomini PS, Barnett MH, et al. Pre-Treatment T-Cell Subsets Associate With Fingolimod Treatment Responsiveness in Multiple Sclerosis. Sci Rep (2020) 10:356. doi: 10.1038/s41598-019-57114-2
172. Wu Q, Mills EA, Wang Q, Dowling CA, Fisher C, Kirch B, et al. Siponimod Enriches Regulatory T and B Lymphocytes in Secondary Progressive Multiple Sclerosis. JCI Insight (2020) 5:e134251. doi: 10.1172/jci.insight.134251
173. Rothhammer V, Kenison JE, Tjon E, Takenaka MC, de Lima KA, Borucki DM, et al. Sphingosine 1-Phosphate Receptor Modulation Suppresses Pathogenic Astrocyte Activation and Chronic Progressive CNS Inflammation. Proc Natl Acad Sci USA (2017) 114:2012–7. doi: 10.1073/pnas.1615413114
174. Hoffmann FS, Hofereiter J, Rübsamen H, Melms J, Schwarz S, Faber H, et al. Fingolimod Induces Neuroprotective Factors in Human Astrocytes. J Neuroinflamm (2015) 12:184. doi: 10.1186/s12974-015-0393-6
175. Trkov Bobnar S, Stenovec M, Miš K, Pirkmajer S, Zorec R. Fingolimod Suppresses the Proinflammatory Status of Interferon-γ-Activated Cultured Rat Astrocytes. Mol Neurobiol (2019) 56:5971–86. doi: 10.1007/s12035-019-1481-x
176. Spampinato SF, Merlo S, Costantino G, Sano Y, Kanda T, Sortino MA. Decreased Astrocytic CCL2 Accounts for BAF-312 Effect on PBMCs Transendothelial Migration Through a Blood Brain Barrier in Vitro Model. J Neuroimmune Pharmacol (2021). doi: 10.1007/s11481-021-10016-5
177. Jonnalagadda D, Kihara Y, Rivera R, Chun J. S1P2-Galpha12 Signaling Controls Astrocytic Glutamate Uptake and Mitochondrial Oxygen Consumption. eNeuro (2021) 8:ENEURO.0040–0021.2021. doi: 10.1523/ENEURO.0040-21.2021
178. Colombo E, Pascente R, Triolo D, Bassani C, De Angelis A, Ruffini F, et al. Laquinimod Modulates Human Astrocyte Function and Dampens Astrocyte-Induced Neurotoxicity During Inflammation. Molecules (2020) 25:5403–17. doi: 10.3390/molecules25225403
179. Comi G, Dadon Y, Sasson N, Steinerman JR, Knappertz V, Vollmer TL, et al. CONCERTO: A Randomized, Placebo-Controlled Trial of Oral Laquinimod in Relapsing-Remitting Multiple Sclerosis. Multiple Sclerosis J (2021). doi: 10.1177/13524585211032803
180. Engel S, Jolivel V, Kraus SH-P, Zayoud M, Rosenfeld K, Tumani H, et al. Laquinimod Dampens IL-1β Signaling and Th17-Polarizing Capacity of Monocytes in Patients With MS. Neurol - Neuroimmunology Neuroinflamm (2021) 8:e908. doi: 10.1212/nxi.0000000000000908
181. Wegner C, Stadelmann C, Pförtner R, Raymond E, Feigelson S, Alon R, et al. Laquinimod Interferes With Migratory Capacity of T Cells and Reduces IL-17 Levels, Inflammatory Demyelination and Acute Axonal Damage in Mice With Experimental Autoimmune Encephalomyelitis. J Neuroimmunology (2010) 227:133–43. doi: 10.1016/j.jneuroim.2010.07.009
Keywords: multiple sclerosis, astrocytes, Th cells, inflammation, demyelination
Citation: Kunkl M, Amormino C, Tedeschi V, Fiorillo MT and Tuosto L (2022) Astrocytes and Inflammatory T Helper Cells: A Dangerous Liaison in Multiple Sclerosis. Front. Immunol. 13:824411. doi: 10.3389/fimmu.2022.824411
Received: 29 November 2021; Accepted: 13 January 2022;
Published: 08 February 2022.
Edited by:
Manolo Sambucci, Laboratory of Neuroimmunology, Santa Lucia Foundation (IRCCS), ItalyReviewed by:
Gordon Meares, West Virginia University, United StatesMarija Mostarica-Stojkovic, University of Belgrade, Serbia
Maksim Sinyuk, Abeona Therapeutics, United States
Gholamreza Azizi, Alborz University of Medical Sciences, Iran
Copyright © 2022 Kunkl, Amormino, Tedeschi, Fiorillo and Tuosto. This is an open-access article distributed under the terms of the Creative Commons Attribution License (CC BY). The use, distribution or reproduction in other forums is permitted, provided the original author(s) and the copyright owner(s) are credited and that the original publication in this journal is cited, in accordance with accepted academic practice. No use, distribution or reproduction is permitted which does not comply with these terms.
*Correspondence: Loretta Tuosto, loretta.tuosto@uniroma1.it
†These authors have contributed equally to this work