- 1Huazhong University of Science and Technology Union Shenzhen Hospital, Shenzhen, China
- 2Institute of Biomedicine and Biotechnology, Shenzhen Institutes of Advanced Technology, Chinese Academy of Sciences, Shenzhen, China
- 3The 6th Affiliated Hospital of Shenzhen University Health Science Center, Shenzhen, China
- 4School of Biomedical Science and Pharmacy, Faculty of Health and Medicine, Hunter Medical Research Institute, University of Newcastle, New Lambton Heights, NSW, Australia
- 5Department of Surgery, Sloan Kettering Institute Z427-D, Mortimer B. Zuckerman Research Center, Memorial Sloan Kettering Cancer Center, New York, NY, United States
Engineered nanomaterials (ENMs) have been widely exploited in several industrial domains as well as our daily life, raising concern over their potential adverse effects. While in general ENMs do not seem to have detrimental effects on immunity or induce severe inflammation, their indirect effects on immunity are less known. In particular, since the gut microbiota has been tightly associated with human health and immunity, it is possible that ingested ENMs could affect intestinal immunity indirectly by modulating the microbial community composition and functions. In this perspective, we provide a few pieces of evidence and discuss a possible link connecting ENM exposure, gut microbiota and host immune response. Some experimental works suggest that excessive exposure to ENMs could reshape the gut microbiota, thereby modulating the epithelium integrity and the inflammatory state in the intestine. Within such microenvironment, numerous microbiota-derived components, including but not limited to SCFAs and LPS, may serve as important effectors responsible of the ENM effect on intestinal immunity. Therefore, the gut microbiota is implicated as a crucial regulator of the intestinal immunity upon ENM exposure. This calls for including gut microbiota analysis within future work to assess ENM biocompatibility and immunosafety. This also calls for refinement of future studies that should be designed more elaborately and realistically to mimic the human exposure situation.
Introduction
Unique properties including large surface area, high catalytic properties and antimicrobial efficacy confer to engineered nanomaterials (ENMs) a significant range of applications in nanomedicine and consumer products (1, 2), raising public concerns about their biosafety. For example, nanoparticulate Ag, TiO2, ZnO and plastics are widely used in food additives (3), components of food packaging and containers (4, 5), and toothpaste (6). Oral exposure to these ENMs in our daily life is therefore likely through ingestion of food or water that deliberately or inadvertently contain ENMs. ENMs might therefore reach the gastro-intestinal tract (GIT) and interact with mucosal cells. Indeed, endocytosis of ENMs by intestinal epithelial cells (IECs) and various immune cells is observed using either conventional 2D in vitro models such as tumor cell lines (7, 8) or in vivo animal models (9). Moreover, it has been reported that ENMs could modulate innate/inflammatory immune responses upon direct interactions with neutrophils, macrophages, dendritic cells (DCs) and the complement system (10–13). Upon ingestion, ENMs most likely also come in contact with gut microbiota, i.e., the population of microbes residing in the intestinal lumen and mucosa. It has been long known that the gut microbiota is essential for the development of the immune system and for immune homeostasis (14). Recent observations suggest that the ENM effects on innate/inflammatory responses largely depend on the co-presence of bacterial agents such as lipopolysaccharide (LPS) (15, 16). Thus, it is a logical assumption that ENMs could affect immunity by altering gut microbiota, a concept that is currently unexplored.
Herein, we provide an overview of the current state-of-the-art, and discuss a hypothetical scenario in which ingested EMNs may affect host immunity by modulating the gut microbiota. From published in vivo studies in different models and with different ENMs, a high level of variability is found regarding the ENM effects on gut microbiota and local/systemic immunity (Table 1).
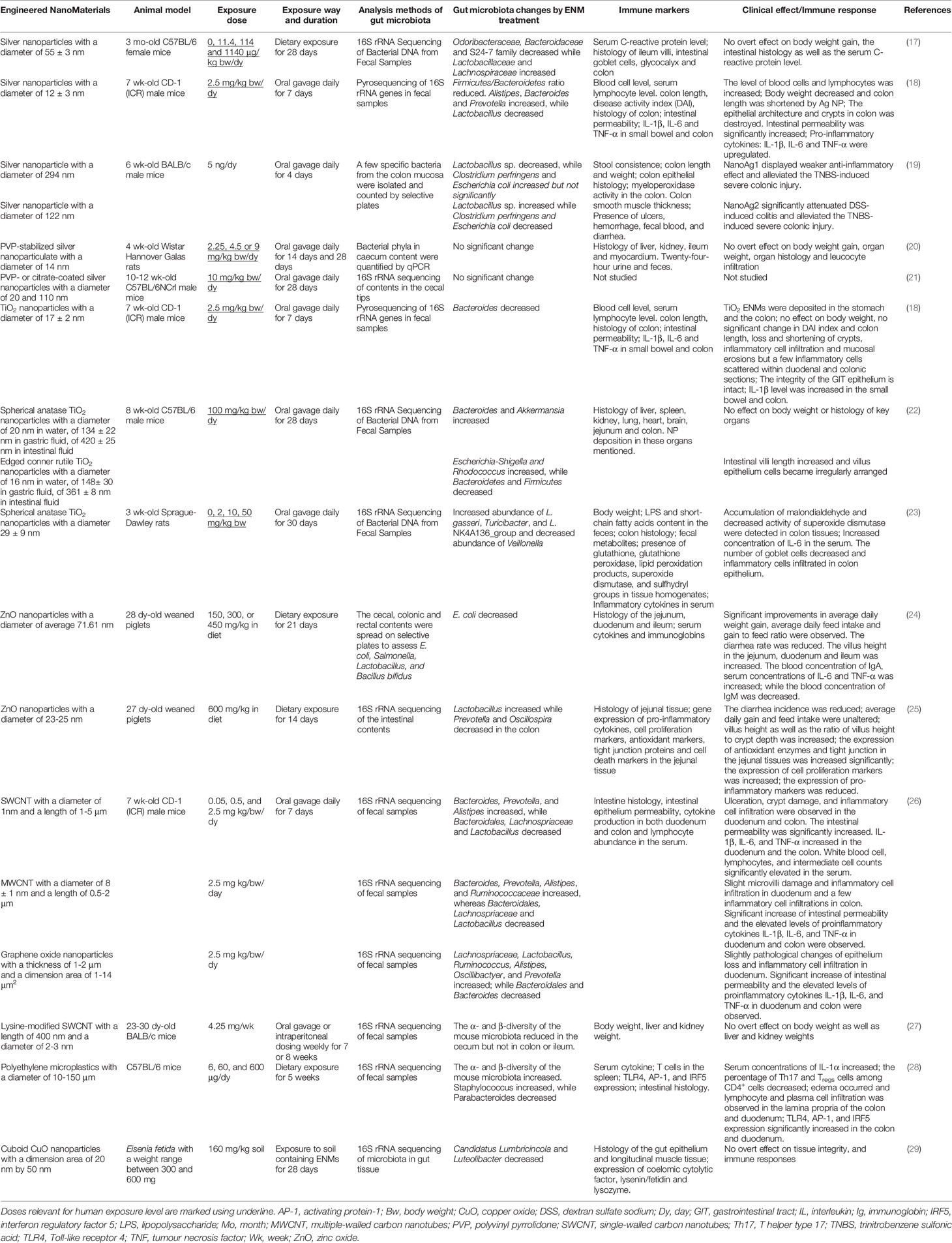
Table 1 Representative in vivo assays studying the impact of ENMs on gut microbiota and subsequent influences on intestinal immunity.
LPS and SCFAs: Two Representative Microbial Molecules Bridging Gut Microbiota and Intestinal Immunity
Mounting evidence has highlighted the tremendous contribution of gut microbiota to human physiology (30–35). Within this microbiota-immune system interaction, a large amount of microbial metabolites and components serve as potent effectors to orchestrate their communication (36, 37). We will specifically discuss hereafter the immunomodulatory effects of short-chain fatty acids (SCFAs) and LPS. More comprehensive information is shown in Figure 1 and extensively discussed in other excellent reviews (32, 33, 36–39). SCFAs are generated from indigestible oligosaccharides by gut commensals, including Lactobacillus, Bacteroides, Bifidobacterium, Feacalibacterium, etc. (40). LPS is the major membrane component of Gram-negative bacteria and has profound immunostimulatory and inflammatory capacity (41). The immunological effects of these microbiota-derived molecules are manifold, covering innate and adaptive immunity.
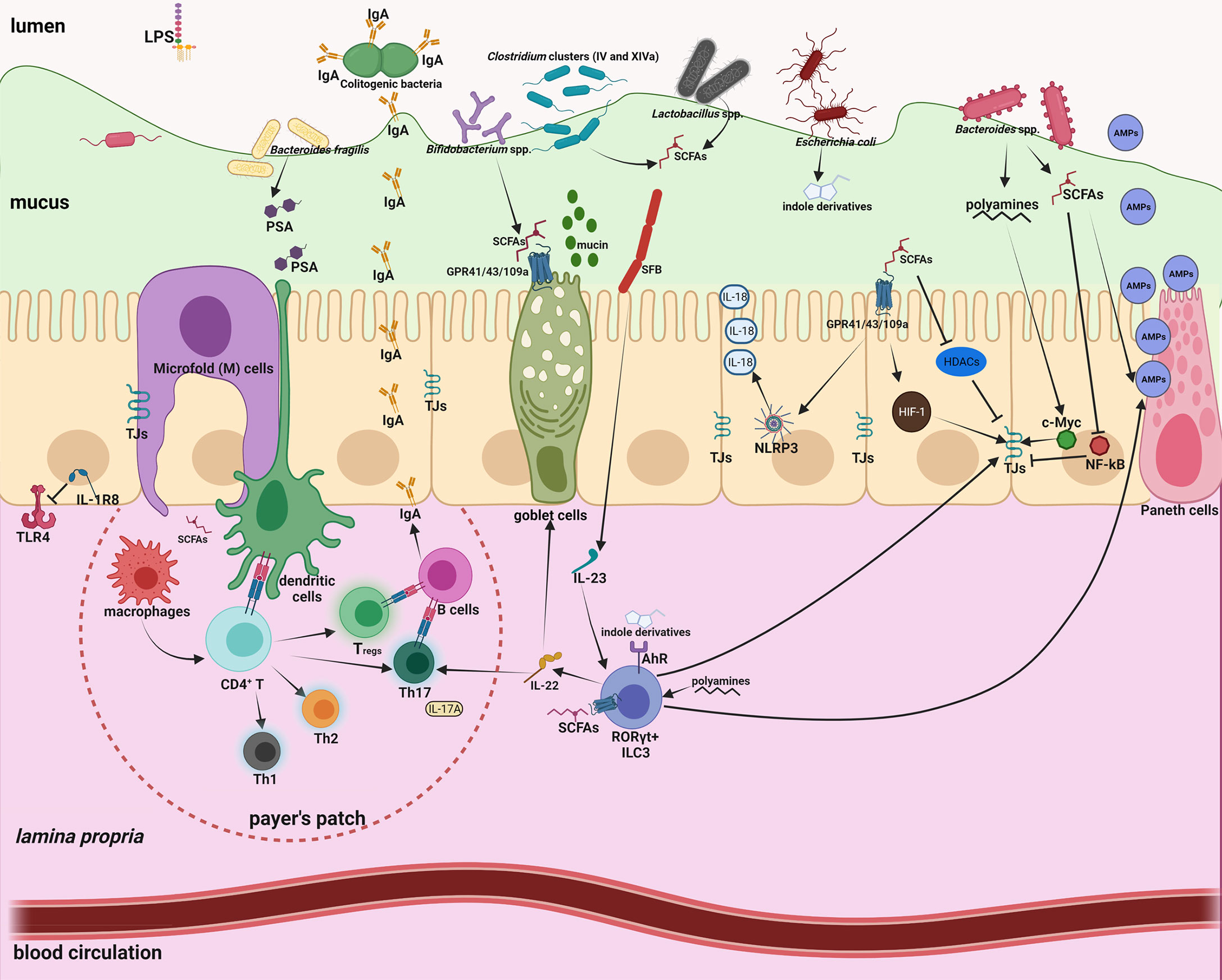
Figure 1 Intestinal homeostasis is tightly controlled by gut microbiota through a large number of microbial metabolites/components. Intestinal mucus not only provides a habitat for bacterial colonization but also serves as a lubricant barrier to restrict most gut microbes in the outer layer. Microfold (M) cells above the Peyer’s patch are essential to transport microbiota-derived metabolites/components to maintain the homeostasis of the mucosal immune system. 1) The effects of short-chain-fatty-acids (SCFAs) are manifold, including enhanced mucus production; inhibition of nuclear factor-κB (NF-κB); activation of NLR-family-pyrin-domain-containing-3 (NLRP3) inflammasomes and subsequent production of interleukin-18 (IL-18); enhanced antimicrobial peptide (AMP) production; polarization of anti-inflammatory macrophages; increased Immunoglobulin A (IgA) secretion; reduced expression of T cell-activating molecules on antigen-presenting cells; and increased number and function of colonic regulatory T (Tregs) cells. 2) Polyamines can activate RORγt+ group 3 innate lymphoid cells (ILC3) and induce production of IL-22, which promote mucus and AMP secretion, and ensure commensal compartmentalization from the intestinal epithelium. 3) Indole derivatives produced by gut commensals can stimulate Aryl-Hydrocarbon-Receptor (AhR) to activate ILC3 and fortify the epithelium barrier function. 4) Polysaccharide A (PSA) from Bacteroides fragilis is taken up by DCs, processed and presented to naive CD4+ T cells, inducing the expansion of FOXP3+ Treg cells. 5) Attachment of segmented filamentous bacteria (SFB) to the epithelium enhances differentiation and expansion of CD4+ Th17 cells. Foxp3+ Treg cells and Th17 cells localize in the Peyer’s patches, and induce B cell class-switch and IgA production, which in turn remodels microbiota. 6) Basolateral location of the LPS receptor TLR4 on IECs and expression of the anti-inflammatory IL-1R8 allow proper immune tolerance.
Regulation of Innate Immunity
As a physical barrier at the intestinal surface, IECs are equipped with an array of immune receptors to sense and integrate microbiota-derived metabolites and components for maintaining immune homeostasis. By activating G-protein-coupled-receptors (GPR41, GPR43, GPR109A) on IECs, SCFAs can promote the activation of the NOD-like-receptor-protein 3 (NLRP3) inflammasome, inducing production of the homeostatic cytokine interleukin-18 (IL-18) (42). SCFAs can also stimulate goblet cell differentiation, mucin gene transcription and mucus secretion (43). Pattern recognition receptors (PRRs) on the IEC surface, such as Toll-like receptors (TLRs), can sense microbial antigens. Notably, a number of homeostatic mechanisms ensure immune tolerance towards commensals, such as the basolateral location of the LPS receptor TLR4 that allows binding and activation only to invading bacteria (44) and the constitutive expression of the anti-inflammatory IL-1R8, which binds to and inhibits TLR and IL-1 receptors (45).
Intriguingly, the commensal gut microbiota also interacts with IECs to maintain an effective gut barrier. SCFAs, particularly butyrate, have crucial roles in regulating tight junction (TJ) proteins via multifaceted signaling pathways (46), such as HIF-1 stabilization (47), and histone deacetylase (HDAC) inhibition (48). By contrast pathogenic E. coli Shiga-toxins and LPS (49) could compromise the epithelial barrier by disrupting TJ. LPS increases intestinal epithelium permeability through the TLR4/MyD88/TGF-β activated kinase 1 (TAK1)/nuclear-factor-κB (NF-κB) cascade in both in vitro and in vivo models (50).
Immunoregulation of gut microbiota also covers innate lymphoid cells (ILCs), a subpopulation of innate cells (natural killer cells, ILC1, ILC2, ILC3) specialized in recognizing and reacting to infectious challenges. SCFAs can modulate ILC3 proliferation and stimulate IL-22 production in an AKT/STAT3-dependent manner. IL-22 promotes antimicrobial peptide (AMP) production, mucin secretion and colonization of commensal microbes (51).
Intestinal resident macrophages maintain the tissue homeostasis by removing senescent and anomalous cells, and contribute to tissue defense by eliminating invading pathogens and foreign objects. Upon binding to TLR4, LPS can promote inflammatory macrophage activation (M1 polarization), with the production of an array of inflammatory cytokines, IL-1β, IL-6, IL-12 and tumor necrosis factor-α (TNF-α) (52). Conversely, SCFA butyrate facilitates the anti-inflammatory/tissue-healing macrophage polarization, probably by activation of the H3K9/STAT6 signaling pathway (53).
Regulation of Adaptive Immunity
The impact of gut microbiota goes beyond the innate immunity, through its ability to affect the activation of antigen-presenting cells (APCs), which are the link between innate and adaptive immunity. APCs in the gut encompass resident DCs and tissue macrophages, which are involved in antigen presentation to naïve and primed T cells. Activation, maturation and functionality of DCs and macrophages can be influenced by LPS and SCFAs. As the major APCs in the intestine (54), macrophages can be regulated by microbial niacin and butyrate via activating GPR109A, which in turn increases production of anti-inflammatory IL-10 and Aldehyde-Dehydrogenase-1-Family-Member-A1 (ALDH1A), and induces differentiation of T cells (55). LPS is a potent elicitor of DC migration and maturation by activating mitogen-activated protein kinase (MAPK) and NF-κB signaling pathways (56). SCFAs can block the DC generation from bone marrow stem cells (57), and down-regulate expression of the T cell-stimulatory proteins CD80, CD83 and major-histocompatibility-complex class II (MHCII) (58).
Through its effects on APCs that produce several cytokines necessary for T cell activation, the gut microbiota is also involved in differentiation of naïve CD4+ T cells into defined subsets, including T helper (Th1, Th2 and Th17) and regulatory T cells (Tregs). Inhibition of HDAC by SCFAs can regulate the mTOR–S6K pathway required for generation of Th17, Th1, and IL-10+ T cells (59). Tregs have important anti-inflammatory roles, allowing the immune system to tolerate antigens derived from gut microbiota and diet. Through binding to GPR43, SCFAs can stimulate Tregs proliferation (60). Additionally, SCFAs control the expression of genes necessary for plasma B cell differentiation and Immunoglobulin A (IgA) production (61). As the largest class of immunoglobulins in the intestinal mucosa, IgA targets microbial antigens and preferentially coats colitogenic bacteria, therefore preventing inflammation and perturbation of intestinal homeostasis (62).
Numerous ENMs Could Reshape the Gut Microbiota Signature but It Is Not a General Effect
ENMs might interact with gut microbes in different manners (Figure 2). Of special concern is the intrinsic antimicrobial potency of some ENMs. Nanoparticulate Au, Ag, TiO2 and ZnO can exert bactericidal activity by disrupting the bacterial membrane (63, 64), inducing intracellular reactive oxygen species (64, 65) and causing direct genotoxicity (66). Conversely, iron oxide and graphene ENMs can promote the growth of some bacterial species, with mechanisms still largely unknown (67, 68). Adding to the complexity is that many gut microbes could rapidly develop strategies to resist ENM bactericidal actions (69). Gram-negative bacteria are thought to be more tolerant to ENMs, in that a lower amount of the negatively charged peptidoglycan may be less effective in trapping the positively-charged metal ions, while other studies argue that Gram-positive bacteria have thicker membranes to ensure stronger protection (69, 70). The resistance mechanism to ENMs could be specific at the bacterial species/strain level. The gut microbiota remodeling effect of ENMs has been substantiated by a panel of in vivo assays. For instance, dietary Ag ENMs for mice decreased Odoribacteraceae, Bacteroidaceae and the S24-7 family while increasing Lactobacillaceae and Lachnospiraceae (17). Oral gavage of TiO2 ENMs in mice also modulated the gut microbiota, with Bacteroides and Akkermansia increased (22). Oral administration of non-metallic single-walled carbon nanotubes (SWCNTs) modestly altered the α- and β-diversity of the mouse microbiome (27). The modulation of animal gut microbiota by other ENMs is systemically summarized in recent reviews, which include nanoparticulate plastics, graphene oxide, multi-walled carbon nanotubes (MWCNT), SWCNT, Ag, ZnO, MoO3, MoS2, TiO2, CuO and SiO2 (5, 71, 72). Numerous in vitro assays also validate the ENM modulatory effect on gut microbiota samples (68, 73–75). Importantly, there appears to be no consensus effect, as multiple ENM-related factors (dose, physicochemical nature, particle size, surface charge, shape and stability) might dictate their modulatory mechanisms and efficacy (64, 76). In addition, the gut microbiota signature varies among individuals, and even within the same subject it changes with time, food intake and health conditions (77–79).
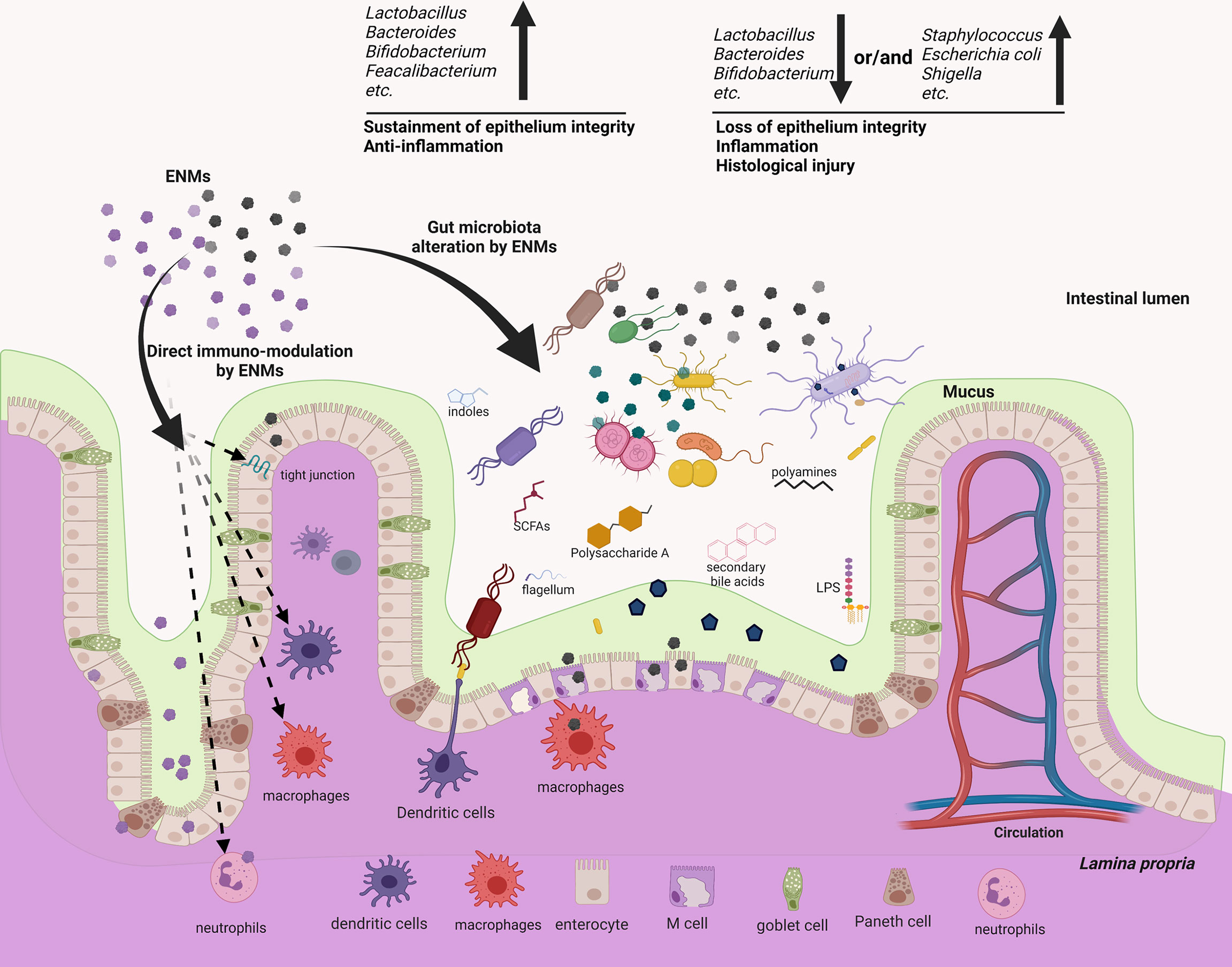
Figure 2 ENMs could not only modulate several components of the mucosal immune systems directly, but also reshape the gut microbiota, which may potentially act as an alternative but important regulator to mediate the immuno-modulatory effects of ENMs. ENMs could accumulate and directly interact with neutrophils, macrophages, dendritic cells (DCs) and the complement system to modulate innate/inflammatory immune responses. On the other hand, several metallic and non-metallic ENMs are proved to be bactericidal, either impairing the bacterial membrane, or causing intracellular oxidative stress, or generating genotoxicity. As responses to the ENM bactericidal effects, members of gut microbiota may rapidly develop resistance, but the associated molecular strategies and efficacy often differ among distinct members. Many in vitro and in vivo assays showed that ENMs can alter the gut microbiota profile, enrich the relative abundance of pathogens or decrease that of gut commensals. This effect often associates with intestinal inflammation and tissue injury. While some ENMs could increase gut commensals, which in turn exert anti-inflammatory effects. Conversely, a few works show that the gut microbiota remains resilient following oral exposure to ENMs, indicating that the ENM effect on gut microbiota/mucosal immunity is not general.
One may suspect the modulatory effect of ENMs on gut microbiota. Because the link between immunity and microbiota is bidirectional (32), could it be possible that ENMs affect immunity and as a consequence the microbiota? Indeed, ENMs could accumulate in the intestine, favor inflammatory responses and impair the barrier function, including IEC apoptosis, tight junction opening, decreased AMP production, Th1/Tregs imbalance, aberrant IgA secretion and inflammatory activation of macrophages (72). In this situation, the gut microbiota can be in turn altered by the mucosal immunity. But, a considerable number of ENMs (Ag, SWCNT, CuO, TiO2) are shown to alter the gut microbiota without inducing any detectable changes in intestinal immunity (17, 22, 27, 29). These data suggest that ENMs may cooperate with the mucosal immunity to modulate the gut microbiota.
The Potential Effect Of ENM-Altered Microbiota on Intestinal Inflammation
As discussed above, microbiota-derived metabolites such as SCFAs have important roles in the regulation of gut immunity (Figure 1), while ENM exposure that reduces SCFA-producing bacteria may perturb the immune homeostasis and cause inflammation (Table 1). Indeed, gut microbiota dysbiosis appears to tightly associate with inflammatory bowel disease (IBD), a chronic and relapsing inflammatory disorder of the intestine (80). This link has been observed in several in vivo assays that model GIT exposure to ENMs. Oral administration of Ag ENM (2.5 mg/kg body weight daily) in mice profoundly reduced the Firmicutes to Bacteroidetes ratio, specifically due to an increase in Alistipes, Bacteroides and Prevotella, and a significant decrease in SCFA-producing Lactobacillus. The altered microbiota could cause some IBD-like symptoms, including disrupted epithelium structure, increased intestinal permeability and upregulation of inflammatory cytokines (IL-1β, IL-6 and TNF-α) (18). In the same study, oral gavage of TiO2 ENMs (2.5 mg/kg body weight daily) significantly decreased the probiotic Bacteroides and triggered a low-grade colonic inflammation (18). Likewise, administration of SWCNT, MWCNT and graphene oxide ENMs (2.5 mg/kg body weight daily) in mice disrupted the gut microbiota signature, with commensal Lactobacillus and Bacteroides decreased. The exposed mice displayed tissue injury, increased intestinal permeability and elevated production of inflammatory IL-1β, IL-6, and TNF-α (26).
Moreover, enrichment of pathogens and associated virulence factors following ENM administration could also cause intestinal inflammation (81). The work of Chen et al. showed that oral administration of TiO2 ENMs (50 mg/kg body weight) in rats decreased the number of goblet cells, elicited immune cell infiltration and mitochondrial abnormalities in the colon tissues, suggesting redox imbalance and inflammation. TiO2 ENM treatment remarkably affected the fecal metabolite profile, and particularly enriched the LPS content (23). In another work, oral gavage of TiO2 ENM (100 mg/kg body weight daily) in mice impaired the intestinal microvilli structure, and increased Escherichia and Shigella, two potential pathogens for elicitation of intestinal inflammation (22). Dietary nanoplastics (600 μg daily) for mice significantly increased pathogenic Staphylococcus abundance alongside a decrease in Parabacteroides (28). The ENM-feeding group displayed a chronic intestinal inflammation, such as increased serum IL-1α, abnormal ratio of Th17 and Tregs among CD4+ cells, infiltration of lymphocytes and plasma B cells in the lamina propria, and higher expression of inflammatory markers (TLR4, AP-1, and IRF5) (28). In these cases, ENMs may enrich opportunistic pathogens or liberate the membrane-bound PAMPs from bacterial cells (82). The inflammatory antigens, such as LPS, exotoxin and flagellin, would bind to PRRs on IECs and immune cells, thus activating inflammatory pathways and promoting an excessive intestinal inflammation (83–86). However, most of these in vivo studies based on animal models rarely simulated the realistic human exposure condition. Table 1 details such shortcomings: either subjects were exposed to an excessive dose of ENMs, or ENMs were administered alone without food which is not a real-life fashion. Whether ENMs were contaminated by LPS was not checked, either.
There is no general effect of ENMs on gut microbiota and intestinal immunity. Contrary to the aforementioned adverse effects, other studies showed that ENM ingestion can increase commensal microbes and exert anti-inflammatory effects. Dietary ZnO ENMs (600 mg/kg food) for weaned piglets increased Lactobacillus, leading to upregulation of tight junction proteins and antioxidant enzymes, and decreased expression of inflammatory interferon-γ (IFN-γ), IL-1β, TNF-α and NF-κB (25). Similarly, oral gavage of Ag ENMs (5ng daily) attenuated the dextran sodium sulfate (DSS)-induced IBD symptoms in mice, probably by increasing Lactobacillus and decreasing Clostridium perfringens and Escherichia coli (19). Enrichment of Lactobacillus was found in these works, again highlighting the protective role of SCFA-producers in epithelium integrity and anti-inflammatory responses.
Strikingly, several studies found no significant effect of ENMs (Au, CuO, Ag and lysine-modified SWCNTs) on intestinal immunity (17, 20, 21, 27, 29, 87). One possibility is that most ENMs may be rapidly excreted following ingestion, so few accumulate in the GIT and they are insufficient to modulate the immune responses. Indeed, 270-day consecutive dietary supplementation with ZnO ENMs (1600 mg/kg food) for mice revealed no detectable ENM distribution in the GIT (88). Hence, this work indicates that there is no general effect regarding the biodistribution and accumulation, it should be specific to each ENM. Additionally, the mucus layer that is mainly composed of highly-glycosylated secreted proteins overlying the intestinal epithelium could trap ENMs and minimize their contact with gut microbes and mucosal cells (8). This can explain why the modulatory effect of ENMs on gut microbes in vitro is always greater than that in vivo. When the earthworms were exposed to soil with CuO or Ag ENMs, the gut microbiota remained largely resilient, whereas both ENMs significantly changed the soil bacterial community composition (89). Moreover, though some ENMs can modify the gut microbiota, members of the core commensal consortium are not affected; or the roles of redundant symbionts affected by ENMs could be compensated by other unchanged commensals. For example, exposure of earthworms to soil supplemented with CuO ENMs (160 mg/kg) induced substantial changes in the gut microbiota with a significant decrease in the symbiont Candidatus Lumbricincola, but it had no effect on the immune competence (29). Thereby, the gut microbiota might adapt itself in a way (which needs to be demonstrated) that ensures maintaining a proper immune homeostasis.
Conclusions and Perspectives
To summarize, increasing observations have claimed a link between GIT exposure to ENMs, gut microbiota dysbiosis and intestinal inflammation (Figure 2). Such effects of ENMs are often dose-dependent. We acknowledge that in a few cases ENMs could induce microbiota dysbiosis characterized by a decrease in commensals (Lactobacillus, Bacteroides, Bifidobacterium, etc.) and/or an enrichment of other members (E. coli, Shigella, Listeria, etc.), which in turn cause an intestinal inflammation, compromise epithelium integrity and induce IBD-like symptoms (Figure 2). But these works suffer shortcomings and are not relevant for human exposure doses or uptake conditions. By contrast, little or no overt effect on intestinal immunity has been found in a large number of in vivo assays, where ENMs are orally administered in a more realistic dose or fashion. Notably, most in vivo studies investigate the immunotoxicity of ENMs in healthy individuals, while it might be more prominent in those with intestinal inflammation (such as IBD). Indeed, inflammatory symptoms like mucus defects (90), dysfunctional macrophages (91), etc. could increase and extend the exposure of intestinal epithelium to ENMs. Interestingly, the DSS-induced IBD symptoms in mice can be either exacerbated (92) or attenuated (19) following oral intake of ENMs, suggesting that ENM exposure do not necessarily have detrimental consequences, even for those with inflamed intestine. Future works should cover more types of ENMs, simulate the real-life ENM exposure situation, exploit both healthy and inflammatory host model, and draw cautious conclusions.
The in vivo studies on different animal models show extensive variation regarding the ENM effects on gut microbiota or intestinal immunity (Table 1). This may be due to discrepancies in the overall experimental settings (animal species, age, EMN exposure time, dose and uptake manner), the ENM physicochemical nature (size, shape, surface decoration and charge), the possible in vivo bio-transformation of ENMs and the methodology for gut microbiota analysis (Table 1). A unifying exposure model is required.
However, pitfalls of current animal models should be considered when translating gut microbiota research results to humans. The murine gut microbiota resembles the human one at phylum level, but differs at genus and species level (93). The anatomy and physiological functions of several GIT segments in the mouse are also different from those of humans (93). Therefore, a future perspective is to establish human models, necessarily in vitro, based on primary cells. To this end, microfluidic intestine-on-chips that can establish a prolonged coculture of human intestinal epithelium and gut microbes could be a promising in vitro human model to evaluate the ENM immunotoxicity (94, 95). When supplemented with immune cells, the intestine-on-a-chip could enable us to monitor the dynamics of ENM behavior in the gut tissue, gut microbiota changes, intestinal barrier function, immune cell activation and inflammation, thus providing predictive values on the ENM immunotoxicity. An additional but important point is the variability of gut microbiota, not only inter-individually but also at the intra-individual level (for instance in different health conditions). This calls for the need of a personalized profiling of the ENM effects on gut immunity, as it will depend on the individual microbiota in a given moment. Future immuno-nanosafety models, like the intestine-on-a-chip mentioned above, will therefore need to include the individual microbiota and the innate immune cells (in particular macrophages) derived from the individual subject.
Author Contributions
MT, JQ, and LL devised the study and wrote the manuscript. SL, LW, and ZH contributed to literature search and gave insightful suggestions in revising this work. All authors contributed to the article and approved the submitted version.
Funding
This work was supported by the National Natural Science Foundation of China (81900071).
Conflict of Interest
The authors declare that the research was conducted in the absence of any commercial or financial relationships that could be construed as a potential conflict of interest.
The Editor declared a shared affiliation with some of the authors MT, LW, JQ, LL at the time of review.
Publisher’s Note
All claims expressed in this article are solely those of the authors and do not necessarily represent those of their affiliated organizations, or those of the publisher, the editors and the reviewers. Any product that may be evaluated in this article, or claim that may be made by its manufacturer, is not guaranteed or endorsed by the publisher.
Acknowledgments
BioRender was used to create schematic representations.
References
1. De Jong WH, Borm PJA. Drug Delivery and Nanoparticles: Applications and Hazards. Int J Nanomed (2008) 3(2):133–49. doi: 10.2147/ijn.s596
2. Ge L, Li Q, Wang M, Ouyang J, Li X, Xing MMQ. Nanosilver Particles in Medical Applications: Synthesis, Performance, and Toxicity. Int J Nanomed (2014) 9:2399–407. doi: 10.2147/IJN.S55015
3. Weir A, Westerhoff P, Fabricius L, Hristovski K, Von Goetz N. Titanium Dioxide Nanoparticles in Food and Personal Care Products. Environ Sci Technol (2012) 46(4):2242–50. doi: 10.1021/es204168d
4. Vance ME, Kuiken T, Vejerano EP, Mcginnis SP, Hochella MF Jr., Rejeski D, et al. Nanotechnology in the Real World: Redeveloping the Nanomaterial Consumer Products Inventory. Beilstein J Nanotechnol (2015) 6:1769–80. doi: 10.3762/bjnano.6.181
5. Hirt N, Body-Malapel M. Immunotoxicity and Intestinal Effects of Nano- and Microplastics: A Review of the Literature. Part Fibre Toxicol (2020) 17(1):57. doi: 10.1186/s12989-020-00387-7
6. Carrouel F, Viennot S, Ottolenghi L, Gaillard C, Bourgeois D. Nanoparticles as Anti-Microbial, Anti-Inflammatory, and Remineralizing Agents in Oral Care Cosmetics: A Review of the Current Situation. Nanomater (Basel Switzerland) (2020) 10(1):140. doi: 10.3390/nano10010140
7. Park E-J, Yi J, Kim Y, Choi K, Park K. Silver Nanoparticles Induce Cytotoxicity by a Trojan-Horse Type Mechanism. Toxicol In Vitro (2010) 24(3):872–8. doi: 10.1016/j.tiv.2009.12.001
8. Georgantzopoulou A, Serchi T, Cambier S, Leclercq CC, Renaut J, Shao J, et al. Effects of Silver Nanoparticles and Ions on a Co-Culture Model for the Gastrointestinal Epithelium. Part Fibre Toxicol (2016) 13(1):9. doi: 10.1186/s12989-016-0117-9
9. Mortensen NP, Moreno Caffaro M, Aravamudhan S, Beeravalli L, Prattipati S, Snyder RW, et al. Simulated Gastric Digestion and In Vivo Intestinal Uptake of Orally Administered CuO Nanoparticles and TiO2 E171 in Male and Female Rat Pups. Nanomater (Basel Switzerland) (2021) 11(6):1487. doi: 10.3390/nano11061487
10. Zolnik BS, GonzíLez-FerníNdez AF, Sadrieh N, Dobrovolskaia MA. Minireview: Nanoparticles and the Immune System. Endocrinology (2010) 151(2):458–65. doi: 10.1210/en.2009-1082
11. Boraschi D, Italiani P, Palomba R, Decuzzi P, Duschl A, Fadeel B, et al. Nanoparticles and Innate Immunity: New Perspectives on Host Defence. Semin Immunol (2017) 34:33–51. doi: 10.1016/j.smim.2017.08.013
12. Dobrovolskaia MA, Shurin M, Shvedova AA. Current Understanding of Interactions Between Nanoparticles and the Immune System. Toxicol Appl Pharmacol (2016) 299:78–89. doi: 10.1016/j.taap.2015.12.022
13. David CA, Owen A, Liptrott NJ. Determining the Relationship Between Nanoparticle Characteristics and Immunotoxicity: Key Challenges and Approaches. Nanomedicine (2016) 11(11):1447–64. doi: 10.2217/nnm-2016-0017
14. Tlaskalova-Hogenova H, Vetvicka V, Sterzl J, Stepankova R. Development of Immune Potential and Migration Pattern of Cells From Germfree (Gf) and Conventionally (Conv) Reared Rats. Adv Exp Med Biol (1982) 149:515–20. doi: 10.1007/978-1-4684-9066-4_72
15. Li Y, Fujita M, Boraschi D. Endotoxin Contamination in Nanomaterials Leads to the Misinterpretation of Immunosafety Results. Front Immunol (2017) 8:472. doi: 10.3389/fimmu.2017.00472
16. Li Y, Shi Z, Radauer-Preiml I, Andosch A, Casals E, Luetz-Meindl U, et al. Bacterial Endotoxin (Lipopolysaccharide) Binds to the Surface of Gold Nanoparticles, Interferes With Biocorona Formation and Induces Human Monocyte Inflammatory Activation. Nanotoxicology (2017) 11(9-10):1157–75. doi: 10.1080/17435390.2017.1401142
17. Van Den Brule S, Ambroise J, Lecloux H, Levard C, Soulas R, De Temmerman PJ, et al. Dietary Silver Nanoparticles Can Disturb the Gut Microbiota in Mice. Part Fibre Toxicol (2016) 13(1):38. doi: 10.1186/s12989-016-0149-1
18. Chen H, Zhao R, Wang B, Cai C, Zheng L, Wang H, et al. The Effects of Orally Administered Ag, TiO2 and SiO2 Nanoparticles on Gut Microbiota Composition and Colitis Induction in Mice. NanoImpact (2017) 8:80–8. doi: 10.1016/j.impact.2017.07.005
19. Siczek K, Zatorski H, Chmielowiec-Korzeniowska A, Pulit-Prociak J, Smiech M, Kordek R, et al. Synthesis and Evaluation of Anti-Inflammatory Properties of Silver Nanoparticle Suspensions in Experimental Colitis in Mice. Chem Biol Drug Des (2017) 89(4):538–47. doi: 10.1111/cbdd.12876
20. Hadrup N, Loeschner K, Bergstrom A, Wilcks A, Gao X, Vogel U, et al. Subacute Oral Toxicity Investigation of Nanoparticulate and Ionic Silver in Rats. Arch Toxicol (2012) 86(4):543–51. doi: 10.1007/s00204-011-0759-1
21. Wilding LA, Bassis CM, Walacavage K, Hashway S, Leroueil PR, Morishita M, et al. Repeated Dose (28-Day) Administration of Silver Nanoparticles of Varied Size and Coating Does Not Significantly Alter the Indigenous Murine Gut Microbiome. Nanotoxicology (2016) 10(5):513–20. doi: 10.3109/17435390.2015.1078854
22. Li J, Yang S, Lei R, Gu W, Qin Y, Ma S, et al. Oral Administration of Rutile and Anatase Tio2 Nanoparticles Shifts Mouse Gut Microbiota Structure. Nanoscale (2018) 10(16):7736–45. doi: 10.1039/c8nr00386f
23. Chen Z, Han S, Zhou D, Zhou S, Jia G. Effects of Oral Exposure to Titanium Dioxide Nanoparticles on Gut Microbiota and Gut-Associated Metabolism in Vivo. Nanoscale (2019) 11(46):22398–412. doi: 10.1039/c9nr07580a
24. Pei X, Xiao Z, Liu L, Wang G, Tao W, Wang M, et al. Effects of Dietary Zinc Oxide Nanoparticles Supplementation on Growth Performance, Zinc Status, Intestinal Morphology, Microflora Population, and Immune Response in Weaned Pigs. J Sci Food Agric (2019) 99(3):1366–74. doi: 10.1002/jsfa.9312
25. Xia T, Lai W, Han M, Han M, Ma X, Zhang L. Dietary Zno Nanoparticles Alters Intestinal Microbiota and Inflammation Response in Weaned Piglets. Oncotarget (2017) 8(39):64878–91. doi: 10.18632/oncotarget.17612
26. Chen H, Zhao R, Wang B, Zheng L, Ouyang H, Wang H, et al. Acute Oral Administration of Single-Walled Carbon Nanotubes Increases Intestinal Permeability and Inflammatory Responses: Association With the Changes in Gut Microbiota in Mice. Adv Healthc (2018) 7(13):1701313. doi: 10.1002/adhm.201701313
27. Mulvey JJ, Littmann ER, Ling L, Mcdevitt MR, Pamer EG, Scheinberg DA. The Effects of Amine-Modified Single-Walled Carbon Nanotubes on the Mouse Microbiota. Int J Nanomed (2018) 13:5275–86. doi: 10.2147/IJN.S168554
28. Li B, Ding Y, Cheng X, Sheng D, Xu Z, Rong Q, et al. Polyethylene Microplastics Affect the Distribution of Gut Microbiota and Inflammation Development in Mice. Chemosphere (2020) 244:125492. doi: 10.1016/j.chemosphere.2019.125492
29. Swart E, Dvorak J, Hernádi S, Goodall T, Kille P, Spurgeon D, et al. The Effects of in Vivo Exposure to Copper Oxide Nanoparticles on the Gut Microbiome, Host Immunity, and Susceptibility to a Bacterial Infection in Earthworms. Nanomater (Basel) (2020) 10(7):1337. doi: 10.3390/nano10071337
30. Kamada N, Kim Y-G, Sham HP, Vallance BA, Puente JL, Martens EC, et al. Regulated Virulence Controls the Ability of a Pathogen to Compete With the Gut Microbiota. Science (2012) 336(6086):1325–9. doi: 10.1126/science.1222195
31. Donohoe DR, Garge N, Zhang X, Sun W, O’connell TM, Bunger MK, et al. The Microbiome and Butyrate Regulate Energy Metabolism and Autophagy in the Mammalian Colon. Cell Metab (2011) 13(5):517–26. doi: 10.1016/j.cmet.2011.02.018
32. Zheng D, Liwinski T, Elinav E. Interaction Between Microbiota and Immunity in Health and Disease. Cell Res (2020) 30(6):492–506. doi: 10.1038/s41422-020-0332-7
33. Belkaid Y, Harrison OJ. Homeostatic Immunity and the Microbiota. Immunity (2017) 46(4):562–76. doi: 10.1016/j.immuni.2017.04.008
34. Albhaisi SM, Bajaj JS, Sanyal AJ. Role of Gut Microbiota in Liver Disease. Am J Physiol Gastrointest Liver Physiol (2020) 318(1):84–98. doi: 10.1152/ajpgi.00118.2019
35. Morais LH, Schreiber HL, Mazmanian SK. The Gut Microbiota–Brain Axis in Behaviour and Brain Disorders. Nat Rev Microbiol (2021) 19(4):241–55. doi: 10.1038/s41579-020-00460-0
36. Rooks MG, Garrett WS. Gut Microbiota, Metabolites and Host Immunity. Nat Rev Immunol (2016) 16(6):341–52. doi: 10.1038/nri.2016.42
37. Wang G, Huang S, Wang Y, Cai S, Yu H, Liu H, et al. Bridging Intestinal Immunity and Gut Microbiota by Metabolites. Cell Mol Life Sci (2019) 76(20):3917–37. doi: 10.1007/s00018-019-03190-6
38. Thaiss CA, Zmora N, Levy M, Elinav E. The Microbiome and Innate Immunity. Nature (2016) 535(7610):65–74. doi: 10.1038/nature18847
39. Honda K, Littman DR. The Microbiota in Adaptive Immune Homeostasis and Disease. Nature (2016) 535(7610):75–84. doi: 10.1038/nature18848
40. Den Besten G, Van Eunen K, Groen AK, Venema K, Reijngoud D-J, Bakker BM. The Role of Short-Chain Fatty Acids in the Interplay Between Diet, Gut Microbiota, and Host Energy Metabolism. J Lipid Res (2013) 54(9):2325–40. doi: 10.1194/jlr.R036012
41. Heine H, Rietschel ET, Ulmer AJ. The Biology of Endotoxin. Mol Biotechnol (2001) 19(3):279–96. doi: 10.1385/MB:19:3:279
42. Macia L, Tan J, Vieira AT, Leach K, Stanley D, Luong S, et al. Metabolite-Sensing Receptors Gpr43 and Gpr109a Facilitate Dietary Fibre-Induced Gut Homeostasis Through Regulation of the Inflammasome. Nat Commun (2015) 6(1):6734. doi: 10.1038/ncomms7734
43. Wrzosek L, Miquel S, Noordine M-L, Bouet S, Chevalier-Curt MJ, Robert V, et al. Bacteroides Thetaiotaomicron and Faecalibacterium Prausnitzii Influence the Production of Mucus Glycans and the Development of Goblet Cells in the Colonic Epithelium of a Gnotobiotic Model Rodent. BMC Biol (2013) 11(1):61. doi: 10.1186/1741-7007-11-61
44. Fusunyan RD, Nanthakumar NN, Baldeon ME, Walker WA. Evidence for an Innate Immune Response in the Immature Human Intestine: Toll-Like Receptors on Fetal Enterocytes. Pediatr Res (2001) 49(4):589–93. doi: 10.1203/00006450-200104000-00023
45. Molgora M, Barajon I, Mantovani A, Garlanda C. Regulatory Role of Il-1r8 in Immunity and Disease. Front Immunol (2016) 7:149. doi: 10.3389/fimmu.2016.00149
46. Parada Venegas D, de la Fuente MK, Landskron G, González MJ, Quera R, Dijkstra G, et al. Short Chain Fatty Acids (Scfas)-Mediated Gut Epithelial and Immune Regulation and Its Relevance for Inflammatory Bowel Diseases. Front Immunol (2019) 10:277. doi: 10.3389/fimmu.2019.00277
47. Fachi JL, Felipe JDS, Pral LP, Da Silva BK, Corrêa RO, De Andrade MCP, et al. Butyrate Protects Mice From Clostridium Difficile-Induced Colitis Through an Hif-1-Dependent Mechanism. Cell Rep (2019) 27(3):750–61.e7. doi: 10.1016/j.celrep.2019.03.054
48. Moore R, Pothoulakis C, Lamont JT, Carlson S, Madara JL. C. Difficile Toxin a Increases Intestinal Permeability and Induces Cl- Secretion. Am J Physiol Gastrointest Liver Physiol (1990) 259(2):G165–G72. doi: 10.1152/ajpgi.1990.259.2.G165
49. Karve SS, Pradhan S, Ward DV, Weiss AA. Intestinal Organoids Model Human Responses to Infection by Commensal and Shiga Toxin Producing Escherichia Coli. PloS One (2017) 12(6):e0178966. doi: 10.1371/journal.pone.0178966
50. Guo S, Al-Sadi R, Said HM, Ma TY. Lipopolysaccharide Causes an Increase in Intestinal Tight Junction Permeability In Vitro and In Vivo by Inducing Enterocyte Membrane Expression and Localization of Tlr-4 and Cd14. Am J Pathol (2013) 182(2):375–87. doi: 10.1016/j.ajpath.2012.10.014
51. Qiu J, Heller JJ, Guo X, Chen Z-ME, Fish K, Fu Y-X, et al. The Aryl Hydrocarbon Receptor Regulates Gut Immunity Through Modulation of Innate Lymphoid Cells. Immunity (2012) 36(1):92–104. doi: 10.1016/j.immuni.2011.11.011
52. Martinez FO, Sica A, Mantovani A, Locati M. Macrophage Activation and Polarization. Front Biosci (2008) 13(2):453–61. doi: 10.2741/2692
53. Ji J, Shu D, Zheng M, Wang J, Luo C, Wang Y, et al. Microbial Metabolite Butyrate Facilitates M2 Macrophage Polarization and Function. Sci Rep (2016) 6(1):24838. doi: 10.1038/srep24838
54. Smith PD, Smythies LE, Shen R, Greenwell-Wild T, Gliozzi M, Wahl SM. Intestinal Macrophages and Response to Microbial Encroachment. Mucosal Immunol (2011) 4(1):31–42. doi: 10.1038/mi.2010.66
55. Singh N, Gurav A, Sivaprakasam S, Brady E, Padia R, Shi H, et al. Activation of Gpr109a, Receptor for Niacin and the Commensal Metabolite Butyrate, Suppresses Colonic Inflammation and Carcinogenesis. Immunity (2014) 40(1):128–39. doi: 10.1016/j.immuni.2013.12.007
56. Granucci F, Ferrero E, Foti M, Aggujaro D, Vettoretto K, Ricciardi-Castagnoli P. Early Events in Dendritic Cell Maturation Induced by Lps. Microbes Infect (1999) 1(13):1079–84. doi: 10.1016/S1286-4579(99)00209-9
57. Singh N, Thangaraju M, Prasad PD, Martin PM, Lambert NA, Boettger T, et al. Blockade of Dendritic Cell Development by Bacterial Fermentation Products Butyrate and Propionate Through a Transporter (Slc5a8)-Dependent Inhibition of Histone Deacetylases. J Biol Chem (2010) 285(36):27601–8. doi: 10.1074/jbc.M110.102947
58. Liu L, Li L, Min J, Wang J, Wu H, Zeng Y, et al. Butyrate Interferes With the Differentiation and Function of Human Monocyte-Derived Dendritic Cells. Cell Mol Immunol (2012) 277(1):66–73. doi: 10.1016/j.cellimm.2012.05.011
59. Park J, Kim M, Kang SG, Jannasch AH, Cooper B, Patterson J, et al. Short-Chain Fatty Acids Induce Both Effector and Regulatory T Cells by Suppression of Histone Deacetylases and Regulation of the Mtor–S6k Pathway. Mucosal Immunol (2015) 8(1):80–93. doi: 10.1038/mi.2014.44
60. Smith PM, Howitt MR, Panikov N, Michaud M, Gallini CA, Bohlooly --M, et al. The Microbial Metabolites, Short-Chain Fatty Acids, Regulate Colonic Treg Cell Homeostasis. Science (2013) 341(6145):569–73. doi: 10.1126/science.1241165
61. Kim M, Qie Y, Park J. And Kim Chang H. Gut Microbial Metabolites Fuel Host Antibody Responses. Cell Host Microbe (2016) 20(2):202–14. doi: 10.1016/j.chom.2016.07.001
62. Palm NW, De Zoete MR, Cullen TW, Barry NA, Stefanowski J, Hao L, et al. Immunoglobulin a Coating Identifies Colitogenic Bacteria in Inflammatory Bowel Disease. Cell (2014) 158(5):1000–10. doi: 10.1016/j.cell.2014.08.006
63. Smetana AB, Klabunde KJ, Marchin GR, Sorensen CM. Biocidal Activity of Nanocrystalline Silver Powders and Particles. Langmuir (2008) 24(14):7457–64. doi: 10.1021/la800091y
64. Guerrero Correa M, Martínez FB, Vidal CP, Streitt C, Escrig J, De Dicastillo CL. Antimicrobial Metal-Based Nanoparticles: A Review on Their Synthesis, Types and Antimicrobial Action. Beilstein J Nanotechnol (2020) 11:1450–69. doi: 10.3762/bjnano.11.129
65. Park H, Kim JY, Kim J, Lee J, Hahn J, Gu MB, et al. Silver-Ion-Mediated Reactive Oxygen Species Generation Affecting Bactericidal Activity. Water Res (2009) 43(4):1027–32. doi: 10.1016/j.watres.2008.12.002
66. Gulbagca F, Ozdemir S, Gulcan M, Sen F. Synthesis and Characterization of Rosa Canina-Mediated Biogenic Silver Nanoparticles for Anti-Oxidant, Antibacterial, Antifungal, and DNA Cleavage Activities. Heliyon (2019) 5(12):e02980. doi: 10.1016/j.heliyon.2019.e02980
67. Borcherding J, Baltrusaitis J, Chen H, Stebounova L, Wu C-M, Rubasinghege G, et al. Iron Oxide Nanoparticles Induce Pseudomonas Aeruginosa Growth, Induce Biofilm Formation, and Inhibit Antimicrobial Peptide Function. Environ Sci Nano (2014) 1(2):123–32. doi: 10.1039/C3EN00029J
68. Lahiani MH, Gokulan K, Williams K, Khare S. Impact of Pristine Graphene on Intestinal Microbiota Assessed Using a Bioreactor-Rotary Cell Culture System. ACS Appl Mater Interfaces (2019) 11(29):25708–19. doi: 10.1021/acsami.9b07635
69. Fröhlich EE, Fröhlich E. Cytotoxicity of Nanoparticles Contained in Food on Intestinal Cells and the Gut Microbiota. Int J Mol Sci (2016) 17(4):509. doi: 10.3390/ijms17040509
70. Xie Y, Wu B, Zhang X-X, Yin J, Mao L, Hu M. Influences of Graphene on Microbial Community and Antibiotic Resistance Genes in Mouse Gut as Determined by High-Throughput Sequencing. Chemosphere (2016) 144:1306–12. doi: 10.1016/j.chemosphere.2015.09.076
71. Zhang Y, Mortimer M, Guo L-H. Interplay Between Engineered Nanomaterials and Microbiota. Environ Sci Nano (2020) 7(9):2454–85. doi: 10.1039/D0EN00557F
72. Lamas B, Martins Breyner N, Houdeau E. Impacts of Foodborne Inorganic Nanoparticles on the Gut Microbiota-Immune Axis: Potential Consequences for Host Health. Part Fibre Toxicol (2020) 17(1):19. doi: 10.1186/s12989-020-00349-z
73. Das P, Mcdonald J, Petrof E, Allen-Vercoe E, Walker V. Nanosilver-Mediated Change in Human Intestinal Microbiota. J Nanomed Nanotechnol (2014) 5:235. doi: 10.4172/2157-7439.1000235
74. Cattò C, Garuglieri E, Borruso L, Erba D, Cristina M, Cappitelli F, et al. Impacts of Dietary Silver Nanoparticles and Probiotic Administration on the Microbiota of an in-Vitro Gut Model. Environ Pollut (2018) 245:754–63. doi: 10.1016/j.envpol.2018.11.019
75. Fondevila M, Herrer R, Casallas MC, Abecia L, Ducha JJ. Silver Nanoparticles as a Potential Antimicrobial Additive for Weaned Pigs. Anim Feed Sci Technol (2009) 150(3):259–69. doi: 10.1016/j.anifeedsci.2008.09.003
76. Marambio-Jones C, Hoek EMV. A Review of the Antibacterial Effects of Silver Nanomaterials and Potential Implications for Human Health and the Environment. J Nanopart Res (2010) 12(5):1531–51. doi: 10.1007/s11051-010-9900-y
77. Qin J, Li R, Raes J, Arumugam M, Burgdorf KS, Manichanh C, et al. A Human Gut Microbial Gene Catalogue Established by Metagenomic Sequencing. Nature (2010) 464(7285):59–65. doi: 10.1038/nature08821
78. Brooks AW, Priya S, Blekhman R, Bordenstein SR. Gut Microbiota Diversity Across Ethnicities in the United States. PloS Biol (2018) 16(12):e2006842–e. doi: 10.1371/journal.pbio.2006842
79. Fan Y, Pedersen O. Gut Microbiota in Human Metabolic Health and Disease. Nat Rev Microbiol (2021) 19(1):55–71. doi: 10.1038/s41579-020-0433-9
80. Shapiro JM, Subedi S, Leleiko NS. Inflammatory Bowel Disease. Pediatr Rev (2016) 37(8):337–47. doi: 10.1542/pir.2015-0110
81. Nishida A, Inoue R, Inatomi O, Bamba S, Naito Y, Andoh A. Gut Microbiota in the Pathogenesis of Inflammatory Bowel Disease. J Clin Gastroenterol (2018) 11(1):1–10. doi: 10.1007/s12328-017-0813-5
82. Lepper P, Held T, Schneider E, Bölke E, Gerlach H, Trautmann M. Clinical Implications of Antibiotic-Induced Endotoxin Release in Septic Shock. J Intensive Care Med (2002) 28(7):824–33. doi: 10.1007/s00134-002-1330-6
83. Neal MD, Leaphart C, Levy R, Prince J, Billiar TR, Watkins S, et al. Enterocyte Tlr4 Mediates Phagocytosis and Translocation of Bacteria Across the Intestinal Barrier. J Immunol (2006) 176(5):3070–9. doi: 10.4049/jimmunol.176.5.3070
84. Elena G, Giovanna D, Brunella P, De Anna F, Alessandro M, Antonietta TM. Proinflammatory Signal Transduction Pathway Induced by Shigella Flexneri Porins in Caco-2 Cells. Braz J Microbiol (2009) 40(3):701–13. doi: 10.1590/S1517-838220090003000036
85. Ray A, Biswas T. Porin of Shigella Dysenteriae Enhances Toll-Like Receptors 2 and 6 of Mouse Peritoneal B-2 Cells and Induces the Expression of Immunoglobulin M, Immunoglobulin G2a and Immunoglobulin A. Immunology (2005) 114(1):94–100. doi: 10.1111/j.1365-2567.2004.02002.x
86. Song WS, Jeon YJ, Namgung B, Hong M, Yoon S-I. A Conserved Tlr5 Binding and Activation Hot Spot on Flagellin. Sci Rep (2017) 7(1):40878. doi: 10.1038/srep40878
87. Li J, Cha R, Zhao X, Guo H, Luo H, Wang M, et al. Gold Nanoparticles Cure Bacterial Infection With Benefit to Intestinal Microflora. ACS Nano (2019) 13(5):5002–14. doi: 10.1021/acsnano.9b01002
88. Liu J-H, Ma X, Xu Y, Tang H, Yang S-T, Yang Y-F, et al. Low Toxicity and Accumulation of Zinc Oxide Nanoparticles in Mice After 270-Day Consecutive Dietary Supplementation. Toxicol Res (2017) 6:134–43. doi: 10.1039/c6tx00370b
89. Swart E, Goodall T, Kille P, Spurgeon DJ, Svendsen C. The Earthworm Microbiome Is Resilient to Exposure to Biocidal Metal Nanoparticles. Environ Pollut (2020) 267:115633. doi: 10.1016/j.envpol.2020.115633
90. Johansson MEV. Mucus Layers in Inflammatory Bowel Disease. Inflamm Bowel Dis (2014) 20(11):2124–31. doi: 10.1097/mib.0000000000000117
91. Na YR, Stakenborg M, Seok SH, Matteoli G. Macrophages in Intestinal Inflammation and Resolution: A Potential Therapeutic Target in Ibd. Nat Rev Gastroenterol Hepatol (2019) 16(9):531–43. doi: 10.1038/s41575-019-0172-4
92. Ogawa T, Okumura R, Nagano K, Minemura T, Izumi M, Motooka D, et al. Oral Intake of Silica Nanoparticles Exacerbates Intestinal Inflammation. Biochem Biophys Res Commun (2021) 534:540–6. doi: 10.1016/j.bbrc.2020.11.047
93. Nguyen TLA, Vieira-Silva S, Liston A, Raes J. How Informative Is the Mouse for Human Gut Microbiota Research? Dis Model Mech (2015) 8(1):1–16. doi: 10.1242/dmm.017400
94. Jalili-Firoozinezhad S, Gazzaniga FS, Calamari EL, Camacho DM, Fadel CW, Bein A, et al. A Complex Human Gut Microbiome Cultured in an Anaerobic Intestine-on-a-Chip. Nat BioMed Eng (2019) 3(7):520–31. doi: 10.1038/s41551-019-0397-0
Keywords: engineered nanomaterials (ENMs), gut microbiota, intestinal permeability, immunomodulation, bacterial components
Citation: Tang M, Li S, Wei L, Hou Z, Qu J and Li L (2021) Do Engineered Nanomaterials Affect Immune Responses by Interacting With Gut Microbiota? Front. Immunol. 12:684605. doi: 10.3389/fimmu.2021.684605
Received: 23 March 2021; Accepted: 26 August 2021;
Published: 14 September 2021.
Edited by:
Diana Boraschi, Shenzhen Institutes of Advanced Technology (CAS), ChinaReviewed by:
Benjamin Swartzwelter, Colorado State University, United StatesMariusz Piotr Madej, OcellO B.V., Netherlands
Copyright © 2021 Tang, Li, Wei, Hou, Qu and Li. This is an open-access article distributed under the terms of the Creative Commons Attribution License (CC BY). The use, distribution or reproduction in other forums is permitted, provided the original author(s) and the copyright owner(s) are credited and that the original publication in this journal is cited, in accordance with accepted academic practice. No use, distribution or reproduction is permitted which does not comply with these terms.
*Correspondence: Liang Li, liang.li@siat.ac.cn; Jing Qu, jing.qu@siat.ac.cn
†These authors have contributed equally to this work and share first authorship