Response of Background Herbivory in Mature Birch Trees to Global Warming
- 1Nakagawa Experimental Forest, Field Science Center for Northern Biosphere, Hokkaido University, Otoineppu, Japan
- 2Wakayama Experimental Forest, Field Science Center for Northern Biosphere, Hokkaido University, Kozagawa, Japan
- 3Graduate School of Environmental Science, Hokkaido University, Sapporo, Japan
- 4Teshio Experimental Forest, Field Science Center for Northern Biosphere, Hokkaido University, Horonobe, Japan
- 5Field Science Center for Northern Biosphere, Hokkaido University, Sapporo, Japan
- 6Graduate School of Agriculture and Life Sciences, The University of Tokyo, Tokyo, Japan
Given the time scale based on the duration of exposure to global warming, natural climate-gradient studies and experimental manipulations have detected long-term (decades to centuries) and short-term (years to decades) ecological responses to global warming. Combination of these two complementary approaches within a single study may enable prediction of the likely responses of ecological processes to global warming. To understand how global warming affects plant–herbivore interactions within a canopy of Erman’s birch, we combined an elevational gradient study and a warming experiment involving mature birch trees in which the soil and tree branches were warmed separately. In the elevational gradient study, herbivory by chewing insects and plant growth increased as elevation decreased, and the concentrations of condensed tannins and total phenolics in the leaves decreased. In the warming experiment, soil warming alone increased herbivory, and the addition of branch warming amplified the effect on herbivory. Soil warming alone decreased the tannin concentration, and the addition of branch warming led to a further reduction. The variation in herbivory was best explained by the tannin content of leaves. Our experimental results demonstrate that the decreased tannin content of leaves due to a combination of soil and branch warming was an important driver of increased herbivory in the canopy of the mature birch trees. The similar tendencies in the short- and long-term responses imply that global warming is likely to increase background herbivory in mature birch trees by decreasing the tannin content of leaves in the canopy.
Introduction
The impact of climate change on plant–herbivore interactions is an important topic in ecology (Bale et al., 2002; Robinet and Roques, 2010; Pureswaran et al., 2018). Within forest stands, insect species diversity is greatest in the high canopy, where associated biological activities are concentrated, rather than in the understory because of the greater plant productivity of mature trees (Basset et al., 2003). However, many field experiments that used warming to examine the effects of global warming have focused on saplings. Many of the physiological and morphological characteristics of woody plants vary during ontogenetic development (Bond, 2000). For example, plant defenses against herbivores often change dramatically from the seedling to the mature stage (Boege and Marquis, 2005; Barton and Koricheva, 2010). This ontogenetic difference, called age-dependent (size-dependent) plasticity (Fischer et al., 2014), has also been observed in plant responses to climate change (Nabeshima et al., 2010; Goodger and Woodrow, 2014). Nabeshima et al. (2010) demonstrated that responses of diameter growth of deciduous trees (Acer mono var. glabrum, A. amoenum, Ostrya japonica, Carpinus cordata, Magnolia obovate, and Quercus crispula) to annual changes in weather conditions (e.g., temperature, precipitation) depended on tree size. Thus, the experimental results obtained in sapling studies may not be entirely relevant for forest ecosystems.
Field-based approaches, most commonly involving natural climate gradients and experimental manipulation, have been used to detect the effects of global warming on natural ecosystems. Given the time scale based on the duration of exposure to global warming, the natural climate gradients provided by changes in elevation and latitude serve as space-for-time substitutions and are therefore useful for predicting long-term (decades to centuries) ecological responses (adaptation) to global warming (Shaver et al., 2000; Sundqvist et al., 2013). However, this approach has several limitations, as long-term adaptations to local conditions and co-varying factors may confound straightforward interpretations of natural gradients (Sundqvist et al., 2013). Conversely, experimental manipulations have fewer confounding factors and provide a mechanistic approach to test the effects of drivers of ecological processes directly (Shaver et al., 2000; Rustad et al., 2001). Therefore, experimental manipulation is useful for detecting short-term (years to decades) ecological responses to global warming (Dunne et al., 2004). However, because such experiments are unable to consider delayed responses, the occurrence of short-term changes may lead to inaccurate predictions of the effects of global warming on natural ecosystems (Wolkovich et al., 2012). Combination of these two complementary approaches within a single study might overcome some of their individual limitations (Anderson and Wadgymar, 2020) and allow predictions of global warming effects (Dunne et al., 2004).
Herbivory plays an important role as a driving force in ecosystem functions in forests (e.g., plant productivity and carbon [C], nutrient, and phosphorous cycles) (Wolf et al., 2008; Metcalfe et al., 2014). Although most studies have paid considerable attention to global warming as a very important factor that directly affects the abundance and species composition of insect herbivores by modulating their survival, development rates, and dispersal (Bale et al., 2002; Robinet and Roques, 2010; Heimonen et al., 2015), indirect effects have received less attention. However, a growing body of evidence shows indirect (plant-mediated) effects of global warming on insect herbivores (e.g., de Sassi et al., 2012; Nakamura et al., 2014; Rasmann et al., 2014; Hamann et al., 2021) and reflects the ability of plants to adjust to global warming via phenotypic plasticity (Nicotra et al., 2010). Indeed, some studies have emphasized that these indirect effects can be stronger than the direct effects (e.g., Barton et al., 2009; O’Connor, 2009).
Many of the effects of temperature on plant phenotypic plasticity, including leaf traits, may be complex (Weih and Karlsson, 2001). According to the C–nutrient balance hypothesis (Bryant et al., 1983), below-ground temperature elevation affects the primary (growth) and secondary (C-based defenses) metabolism of plants (Veteli et al., 2002; Zvereva and Kozlov, 2006). An increase in below-ground temperatures often accelerates soil nitrogen (N) mineralization (Rustad et al., 2001; Melillo et al., 2002), which is expected to lead to a decrease in the concentrations of C-based defensive compounds, as the C becomes allocated to plant growth rather than to defensive-compound synthesis (Veteli et al., 2002; Zvereva and Kozlov, 2006). Furthermore, increases in above-ground temperatures are expected to amplify the effects of increased below-ground temperatures by enhancing plant growth in boreal forests, where air (above-ground) temperatures are the most important limiting factor for plant growth (Ong and Baker, 1985; Weih and Karlsson, 2001). High air temperatures may accelerate C allocation to plant growth rather than to defensive-compound synthesis. Thus, in field experiments involving the canopy of mature trees, the growth of which is frequently limited by low temperatures, both above- and below-ground temperature increases must be considered, alone and in combination, to elucidate the mechanism by which increased temperature affects plant–herbivore interactions (Nakamura et al., 2014).
In this study, we combined an elevational gradient study and a warming experiment involving Erman’s birch trees (Betula ermanii Cham.) growing on Hokkaido, a northern island of Japan, to predict the likely response of plant–herbivore interactions to global warming. Specifically, in the warming experiment, the canopy branches of mature birch trees 18–21 m in height and the soil surrounding their roots were warmed separately, and the results were compared with those of the elevational study. We predict that global warming would increase background herbivory in mature birches by reducing the concentration of leaf C-based defense compounds in the canopy. The background herbivory is defined as the damage to plants occurring when herbivore populations are at their normal densities (Kozlov and Zvereva, 2017). The following questions were addressed. (1) How do background herbivory, plant growth, and leaf traits of birch trees vary along an elevational gradient? (2) How do increased below-ground temperature (soil warming) alone and the combined effects of above- and below-ground (branch and soil) warming affect these same parameters of mature birch trees? (3) Which leaf traits explain the variation in the herbivory rate observed in the elevational gradient study and the warming experiment?
Materials and Methods
Elevational Gradient Study
The focus of this study was Erman’s birch (Betula ermanii Cham.), a common tree line and early successional species (pioneer) that is widely distributed from the plains to the mountains on the northern Japanese island of Hokkaido (Ito, 1987). The elevational gradient study was conducted on Mt. Youtei (42°49′N, 140°48′E, 1898 m a.s.l.), Hokkaido. The study area was a typical stratovolcano with a simple geography. The mean annual temperature at the foot of the mountain (176 m a.s.l.) is 5.7°C, and the mean annual precipitation is 1149 mm. Four sites (at 700, 1000, 1300, and 1600 m) were selected along the elevational gradient of Mt. Youtei in August 2012. The height of Erman’s birch trees was 10–15 m at 700 m, 5–10 m at 1000 m, 3–5 m at 1300 m, and 2–3 m at 1600 m. Since the elevational difference was 900 m, the estimated temperature difference was approximately 5°C using a lapse rate of 0.6°C per 100 m. The vegetation turns into pure stands of Erman’s birch toward higher elevations. At each site, eight Erman’s birch trees growing along narrow forest roads (1–2 m in width) were randomly chosen, and two or three branches approximately 1 cm in diameter were sampled from the top of the canopy of each of these trees using a tree-height pole fitted with a sickle at the top. National and prefectural institutes reported no outbreaks of defoliating insects on Erman’s birch on Mt. Youtei in 2012 (Sayama et al., 2014). Thus, we measured the rates of background herbivory on 50 current-year shoots, including 25 long and 25 short shoots from the canopy branches of each tree. The shoot development of Erman’s birch can be divided into two types: long shoots continuously expand the leaves from early to late, while short shoots expand only the early leaves (Koike, 1995). Herbivory was caused mainly by leaf-chewing insects and late-instar larvae of leaf-mining insects, whereas sap-feeding and gall-making insects were not included. The percent area consumed was scored visually for each leaf, and the percentages were grouped into six ranked indices as follows: 0% = 0, 1–10% = 1, 11–25% = 2, 26–50% = 3, 51–75% = 4, and 76–100% = 5 (Nakamura et al., 2014). The median of each class was used for statistical analysis of herbivory (0 = 0, 1 = 5, 2 = 17.5, 3 = 37.5, 4 = 62.5, and 5 = 87.5%). To identify leaf traits potentially responsible for variation in herbivory rates, 10 leaves were sampled from the canopy of each tree. Ten leaf disks (6 mm radius) punched from each leaf were oven-dried at 40°C for at least 7 days. The mean leaf mass per area (LMA) of each leaf was then calculated. The N and C contents (percentage of dry mass) of the leaves were measured using an NC analyzer (NC-900, Sumika Chemical Analysis Service, Japan), and the C/N ratio was then calculated. The total N content per shoot was calculated as (N content (mg/g) × LMA (g/m2) × leaf size (cm2) × leaf number per shoot)/10,000. Five leaves from each tree were used to determine the concentrations of soluble condensed tannins and soluble total phenolics following the Butanol–HCl and Folin–Ciocalteu methods (Julkunen-Titto, 1985). Plant growth characteristics were assessed by measuring the numbers of leaves per shoot and the lengths of 50 current-year long shoots on each tree.
Warming Experiment
Our warming experiment was conducted in Hokkaido at a different site from the elevational gradient study on Mt. Youtei. The warming experiment, which was based on a factorial design of elevated temperature of soil surrounding the roots and canopy branches of mature birch trees, was conducted in the Nakagawa Experimental Forest of Hokkaido University (44°48′N, 142°14′E, 42 m a.s.l.), where the mean annual temperature is 5.4°C and the mean annual precipitation 1449 mm. In addition to the control condition (Control), the treatments comprised soil warming alone (SW) and soil warming together with canopy branch warming (SWBW). Direct observations were carried out from three steel scaffolds, each 36 m2 (6 m by 6 m) and 20 m in height, with steps that were constructed specifically for this purpose between May and June 2010 around 10 tall B. ermanii trees 18–21 m in height (Nakamura et al., 2016). For the SW treatment, five mature birch trees whose canopies were accessible from two of the scaffolds were selected. In October 2010, we established two 36 m2 (6 m by 6 m) plots at the base of these two scaffolds, one containing two mature birch trees, and one containing three. An electric heating cable made of copper resistance wire and 120 m in length was buried in the soil of each plot at a depth of 5–10 cm at 20 cm intervals, using a previously described method (Melillo et al., 2002; Nakamura et al., 2014). Five mature birch trees whose canopies were accessible from a scaffold were selected as the control. At the base of this scaffold, a 36 m2 (6 m by 6 m) plot was similarly established, and the soil was dug to the same depth as in the warmed plots to ensure a similar level of disturbance to the control trees. Due to the difficulty of experimental manipulation, it was not possible to establish spatially replicated plots. We therefore treated individual tree as a replicate, but we recognize the limitation of not including spatial heterogeneity (Oksanen, 2001; Colegrave and Ruxton, 2018). Due to the early successional species (pioneer), there was plenty of space between the mature trees of B. ermanii. Therefore, these trees were not suppressed by surrounding trees. Soil temperature was set at approximately 5°C higher than the ambient temperature using thermal sensors (K cables) coupled with a controller to regulate the power supply. When the difference in temperature between the control and treatment dropped to <5°C, the controller relay switch opened, and power was supplied to the electric cable. When the difference was ≥5°C, the relay switch closed. The soil was warmed throughout the year. Soil warming increased the temperature of the soil by 5.10 ± 0.51°C (mean ± SD) compared with the ambient temperature.
For the SWBW treatment, we selected four of the five SW trees and warmed an area of approximately 0.5 m × 1.5 m in the canopy of each selected SW tree using infrared heat lamps (500 W, 200 V; Nippon Heater, Tokyo, Japan). The warmed canopy region of each selected SW tree was considered the SWBW treatment, and the rest of the canopy region was considered the SW treatment. The infrared lamps were fixed to the upper parts of the scaffolds (Nakamura et al., 2016). Warming of the canopy branches was started before leaf flush (late May) and continued until after leaf fall (mid-November) of 2012. At the onset of the experiment, the lamps were positioned approximately 30 cm above the tops of the branches. The lamps increased the temperature of the canopy branches by 1.37 ± 1.59°C (mean ± SD) above the ambient temperature (Nakamura et al., 2016).
Because phenotypic plasticity is a property of individual modules (leaves, shoots, and branches) triggered by local environmental conditions rather than a whole-plant response (de Kroon et al., 2005), a modular approach is particularly useful for examining the effects of temperature elevation on mature birch trees. Accordingly, we conducted shoot-level censuses to measure the responses of the mature birch trees to the treatments. Six branches per SW tree and control tree and four branches per SWBW tree were randomly selected for sampling and observation; as only a small part of the canopy was warmed, only four branches were available per tree for the SWBW treatment. Background herbivory rates were assessed using 10 randomly selected current-year shoots, which comprised 5 long and 5 short shoots from each branch using the same method described for the elevational gradient study on Mt. Youtei. Leaf traits including LMA, N and C contents, total N content per shoot, C/N ratio, soluble condensed tannins, and soluble total phenolics were assessed based on two leaves from each branch using the same method described for the elevational gradient study. To assess plant growth characteristics, the length and number of leaves per shoot of the 10 current-year shoots on each branch were measured. The measurements were conducted in September 2010 before the warming experiment started and in September 2012 during the warming experiment to accurately assess how individual mature trees responded to the warming treatments.
Statistical Analyses
In the elevational gradient study, data on herbivory rate, total N content per shoot, C/N ratio, LMA, the concentrations of condensed tannins and total phenolics, shoot length, and number of leaves per shoot were normally distributed (Shapiro–Wilk test, P > 0.05). As the data on N and C contents did not show a normal distribution (Shapiro–Wilk test, P < 0.05), they were arcsine (n)-transformed to satisfy the assumption of normal distribution. Correlation coefficients were used to analyze the strength of the relationships of elevation with herbivory rate, leaf traits, and plant growth characteristics. In all analyses in the elevational gradient study, Individual trees served as replicates (n = 32).
Before the warming experiment started (2010), the herbivory rate, condensed tannin concentration, and shoot length differed significantly among the Control, SW, and SWBW treatments (P < 0.05, Supplementary Figures 1, 2), but several leaf traits (N and C contents, total N content per shoot, LMA, C/N ratio, and total phenolic concentration) as well as number of leaves per shoot did not differ (P > 0.05). To remove the effect of heterogeneity from these variables before the warming experiment, effect sizes were used to compare the herbivory rate, leaf traits, and plant growth characteristics among treatments. We estimated the effect sizes using Cohen’s d metric and 95% confidence intervals (95% CI) using the “meta” package version 4.18 in R 4.0.2 (Schwarzer et al., 2015). Cohen’s d was calculated as the standardized mean difference in the value of each variable from before (2010) to after (2012) the warming experiment in each treatment. A positive effect size indicates that the value of a variable increased from 2010 to 2012 (from before to after the warming experiment), whereas a negative effect size indicates the inverse. Temporal variation between 2010 and 2012 was considered to have a statistically significant effect if the 95% confidence interval of the mean effect size did not include zero. Variation in the effect size of each variable among treatments was explored by calculating the heterogeneity index (QB). The index was tested against the chi-square distribution. All analyses were performed using random effect models. Individual trees served as replicates in the warming experiment analyses (control, n = 5; SW, n = 5; SWBW, n = 4).
To examine whether multiple leaf traits affected elevational and experimental variations in herbivory rates, generalized linear models (GLMs) were applied using a normal distribution with an identity link function; all leaf traits (C/N ratio, N and C contents, condensed tannin and total phenolic concentrations, and LMA) were set as the independent variables. We conducted separate analyses using data from the elevational gradient study and from both 2010 and 2012 to include variation before and after the warming experiment. There was no significant difference in the herbivory rates of the control trees between 2010 and 2012 (ANOVA, df = 9, F = 0.9687, P = 0.354), suggesting similar herbivory pressures during the 2-year period. Before the generalized linear models, we removed leaf traits with a variance inflation factor (VIF) value > 3 because a higher VIF indicates a greater influence of multicollinearity on the variance of an estimated regression coefficient. In both studies, to maintain a low VIF (<3), we removed the C/N ratio, which was strongly correlated with the N content. Model selections were performed based on an information theoretic approach using Akaike’s information criterion (AIC; Burnham and Anderson, 2002). AIC values and Akaike weights were computed for all alternatives to select a best-fit model. According to the principle of parsimony, when alternative models had indistinguishable AIC values (ΔAIC < 2), the model with fewer parameters was selected (Burnham and Anderson, 2002).
Results
Elevational Gradients in Herbivory, Leaf Traits, and Plant Growth
Herbivory rates increased significantly as elevation decreased (F = 66.849, P < 0.001, Figure 1). Most of the measured leaf traits were significantly related to elevation. As elevation decreased, the N content, LMA, and concentrations of condensed tannins and total phenolics decreased significantly (N content, F = 6.294, P = 0.018, Figure 2A; LMA, F = 39.356, P < 0.001, Figure 2C; condensed tannins, F = 51.324, P < 0.001, Figure 2F; total phenolics, F = 9.849, P = 0.004, Figure 2G). In contrast, the total N content per shoot and C/N ratio increased significantly with decreasing elevation (total N, F = 3.070, P = 0.090, Figure 2B; C/N ratio, F = 10.687, P = 0.003, Figure 2D). Although the relationships were marginally significant, the C content also increased with decreasing elevation (C content, F = 3.432, P = 0.074, Figure 2E). Plant growth characteristics such as shoot length and the number of leaves per shoot increased significantly with decreasing elevation (shoot length, F = 56.836, P < 0.001, Figure 2H; leaf number, F = 5.684, P = 0.024, Figure 2I).
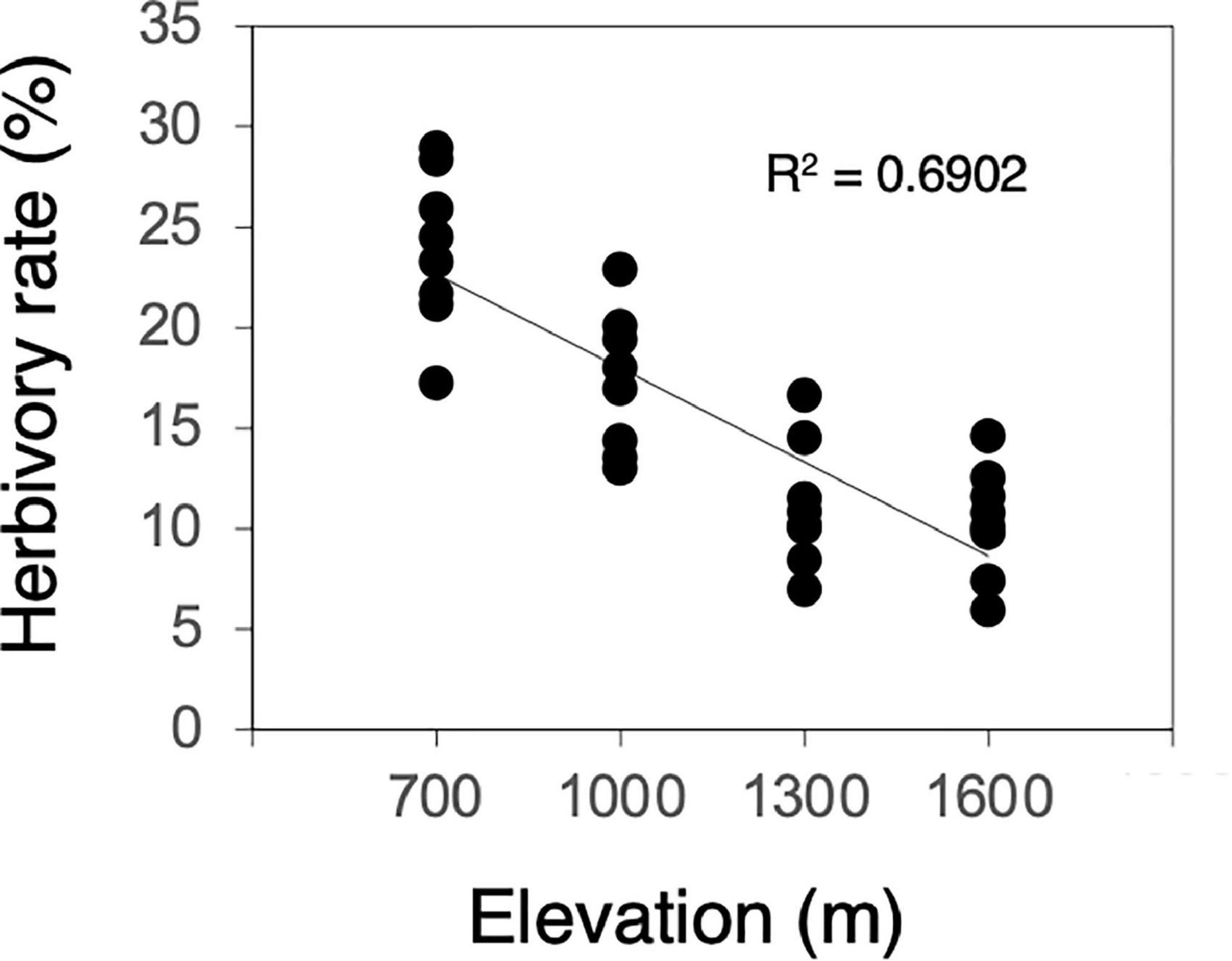
Figure 1. Correlation between herbivory rate and elevation (n = 32). Solid line denotes significant correlation.
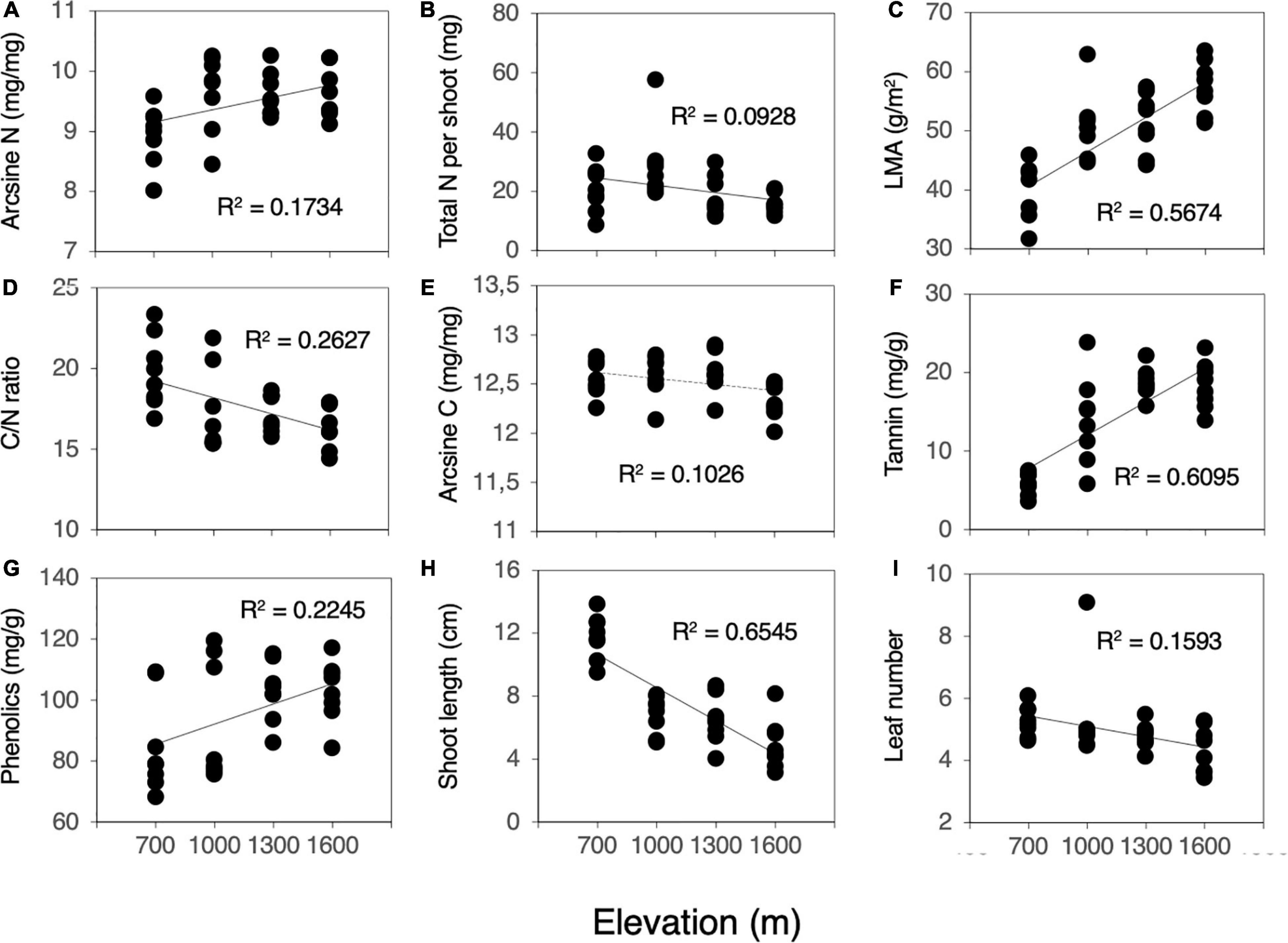
Figure 2. Correlation between plant characteristics (leaf traits and plant growth) and elevation (n = 32). (A) N, (B) Total N per shoot, (C) LMA, (D) C/N ratio, (E) C, (F) Condensed tannin, (G) Total phenolics, (H) Shoot length, and (I) Number of leaves per shoot. The y-axis in (A,E) shows arcsine (n)-transformed N and C contents. Solid lines denote significant correlation, and dashed lines denote marginal significant correlation.
Response of Herbivory to Soil and Branch Warming
Significant differences in the effect size of temporal variation in herbivory were observed among the treatments (QB = 13.62, P = 0.001, Figure 3). The herbivory in control trees did not differ between 2010 and 2012 (before and after the warming experiment), whereas that in SW and SWBW trees increased (SW: 1.464, 95% CI: 0.068–2.860; SWBW: 4.601, 95% CI: 1.955–7.248). SWBW exhibited a stronger positive effect on herbivory than did SW alone.
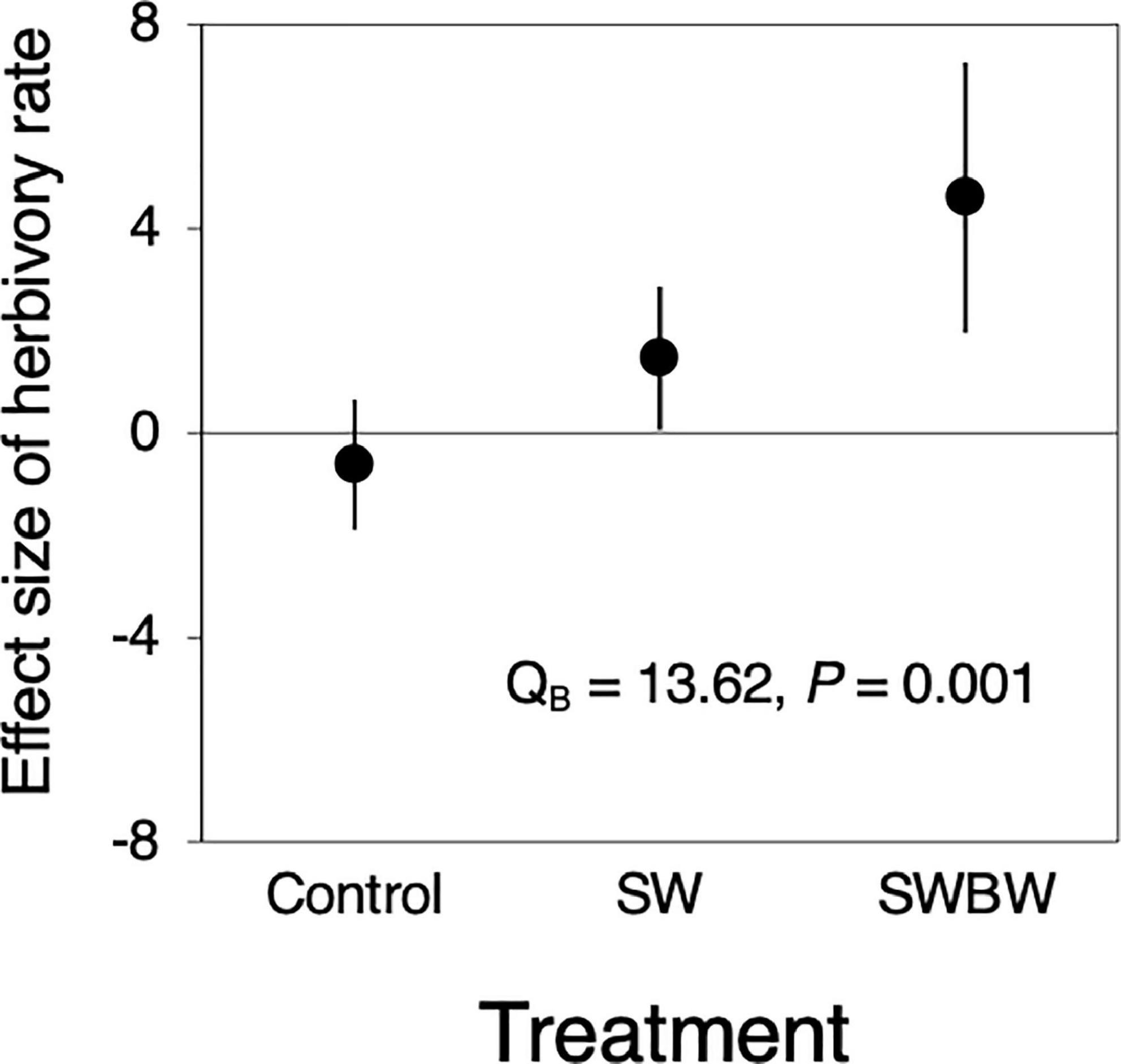
Figure 3. Effect sizes (Cohen’s d) of temporal variation (between 2010 and 2012) in herbivory rate in control trees (Control, n = 5), the soil-warmed trees (SW, n = 5), and soil and canopy branch-warmed trees (SWBW, n = 4). Positive effect size indicates that herbivory rate increased from 2010 to 2012 (before and after the warming experiment). Each dot indicates mean effect size. Each vertical line denotes 95% confidence interval (CI). The effect is significant if the 95% CI does not include zero. Significant QB value indicates between-treatment heterogeneity.
Response of Leaf Traits to Soil and Branch Warming
Differences in the effect size of temporal variation in N and C contents, LMA, and C/N ratio among treatments were not significant (all P > 0.05, Figures 4A,C–E). Although difference in the effect size of temporal variation in total N per shoot was marginally significant (QB = 5.34, P = 0.069, Figure 4B), the effect sizes of temporal variation in condensed tannin and total phenolic concentrations differed significantly among the treatments (condensed tannins: QB = 24.54, P < 0.001, Figure 4F; total phenolics: QB = 6.23, P = 0.044, Figure 4G). These concentrations did not change during this period in control trees, whereas they decreased in SW (tannins: −4.228, 95% CI: −6.458 to −1.999; phenolics: −2.283, 95% CI: −3.876 to −0.690) and SWBW (tannins: −5.677, 95% CI: −8.784 to −2.2569; phenolics: −2.552, 95% CI: −4.419 to −0.685). Furthermore, SWBW showed a slightly stronger negative effect on the condensed tannin concentration than did SW.
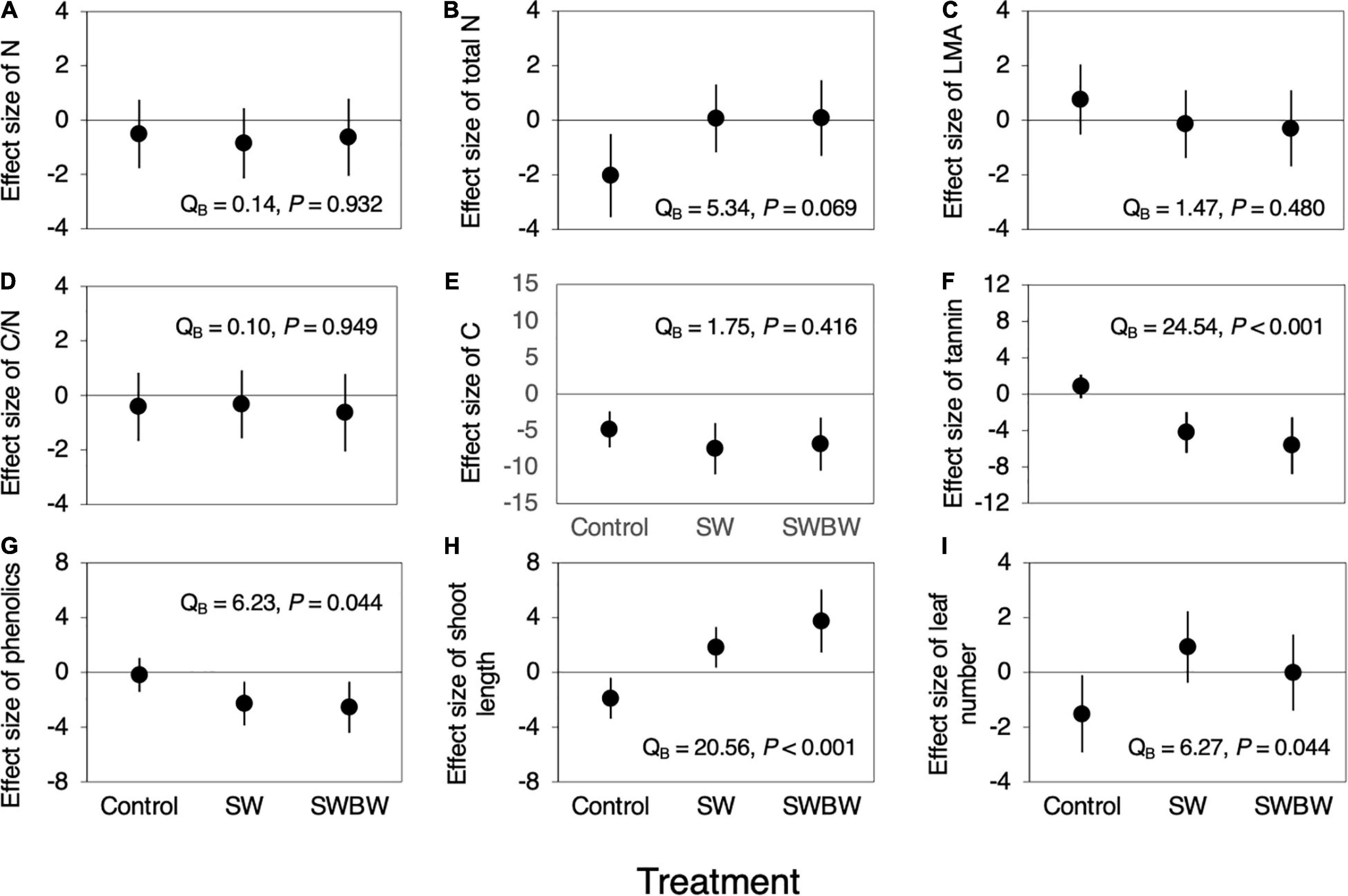
Figure 4. Effect sizes (Cohen’s d) of temporal variation (between 2010 and 2012) in leaf traits and plant growth characteristics in control trees (Control, n = 5), the soil-warmed trees (SW, n = 5), and soil and canopy branch-warmed trees (SWBW, n = 4). (A) N, (B) Total N per shoot, (C) LMA, (D) CN ratio, (E) C, (F) Condensed tannin, (G) Total phenolics, (H) Shoot growth, and (I) Number of leaves per shoot. Positive effect size indicates that leaf traits and plant growth characteristics increased from 2010 to 2012 (before and after the warming experiment). Each dot indicates mean effect size. Each vertical line denotes 95% confidence interval (CI). The effect is significant if the 95% CI does not include zero. Significant QB value indicates between-treatment heterogeneity.
Response of Plant Growth to Soil and Branch Warming
The effect sizes of temporal variation in shoot length and leaf number differed significantly among treatments (shoot length: QB = 20.56, P < 0.001, Figure 4H; leaf number: QB = 6.27, P = 0.044, Figure 4I). Shoot length and leaf number decreased during this period in control trees (shoot length: −1.895, 95% CI: −3.387 to −0.403; leaf number: −1.517, 95% CI: −2.923 to −0.110). However, shoot length increased in SW and SWBW trees during this period (SW: 1.823, 95% CI: 0.350–3.301; SWBW: 3.740, 95% CI: 1.443–6.038), but the difference in leaf number was not significant. These results suggest that SW and SWBW increased the shoot length and leaf number. Furthermore, SWBW yielded a slightly stronger positive effect on shoot length compared with SW.
Leaf Traits Explaining Herbivory Rates in the Elevational Gradient Study and Warming Experiment
According to the principle of parsimony, the model that included the condensed tannin concentration and N content was selected as the best-fit model for elevational variation in herbivory rates (Supplementary Table 1). The regression coefficient for the condensed tannin concentration was negative (−0.701, 95% CI: −0.954 to 0.104) (Supplementary Table 2). Although the slope was marginally significant, the regression coefficient for the N content was negative (−0.441, 95% CI: −0.947 to 0.352). To explain the experimental variation in herbivory rates, the model that included the condensed tannin concentration was selected as the best-fit model (Supplementary Table 1). The regression coefficient for the condensed tannin concentration was negative (−0.149, 95% CI: −0.251 to −0.047) (Supplementary Table 2).
Discussion
Single and Combined Effects of Above- and Below-Ground Warming
A decrease in the defensive compounds of birch saplings during warming experiments has been reported in previous studies, predicting a critical influence of environmental changes during the juvenile stage (Kuokkanen et al., 2004; Zvereva and Kozlov, 2006), but to date, no detailed studies have considered the separate effects of above- and below-ground warming on mature birch trees (but see Weih and Karlsson, 2001). According to the C–nutrient balance hypothesis, in the presence of increased N availability for plants, C is used for plant growth rather than for defensive-compound synthesis (Bryant et al., 1983). Our warming experiment revealed that soil (below-ground) warming alone significantly increased shoot length and leaf number but decreased the concentrations of condensed tannins and total phenolics in the canopy leaves of mature birch trees. These findings imply that increased soil N availability likely caused more C to be allocated to plant growth than to defensive-compound synthesis, probably due to enhanced microbial N mineralization in the warmed soil (Rustad et al., 2001; Melillo et al., 2002). Contrasting soil (below-ground) and air (above-ground) temperatures are likely an important limiting factor in the growth of woody plants in boreal forest (Ong and Baker, 1985; Weih and Karlsson, 2001). Our warming experiment revealed that the addition of branch warming produced a stronger positive effect on shoot length than did soil warming alone but further decreased condensed tannin concentrations in canopy leaves. The greater C allocation to shoot length due to branch warming seemed to further lower condensed tannin concentrations. This implies that below- (soil) and above-ground (branch) warming may have different effects on the production of C-based defensive compounds and plant growth. However, the absence of branch warming alone in our warming experiment limits the interpretation of our experimental results. Thus, future studies examining the effects of branch warming alone are needed to understand the effects of branch warming on plant growth and defensive compounds in mature birch trees. Although an ontogenetic difference in plant responses (age-dependent plasticity) to climate change was expected (Nabeshima et al., 2010; Fischer et al., 2014; Goodger and Woodrow, 2014), similar to the sapling stage of birch trees, our warming experiment revealed that soil and branch warming significantly decreased defensive compounds even during the mature stage.
Although an increase in the N content of canopy leaves in response to soil warming was expected, there was no effect on either the N content or the leaf C/N ratio, despite a tendency for the total N content per shoot to increase. This finding indicates that the increased plant growth due to soil warming may dilute leaf nutrient levels (Veteli et al., 2002).
The model selection revealed that experimental variation in herbivory rates was best explained by condensed tannin concentrations. Although soil warming alone reduced the condensed tannin concentrations of canopy leaves, this effect was amplified by the addition of branch warming. This combined effect was sufficient to drive a significant increase in background herbivory in the canopy of the mature birch trees. Our experimental results provide a mechanistic understanding of how, over the short term (years), the combination of soil and branch warming can induce positive indirect (plant-mediated) ecological effects in the form of increased background herbivory of mature birch trees. However, since individual trees were used as replicates in this study, spatial heterogeneity due to local conditions (e.g., soil types, precipitation, and temperature) may limit the interpretation of our experimental results. In the next step, plot replication and/or block design may provide more general results.
Elevational Gradient
The natural climate gradient is useful to predict long-term (decades to centuries) ecological responses to global warming (Shaver et al., 2000; Sundqvist et al., 2013). Our elevational gradient study with an estimated temperature difference of approximately 5°C (elevation difference of 900 m) revealed a significant increase in background herbivory in canopy leaves at relatively low elevations compared to the high elevations. Furthermore, condensed tannin and total phenolic concentrations decreased at relatively low elevations, while shoot length and leaf number increased. A meta-analysis of 1,027 plant species by Galman et al. (2017) showed that herbivory increased toward lower elevations for deciduous woody species. Similar to this elevational gradient, it is widely accepted that forests at low elevations suffer greater herbivory than those at high elevations (Kozlov, 2008; Kozlov et al., 2013; Lim et al., 2015; Wang et al., 2016; Zhang et al., 2016). These herbivory results supported the pattern detected in our elevational gradient study.
The model selection revealed that the elevational variation in herbivory rates was best explained by the condensed tannin concentrations and N content. This result was partly consistent with the model selection result of the warming experiment. Thus, elevational variation in herbivory likely occurs via a similar mechanism involving the positive indirect (plant-mediated) effects of warmer temperatures. However, the difference in the magnitude ([variable in 2012 – variable in 2010]/variable in 2010) of the herbivory rate from control to SWBW was 0.772, whereas the magnitude from 1600 to 700 m ([variable at 700 m – variable at 1600 m]/variable at 1600 m) was 1.312. Thus, the magnitude of the effect of elevational difference in the elevational gradient study on background herbivory was nearly twice as large as that of increased temperature in the warming experiment. There are at least three possible reasons for the smaller magnitude of the warming experiment effect. First, the magnitude of the effect of above-ground (branch) temperature elevation of only 1.4°C in the warming experiment may have been smaller than that of the above-ground temperature difference (∼5°C) estimated for an elevation difference of 900 m. Second, the period of temperature elevation achieved by branch warming was short, covering just one growing season (6 months). Third, since the soil was warmed in 36 m2 plots, only a small section of the fine roots of each individual mature tree was warmed. These possible explanations imply that the actual magnitude of the effects of global warming on background herbivory may be closer to the result of the elevational gradient study than to that of the warming experiment. In the elevational gradient study, there were only eight individual trees (replications) at each site, but more individual trees may be needed to more accurately calculate the magnitude of the effect of elevational difference.
Prediction of Global Warming Effects
Combination of a natural climate gradient and an experimental manipulation into a single study can provide a research approach that more accurately predicts global warming effects (Dunne et al., 2004). Our results of these two complementary approaches revealed that the short-term responses (years) of plant–herbivore interactions and the growth in the canopy of mature birch trees detected in the warming experiment were consistent with the long-term responses (decades to centuries) detected in the elevational gradient study. Furthermore, a 38-year field monitoring study on forest dynamics in the Nakagawa Experimental Forest revealed an accelerated growth response to increased temperature in B. ermanii (Hiura et al., 2019). Together, these results yield insights into some of the potential effects of global warming. Specifically, we showed that global warming will increase background herbivory in mature birch trees by decreasing tannin concentrations in the canopy. In contrast to mature birch trees (B. ermanii), an early successional species (pioneer), mature oak trees (Quercus crispula), a late successional species (climax), showed decreased herbivory in response to soil warming (Nakamura et al., 2014) and toward lower elevations (Takafumi et al., 2021). These combined results suggest that global warming is likely to decrease background herbivory in mature oak trees. It is possible that the response of herbivory on mature trees to global warming may depend on tree species and their successional status, suggesting that the responses of herbivory to global warming in forests may be predicted from the species composition of woody plants. Several other aspects remain to be considered in future studies. First, triterpenoids on leaf surface exhibit defense properties against herbivores (Reichardt et al., 1984; Laitinen et al., 2004) and its content in silver birch leaves was reported to decrease toward southern latitudes (Makhnev et al., 2012). Since some of triterpenoids have been reported from Erman’s birch (Yamaguchi et al., 2009), changes in the triterpenoid content due to global warming may affect background herbivory in mature birch trees. Second, both direct and indirect effects of global warming on insect herbivores should be considered in assessing the impact of global warming, because global warming directly affects the abundance and species composition of insect herbivores by modulating their survival, development rate, and dispersal (Bale et al., 2002; Robinet and Roques, 2010; Heimonen et al., 2015). Third, previous studies on birch saplings showed that tannin concentrations in birch leaves increased greatly in a CO2-enriched atmosphere, which coincidently increase with increasing temperature (e.g., McDonald et al., 1999; Kuokkanen et al., 2003; Peltonen et al., 2005). Therefore, simultaneous increases in both temperature and CO2 must be considered to better understand the responses of plant defenses and plant–herbivore interactions in the canopy of mature birch trees to actual global changes.
Data Availability Statement
The original contributions presented in the study are included in the article/Supplementary Material, further inquiries can be directed to the corresponding author/s.
Author Contributions
The idea for the study was conceived by MN and TH. The methodology was designed by MN, KT, and HS. Data was collected by MN and MM. Data analysis and interpretation was performed by MN, MM, CT, and KM. MN led the writing of the manuscript. All authors contributed critically to the drafts and gave final approval for publication.
Funding
This work was financially supported by Grants from Japan Society for the Promotion of Science (No. 26450188 to MN, No. 21248017 to TH), and from the Ministry of Environments (D-0909 to TH).
Conflict of Interest
The authors declare that the research was conducted in the absence of any commercial or financial relationships that could be construed as a potential conflict of interest.
Publisher’s Note
All claims expressed in this article are solely those of the authors and do not necessarily represent those of their affiliated organizations, or those of the publisher, the editors and the reviewers. Any product that may be evaluated in this article, or claim that may be made by its manufacturer, is not guaranteed or endorsed by the publisher.
Acknowledgments
We thank Ministry of the Environment and Agency for Cultural Affairs for allowing us to sample branches form Erman’s birch trees at Mt. Youtei. Also thanks to staff members at Nakagawa Experimental Forest, Hokkaido University for their construction of steel pipe scaffolds and support during the study.
Supplementary Material
The Supplementary Material for this article can be found online at: https://www.frontiersin.org/articles/10.3389/ffgc.2021.675401/full#supplementary-material
Supplementary Figure 1 | Original values of herbivory rate before (2010) and after (2012) the warming experiment in control trees (Control: open bar, n = 5), the soil-warmed trees (SW: closed bar, n = 5), and soil and canopy branch-warmed trees (SWBW: closed bar, n = 4). Mean and SE are presented. One-way ANOVA was used to compare among treatments for each year. Tukey test was used for a multiple-comparison between all treatments. Different upper and lower case letters indicate significant differences (P < 0.05) after the multiple-comparison correction before (2010) and after (2012) the warming experiment, respectively.
Supplementary Figure 2 | Original values of leaf traits and plant growth characteristics before (2010) and after (2012) the warming experiment in control trees (Control: open bar, n = 5), the soil-warmed trees (SW: closed bar, n = 5), and soil and canopy branch-warmed trees (SWBW: closed bar, n = 4). (A) N, (B) Total N per shoot, (C) LMA, (D) CN ratio, (E) C, (F) Condensed tannin, (G) Total phenolics, (H) Shoot growth, and (I) Number of leaves per shoot. Mean and SE are presented. One-way ANOVA was used to compare among treatments for each year. Tukey test was used for a multiple-comparison between all treatments. Different upper and lower case letters indicate significant differences (P < 0.05) after the multiple-comparison correction before (2010) and after (2012) the warming experiment, respectively.
Supplementary Table 1 | Summary of the model selection procedure for the variation in herbivory rate in (A) the elevational-gradient study and (B) the warming experiment. The most likely multivariate models (ΔAIC < 2) are shown. The Log-likelihood value (LL), difference of the AIC between the current and the smallest model (ΔAIC), and Akaike weight (wi) are shown.
Supplementary Table 2 | Summary of the best-fit models by GLMs explaining the variation in herbivory rate in (A) the elevational-gradient study and (B) the warming experiment.
References
Anderson, J. T., and Wadgymar, S. M. (2020). Climate change disrupts local adaptation and favours upslope migration. Ecol. Lett. 23, 181–192. doi: 10.1111/ele.13427
Bale, J. S., Masters, G. J., Hodkinson, I. D., Awmack, C., Bezemer, T. M., Brown, V. K., et al. (2002). Herbivory in global climate change research: direct effects of rising temperature in insect herbivores. Global Change Biol. 8, 1–16. doi: 10.1046/j.1365-2486.2002.00451.x
Barton, B. T., Beckerman, A. P., and Schmitz, O. J. (2009). Climate warming strengthens indirect interactions in an old-field food web. Ecology 90, 2346–2351. doi: 10.1890/08-2254.1
Barton, K. E., and Koricheva, J. (2010). The ontogeny of plant defense and herbivory: characterizing general patterns using meta-analysis. Am. Nat. 175, 481–493. doi: 10.1086/650722
Basset, Y., Horlyck, V., and Wright, J. (2003). “Forest canopies and their importance,” in Studying Forest Canopies from Above: the International Canopy Crane Network, eds Y. Basset, V. Horlyck, and J. Wright (Panama: Smithsonian Tropical Research Institute and UNEP), 27–34.
Boege, K., and Marquis, R. J. (2005). Facing herbivory as you grow up: the ontogeny of resistance in plants. Trends Ecol. Evol. 20, 441–448. doi: 10.1016/j.tree.2005.05.001
Bond, B. J. (2000). Age-related changes in photosynthesis of woody plants. Trends Plant Sci. 5, 349–353. doi: 10.1016/S1360-1385(00)01691-5
Bryant, J. P., Chapin, F. S., and Klein, D. R. (1983). Carbon/nutrient balance of boreal plants in relation to vertebrate herbivory. Oikos 40, 357–368. doi: 10.2307/3544308
Burnham, K. P., and Anderson, D. R. (2002). Model selection and multi-model inference: a practical information-theoretic approach. New York: Springer.
Colegrave, N., and Ruxton, G. D. (2018). Using biological insight and pragmatism when thinking about pseudoreplication. Trends Ecol. Evol. 33, 28–35. doi: 10.1016/j.tree.2017.10.007
de Kroon, H., Huber, H., Stuefer, J. F., and van Groenendael, J. M. (2005). A modular concept of phenotypic plasticity in plants. New Phytol. 166, 73–82. doi: 10.1111/j.1469-8137.2004.01310.x
de Sassi, C., Lewis, O. T., and Tylianakis, J. M. (2012). Plant-mediated and nonadditive effects of two global change drivers on an insect herbivore community. Ecology 93, 1892–1901. doi: 10.1890/11-1839.1
Dunne, J. A., Saleska, S. R., Fischer, M. L., and Harte, J. (2004). Integrating experimental and gradient methods in ecological climate change research. Ecology 85, 904–916. doi: 10.1890/03-8003
Fischer, B., van Doom, G. S., Dieckmann, U., and Taborsky, B. (2014). The evolution of age-dependent plasticity. Am. Nat. 183, 108–125. doi: 10.1086/674008
Galman, A., Abdala-Roberts, L., Zhang, S., Teran, J. C. B., Rasmann, S., and Moreira, X. (2017). A global analysis of elevational gradients in leaf herbivory and its underlying drivers: Effects of plant growth form, leaf habit and climatic correlates. J. Ecol. 106, 413–421. doi: 10.1111/1365-2745.12866
Goodger, J. Q. D., and Woodrow, I. E. (2014). “Influence of atmospheric and climate change on tree defence chemicals,” in Trees in a Changing Environment, eds M. Tahsz and N. Gruke (Netherlands: Springer), 165–190.
Hamann, E., Blevins, C., Franks, S. J., Jameel, M. I., and Anderson, J. T. (2021). Climate change alters plant-herbivore interactions. New Phytol. 229, 1894–1910. doi: 10.1111/nph.17036
Heimonen, K., Valtonen, A., Kontunen-Soppela, S., Keski-Saari, S., Rousi, M., Oksanen, E., et al. (2015). Insect herbivore damage on latitudinally translocated silver birch (Betula pendula) – predicting the effects of climate change. Clim. Change 131, 245–257. doi: 10.1007/s10584-015-1392-4
Hiura, T., Go, S., and Iijima, H. (2019). Long-term forest dynamics in response to climate change in northern mixed forests in Japan: A 38-year individual-based approach. For. Ecol. Manag. 449:e117469.
Julkunen-Titto, R. (1985). Phenolic constituents in the leaves of northern willows: methods for the analysis of certain phenolics. J. Agric. Food. Chem. 33, 213–217. doi: 10.1021/jf00062a013
Koike, T. (1995). “Physiological ecology of the growth characteristics of Japanese mountain birch in Northern Japan: a comparison with Japanese white birch,” in Vegetation Science in Forestry, eds E. E. O. Box, R. K. Peet, T. Masuzawa, I. Yamada, K. Fujiwara, and P. F. Maycock (Netherlands: Kluwer Academic Publishers), 409–422.
Kozlov, M. V., van Nieukerken, E. J., Zverev, V., and Zvereva, E. L. (2013). Abundance and diversity of birch-feeding leafminers along latitudinal gradients in northern Europe. Ecography 16, 1138–1149. doi: 10.1111/j.1600-0587.2013.00272.x
Kozlov, M. V., and Zvereva, E. L. (2017). “Background insect herbivory: impacts, patterns and methodology,” in Progress in Botany, Vol. 79, eds F. M. Cánovas, U. Lüttge, and R. Matyssek (Switzerland: Springer), 313–355.
Kozlov, V. M. (2008). Losses of birch foliage due to insect herbivory along geographical gradients in Europe: a climate driven pattern? Clim. Change 87, 107–117. doi: 10.1007/s10584-007-9348-y
Kuokkanen, K., Niemelä, P., Matala, J., Julkunen-Tiitto, R., Heinonen, J., Rousi, M., et al. (2004). The effects of elevated CO2 and temperature on the resistance of winter-dormant birch seedlings (Betula pendula) to hares and voles. Global Change Biol. 10, 1504–1512. doi: 10.1111/j.1365-2486.2004.00820.x
Kuokkanen, K., Yan, S., and Niemelä, P. (2003). Effects of elevated CO2 and temperature on the leaf chemistry of birch Betula pendula (Roth) and the feeding behavior of the weevil Phyllobius maculicornis. Agric. For. Entomol. 5, 209–217. doi: 10.1046/j.1461-9563.2003.00177.x
Laitinen, M. L., Julkunen-Tiitto, R., Yamaji, K., Heinonen, J., and Rousi, M. (2004). Variation in birch bark secondary chemistry between and within clones: implications for herbivory by hares. Oikos 104, 316–326. doi: 10.1111/j.0030-1299.2004.12793.x
Lim, J. Y., Fine, P. V. A., and Mittelbach, G. G. (2015). Assessing the latitudinal gradient in herbivory. Global Ecol. Biogeogr. 24, 1106–1112. doi: 10.1111/geb.12336
Makhnev, A. K., Degtyarev, E. S., and Migalina, S. V. (2012). Intraspecific variability of triterpene content in the leaves of Betula pendula Roth. Contemp. Probl. Ecol. 5, 179–184. doi: 10.1134/S1995425512020096
McDonald, E. P., Agrell, J., and Lindroth, R. L. (1999). CO2 and light effects on deciduous trees: growth, foliar chemistry, and insect performance. Oecologia 119, 389–399. doi: 10.1007/PL00008822
Melillo, J. M., Steudler, P. A., Aber, J. D., Newkirk, K., Lux, H., Bowles, F. P., et al. (2002). Soil warming and carbon-cycle feedbacks to the climate system. Science 298, 2173–2176. doi: 10.1126/science.1074153
Metcalfe, D. B., Gregory, P. A., Martin, R. E., Espejo, E. S., Huasco, W. H., Amézquita, F. F. F., et al. (2014). Herbivory makes major contributions to ecosystem carbon and nutrient cycling tropical forests. Ecol. Lett. 17, 324–332. doi: 10.1111/ele.122233
Nabeshima, E., Kubo, T., and Hiura, T. (2010). Variation in tree diameter growth in response to the weather conditions and tree size in deciduous broad-leaved trees. For. Ecol. Manage. 259, 1055–1066. doi: 10.1016/j.foreco.2009.12.012
Nakamura, M., Makoto, K., Tanaka, M., Inoue, T., Son, Y., and Hiura, T. (2016). Leaf flushing and shedding, bud and flower production, and stem elongation in tall birch trees subject to increases in aboveground temperature. Trees-Struct. Funct. 30, 1535–1541. doi: 10.1007/s00468-016-1387-4
Nakamura, M., Nakaji, T., Muller, O., and Hiura, T. (2014). Different initial responses of the canopy herbivory rate in mature oak trees to experimental soil and branch warming in a soil-freezing area. Oikos 124, 1071–1077. doi: 10.1111/oik.01940
Nicotra, A. B., Atkin, O. K., Bonser, S. P., Davidson, A. M., Finnegan, E. J., Mathesius, U., et al. (2010). Plant phenotypic plasticity in a changing climate. Trends Plant Sci. 15, 684–692. doi: 10.1016/j.tplants.2010.09.008
O’Connor, M. I. (2009). Warming strengthens an herbivore-plant interaction. Ecology 90, 388–398. doi: 10.1890/08-0034.1
Oksanen, L. (2001). Logic of experiments in ecology: Is pseudoreplication a pseudoissue? Oikos 94, 27–38. doi: 10.1034/j.1600-0706.2001.11311.x
Ong, C. K., and Baker, C. K. (1985). “Temperature and leaf growth,” in Control of Leaf Growth, eds N. R. Bakker, W. J. Davies, and C. K. Ong (London: Cambridge University Press), 175–200.
Peltonen, P. A., Vapaavuori, E., and Julkunen-Tiitto, R. (2005). Accumulation of phenolic compounds in birch leaves is changed by elevated carbon dioxide and ozone. Global Change Biol. 11, 1305–1324. doi: 10.1111/j.1365-2486.2005.00979.x
Pureswaran, D. S., Roques, A., and Battisti, A. (2018). Forest insects and climate change. Curr. For. Rep. 4, 35–50. doi: 10.1007/s40725-018-0075-6.pdf
Rasmann, S., Pellissier, L., Defossez, E., Jactel, H., and Kunstler, G. (2014). Climate-driven change in plant-insect interactions along elevation gradients. Funct. Ecol. 28, 46–54. doi: 10.1111/1365-2435.12135
Reichardt, P. B., Bryant, J. P., Clausen, T. P., and Wieland, G. D. (1984). Defense of winter-dormant Alaska paper birch against snowshoe hares. Oecologia 65, 58–69. doi: 10.1007/BF00384463
Robinet, C., and Roques, A. (2010). Direct impacts of recent climate warming on insect populations. Integr. Zool. 5, 132–142. doi: 10.1111/j.1749-4877.2010.00196.x
Rustad, L., Campbell, J., Marion, G., Norby, R., Mitchell, M., Hartley, A., et al. (2001). A meta-analysis of the response of soil respiration, net nitrogen mineralization, and aboveground plant growth to experimental ecosystem warming. Oecologia 126, 543–562. doi: 10.1007/s004420000544
Sayama, K., Ozaki, K., Hara, H., and Onodera, K. (2014). Forest insect herbivores occurred in Hokkaido in 2012. Northern Forestry, Japan 66, 15–17.
Schwarzer, G., Carpenter, J. R., and Rücker, G. (2015). Meta-Analysis with R. Switzerland: Springer International Publishing.
Shaver, G. R., Canadell, J., and Chapin, F. S. (2000). Global warming and terrestrial ecosystems: a conceptual framework for analysis. Bioscience 50, 871–882. doi: 10.1641/0006-3568
Sundqvist, N. K., Sanders, N. J., and Wardle, D. A. (2013). Community and ecosystem responses to elevational gradients: processes, mechanisms, and insights for global change. Annu. Rev. Ecol. Evol. Syst. 44, 261–280. doi: 10.1146/annurev-ecolsys-110512-135750
Takafumi, H., Kanno, Y., Abe, S., Abe, T., Enoki, T., Hirao, T., et al. (2021). Assessing insect herbivory on broadleaf canopy trees at 19 natural forest sites across Japan. Ecol. Res. 36, 562–572.
Veteli, T. O., Kuokkanen, K., Julkunen-Tiito, R., Roininen, H., and Tahvanainen, J. (2002). Effects of elevated CO2 and temperature on plant growth and herbivore defensive chemistry. Global Change Biol. 8, 1240–1252. doi: 10.1046/j.1365-2486.2002.00553.x
Wang, X. F., Liu, J. F., Gao, W. Q., Deng, Y. P., Ni, Y. Y., Xiao, Y. H., et al. (2016). Defense pattern of Chinese cork oak across latitudinal gradients: influences of ontogeny, herbivory, climate and soil nutrients. Sci. Rep. 6:27269. doi: 10.1038/srep27269
Weih, M., and Karlsson, P. S. (2001). Growth response of mountain birch to air and soil temperature: is increasing leaf-nitrogen content an acclimation to lower air temperature? New Phytol. 150, 147–155. doi: 10.1046/j.1469-8137.2001.00078.x
Wolf, A., Kozlov, M. V., and Callaghan, T. V. (2008). Impact of non-outbreak insect damage on vegetation in northern Europe will be greater than expected during a changing climate. Clim. Change 87, 91–106. doi: 10.1007/s10584-007-9340-6
Wolkovich, E. M., Cook, B. I., Allen, J. M., Crimmins, T. M., Betancourt, J. L., Travers, S. E., et al. (2012). Warming experiments underpredict plant phonological responses to climate change. Nature 485, 494–497. doi: 10.1038/nature11014
Yamaguchi, C., In, Y., Wada, S., Yamada, T., Tokuda, H., and Tanaka, R. (2009). Cancer chemopreventive activity of oleanane-type triterpenoids from the stem bark of Betula ermaii. Chem. Biodivers. 6, 1093–1100. doi: 10.1002/cbdv.200800266
Zhang, S., Zhang, Y., and Ma, K. (2016). latitudinal variation in herbivory: hemispheric asymmetries and the role of climatic drivers. J. Ecol. 104, 1089–1095. doi: 10.1111/1365-2745.12588
Keywords: above-ground warming, below-ground warming, experimental manipulation, natural gradient, plant-mediated effect
Citation: Nakamura M, Minoshima M, Terada C, Takagi K, Makoto K, Shibata H and Hiura T (2021) Response of Background Herbivory in Mature Birch Trees to Global Warming. Front. For. Glob. Change 4:675401. doi: 10.3389/ffgc.2021.675401
Received: 03 March 2021; Accepted: 25 August 2021;
Published: 22 September 2021.
Edited by:
Akihiro Nakamura, Xishuangbanna Tropical Botanical Garden, Chinese Academy of Sciences (CAS), ChinaReviewed by:
Markku Keinänen, University of Eastern Finland, FinlandLouise A. Ashton, The University of Hong Kong, Hong Kong, SAR China
Copyright © 2021 Nakamura, Minoshima, Terada, Takagi, Makoto, Shibata and Hiura. This is an open-access article distributed under the terms of the Creative Commons Attribution License (CC BY). The use, distribution or reproduction in other forums is permitted, provided the original author(s) and the copyright owner(s) are credited and that the original publication in this journal is cited, in accordance with accepted academic practice. No use, distribution or reproduction is permitted which does not comply with these terms.
*Correspondence: Masahiro Nakamura, masahiro@fsc.hokudai.ac.jp