Effects of warming and litter positions on litter decomposition in a boreal peatland
- 1Key Laboratory of Wetland Ecology and Environment, Northeast Institute of Geography and Agroecology, Chinese Academy of Sciences, Changchun, China
- 2Key Laboratory of Sustainable Forest Ecosystem Management-Ministry of Education, School of Forestry, Northeast Forestry University, Harbin, China
- 3Heilongjiang Sanjiang Plain Wetland Ecosystem Research Station, Fuyuan, China
Litter decomposition is an important source of carbon accumulation in the permafrost peatlands. Climate warming has led to shrub expansions and accelerated litter mixing with soils and fluctuations in the water table. However, little is known about how changes in the position of the litter will affect litter decomposition under climate warming. To reveal the mechanisms of response of the location of litter in the soil and climate warming to litter decomposition in permafrost peatlands. Here, we selected the evergreen shrub, Chamaedaphne calyculata, and the deciduous shrub, Vaccinium uliginosum, from the permafrost peatlands of the Greater Hing’an Mountains, China. The leaf litter was placed on the soil surface (no-mixing) and mixed with the soil (soil-litter mixing), and then it was incubated for 124 days at 15°C (control) and 20°C (warming). Our results showed that warming significantly increased the CO2 emission rates of C. calyculata and V. uliginosum by 19.9 and 17.4%, respectively. When compared to no-mixing, the CO2 emission rates were reduced (not significantly) by 1.5 (C. calyculata) and increased 13.6% (V. uliginosum) with soil-litter mixing. Interestingly, soil-litter mixing suppressed the positive effect of warming on the CO2 emission rates relative to no-mixing, and the suppressing effects in the V. uliginosum subplot were stronger than those in the C. calyculata subplot. Specifically, warming significantly increased the CO2 emissions of C. calyculata by 27.4% under no-mixing but the increase decreased to 13.1% under soil-litter mixing. Similarly, warming induced significant increases in the CO2 emissions of V. uliginosum, with an increase of 38.8% under no-mixing but non-significant increases (1.9%) were observed under soil-litter mixing. The combination of the enzyme activities of β-1,4-glucosidase, β-1,4-xylosidase and β-D-1,4-cellobiosidase and laccase and phenolics explained more than 60.0% of the variability in the CO2 emissions of C. calyculata and V. uliginosum, respectively. Our study highlights the importance of litter positions in mediating the responses of litter decomposition to climate warming and shrub expansions in the northern peatlands.
Introduction
Around 400–500 Pg of C has been stored in the peatlands since the beginning of the Holocene (Yu et al., 2010). The distribution of the peat soil organic carbon is uneven and widespread across the northern peatlands. This occurs mainly because organic matter decomposition is limited due to the low temperature in this ecosystem, resulting in an imbalance between plant production and organic matter decomposition (Freeman et al., 2001; Moore and Basiliko, 2006). The consequence is a large accumulation of peat, composed of dead plant litter (Clymo, 1984). Thus, litter decomposition is one of the key factors affecting soil organic carbon storage in the northern peatlands (Strakova et al., 2012). Climate warming has been identified as an essential driver of litter decomposition. Warming accelerates changes in the community compositions (Zhang, Y. et al., 2022), such as vascular plant expansions (Luan et al., 2019), and the mixture of the soils and litter (Song et al., 2017). However, there is very limited knowledge about the interactive effects of warming and changes in the plant community structure and litter positions on litter decomposition in the northern peatlands. Therefore, to accurately assess and predict the carbon balance of the boreal peatlands, it is crucial to re-examine litter decomposition and its response to climate warming.
The effect of climate warming on litter decomposition could be regulated by functional groups in the northern peatlands. It is commonly accepted that climate warming effectively increases microbial activities, and, thus, stimulates litter decomposition (Ferreira et al., 2015; Liu et al., 2017). In contrast, the neutral and negative effects of warming on litter decomposition have also been emphasized in the northern peatlands (Aerts, 2006; Boyero et al., 2011; Butenschoen et al., 2011). These discrepancies suggest that climate warming alone is not enough to explain the complex variability in litter decomposition and, therefore, it might also be regulated by functional groups. With climate warming, there is growing evidence that the northern peatlands will experience greater vascular plant expansions (Elmendorf et al., 2012; Sistla et al., 2013; Dieleman et al., 2015). Additionally, due to the differences in the litter chemistries of vascular plants, it has been found that the decomposition rate of leaf litter from the deciduous shrub Betula nana is higher than that of the evergreen shrubs Vaccinium vitis-idaea and Rhododendron palustre (McLaren et al., 2017). However, studies on the differences in the responses of the decomposition of different vascular plants to climate warming in the northern peatlands are still limited.
The uncertainties of the effects of warming on decomposition might also be attributed to the litter positions. Strong winds and fluctuations in the water table could lead to the topsoil mixing with the litter under climate warming (Hewins et al., 2017). Soil-litter mixing could influence litter decomposition through two different but equally important ways, including (1) acting as a microbial colonization vehicle on the surface of the litter and (2) insulating the litter from temperature or moisture, thus, extending the time window for microbially mediated decomposition opportunities (Throop and Archer, 2007). Recent incubation experiments (Gao et al., 2022; Zhang, X. H. et al., 2022) and in situ litter decomposition in arid ecosystems (Siegenthaler et al., 2010; Corteselli et al., 2017) have well documented the differences in the litter decomposition rates among litter positions. Nevertheless, current studies have mostly focused on the litter decomposition rates in the surface soils but have not considered soil-litter mixing. Considering the important role that soil-litter mixing plays in affecting the litter decomposition, lacking related studies of soil-litter mixing in the northern peatlands potentially amplifies the critical uncertainties regarding the response of CO2 emissions that are induced by litter decomposition due to climate warming.
Peatlands are widespread in the Greater Hing’an Mountains, which is experiencing rapid climate warming and, consequently, permafrost degradation, leading to shrub expansion and changes in the water table, which may induce changes in the litter position. Therefore, the boreal peatlands of the Greater Hing’an Mountains provide a rare opportunity to investigate the linkages between climate warming, species change, litter position, and litter decomposition. Here, we collected fresh litter from the evergreen shrub Chamaedaphne calyculata and the deciduous shrub Vaccinium uliginosum in the Greater Hing’an Mountains. The litter was located on the soil surface or mixed with the soil, and they were incubated at 15 and 20°C for a period of 124 days to evaluate the response of the CO2 emission rates to warming, the functional group, litter positions, and their interactions. Therefore, the aims of the study were (1) to estimate the impacts of warming on the CO2 emission rates of litter decomposition and differentiate whether the warming impacts varied due to the functional groups and litter positions; and (2) to identify the driving factors of the response of the CO2 emission rates from litter decomposition to the different treatments.
Materials and methods
Study region
Plant litter and soil samples were collected from a site in the Greater Hing’an Mountains permafrost peatlands in northeastern China (52.56 °N, 122.51 °E, 467 m a.s.l.). The region has a cool temperate continental monsoon climate. The mean annual temperature and precipitation at the study site over the last 30 years were −3.9°C and 452 mm (Song et al., 2018), respectively. The vegetation of the study site is relatively simple, including the shrubs Betula fruticosa, C. calyculata, and V. uliginosum, Cyperaceae Eriophorum vaginatum, and bryophyte Sphagnum palustre (Zhang, X. H. et al., 2022). In this study, we selected the litter of the evergreen shrub C. calyculata and deciduous shrub V. uliginosum to study the effects of different temperatures and different locations on the early decomposition of peatland litter in permafrost regions.
Sample collection and incubation
Six 20 × 20 m plots were randomly selected in the peatland. Within each sample plot, five peat cores (10 cm inner diameter) were randomly collected in the 0–10 cm soil layer in early September 2021. The soil samples were air-dried after removing the plant roots, and all the samples were mixed evenly and passed through a 2 mm sieve. The fresh litter was collected by gently shaking the shrubs. For each species, the plant litter that was sampled from the different plots was mixed well, oven-dried at 65°C, and divided into two subsamples. One subsample was used for the incubation experiments, and the other subsample was oven dried to a constant weight at 65°C to determine the moisture content and milled to pass through a 0.15 mm sieve for chemical analysis (the C, N, P, and phenolic initial content) (Table 1).
The litter decomposition C emission rate was determined by a laboratory incubation experiment (Gao et al., 2022). For each litter treatment, a cylindrical culture bottle (12 cm in diameter and 16 cm in height) was filled with 80 g of dry soil at the bottom, and the dry soil was adjusted to 60.0% of the maximum water-holding capacity in the field with 0–10 cm of pore water from the peatlands. The location of the litter (5 g) in the soil was divided into two treatments: at the soil surface (no-mixing) and fully mixed with the soil (soil-litter mixing). To prevent the litter from absorbing soil water, the litter was soaked with pore water in advance until it was saturated. Then, it was cultivated in the dark at 15°C (control) and 20°C (warming) for 124 days, which represents the average temperature of the growing season from May to September (13°C) and July (18°C) at the sampling sites, respectively. In total, there were 40 microcosm samples (2 litter treatments × 2 incubation temperatures × 2 litter locations × 4 replicates). During the incubation period, to maintain a constant microcosm sample moisture, water supplementation was carried out by weighing the samples every 7 days. The frequency of the sampling was increased at the beginning of the incubation period because the high C content that was available at the beginning of the decomposition of the litter contributed to a high respiration rate for the microorganisms (Mao et al., 2018; Gao et al., 2022). Additionally, a gas-tight syringe was used to collect 30 ml of the sample gas on days 1, 2, 4, 7, 11, 17, 24, 34, 44, 64, 84, 104, and 124 of the incubation. The bottles were closed tightly, the plastic cap was fitted with the pumping port for 24 h before pumping, and they were open for the rest of the incubation time. The analysis of the sample CO2 concentration in the syringes was conducted within 24 h using an Agilent 7820A gas chromatograph (Agilent Technologies, Carpinteria, CA, United States). The CO2 release rate was calculated as described by Robertson et al. (1999). After the incubation, the litter was collected and its C, N, P, and phenolic compound contents were measured.
Sample analysis
We used 5 g of fresh soil to determine the potential activity of four hydrolytic enzymes β-1,4-glucosidase (βG, EC 3.2.1.21), β-1,4-xylosidase (βX, EC 3.2.1.37), β-D-1,4-cellobiosidase (CBH, EC 3.2.1.91), and β-1,4-N-ace-tylglucosaminidase (NAG, EC 3.2.1.14) and the oxidative enzyme laccase (EC 1.10.3.2). The enzymes, βG, βX, CBH, and NAG were assayed using 4-methylumbelliferone (MUB)-β-D-glucoside, 4-MUB-β-D-xyloside, 4-MUB-β-D-cellobioside, and 4-MUB-N-acetyl-β-D-glucosaminide, respectively, as the specific substrates. The hydrolases were analyzed using a black 96-well microtiter plate. Briefly, under the different treatments, 5 g of soil was mixed with 150 ml of 50 mM sodium acetate buffer (pH 5.5) in a high-speed stirrer for 10 min. Then, 4-MUB (10 mM) was used as a standard solution. Next, using an 8-channel electronic pipette, we added 200 μl of the soil suspension and 50 μl of sodium acetate buffer (blank control), 200 μl of the soil suspension and 50 μl of the standard solution (quench standard), 200 μl of sodium acetate buffer and 50 μl of the standard solution (reference standard), 200 μl of sodium acetate buffer and 50 μl of enzyme substrate (negative control), and 200 μl of the soil suspension and 50 μl of enzyme substrate (sample controls). After completion, the microplates were incubated in the dark at 25°C for 4 h. Subsequently, 10 μl of 0.5 mol L−1 NaOH solution was added to each well to stop the reaction. The fluorescence values of each well were measured using a Multi-Detection Microplate Reader (CYTATION 5, BioTek, United States) with excitation and emission wavelengths of 365 mm and 450 mm, respectively. The activity of phenol oxidase was estimated using 2,2-azino-bis (3-ethylbenzthiazoline-6-sulfonic acid) as a substrate. Furthermore, estimation of the potential laccase activity for promoting polyphenol degradation was conducted on 96-well transparent microtiter plates. Each assay microplate also contained a sample well (50 μl of the substrate solution and 200 μl of the soil suspension), blank well (200 μl of the soil suspension and 50 μl of the sodium acetate buffer), and negative control (200 μl of sodium acetate buffer and 50 μl of the substrate solution). The plates were incubated at 20°C in darkness for at least 2 h (Rinkes et al., 2013). Also, the activity was quantified by measuring the absorbance at 420 nm using a Multi-Detection Microplate Reader (BioTek; Eichlerova et al., 2012), and the enzyme activities were expressed in nmol h−1 g−1.
To measure the extractable phenolic content of each plant litter sample, the Folin–Ciocalteu method was used to determine the phenolic concentration (Bärlocher et al., 2020). We put 0.2 g of the litter into a 50 ml centrifuge tube, added 30 ml of 95% methanol solution, and sonicated it at 540 W and 40–50°C for 30 min, three times. The extracts were combined, and they were centrifuged at 5000 r/min for 15 min, 0.2 ml of supernatant was transferred to a 10 ml centrifuge tube, 4.8 ml of deionized water was added and it was shaken well, 0.5 ml of Folin-Ciocalteau’s reagent and 1.5 ml of Na2CO3 were added, and the sample was mixed thoroughly and incubated at 30°C for 2 h. The same procedure was applied to 10 mg/ml of gallic acid standard to produce a standard curve. The absorbance values were measured at 760 nm.
The total carbon (TC) and nitrogen (TN) were measured on a TC-TN analyzer (Shimadzu, Tokyo, Japan). To determine the concentration of Phosphorus (TP), the litter samples were digested at 365°C in concentrated H2SO4 and an AA3 continuous flow autoanalyzer (Seal AA3 Analytical, Norderstedt, Germany) was used.
Statistical analysis
The effects of the experimental warming, different vegetation litter, different locations, and their interaction effects, were analyzed using a repeated measures analysis of variance and IBM SPSS Statistics 20, with the sampling date as a random effect. This was followed by Tukey’s comparison test, which was used to compare the difference in the means of the litter’s chemical properties. Additionally, a new method, relative weights, was used to quantify the relative contributions of the NAG, litter stoichiometry, phenolics, laccase, and carbon cycle enzymes to the CO2 emission rate. This approach approximates the average increase in R2 (coefficient of determination), which is obtained by adding a predictor variable to allthe possible submodels (LeBreton and Tonidandel, 2008), and it has been used in recent analyses (Chen et al., 2021). In this study, a principal component analysis (PCA) was used to reduce the number of variables that were tested (Cardenas et al., 2015). The PCA was performed on the βG, CBH, and βX enzyme activities and the litter C, N, P, C/N, C/P, and N/P, and their final scores were used as variables for the relative weight analysis of the carbon cycle enzymes and litter stoichiometry, respectively.
Results
Effects of warming and the litter positions on the CO2 emissions
The warming and litter positions and their interactions significantly affected the CO2 emission rates (p < 0.05; Figure 1; Table 2). Warming induced significant increases in the CO2 emissions of C. calyculata and V. uliginosum, which were 19.9 and 17.4% (p < 0.05), respectively, relative to the control treatment (Figure 1; p < 0.05). When compared to the soil-litter mixing treatment, the CO2 emission rates of C. calyculata and V. uliginosum were reduced (not significantly) by 1.5% (p > 0.05) and increased by 13.6% (p > 0.05), respectively (Figure 1). It was also observed that warming-induced positive effects on the CO2 emission rates were significantly negatively regulated by the litter positions of the observed species (Figure 1; Table 2; p < 0.05). The CO2 emission rate of C. calyculata under the warming treatment was significantly 27.4 and 13.1% higher than that of the control in the no-mixing and soil-litter mixing treatments, respectively (p < 0.05; Figure 1). Then, in the no-mixing treatment, the CO2 emission rate of V. uliginosum under the warming treatment was, on average, 2.99 ± 0.16 C kg−1 h−1 mg higher than that of the control (p < 0.05) but this significant difference, which was induced by warming, disappeared in the soil-litter mixing treatment (p > 0.05; Figure 1). Overall, compared with no-mixing, soil-litter mixing decreased the sensitivity of the litter CO2 emission rate to warming but the decreases for V. uliginosum were higher than those for C. calyculata.
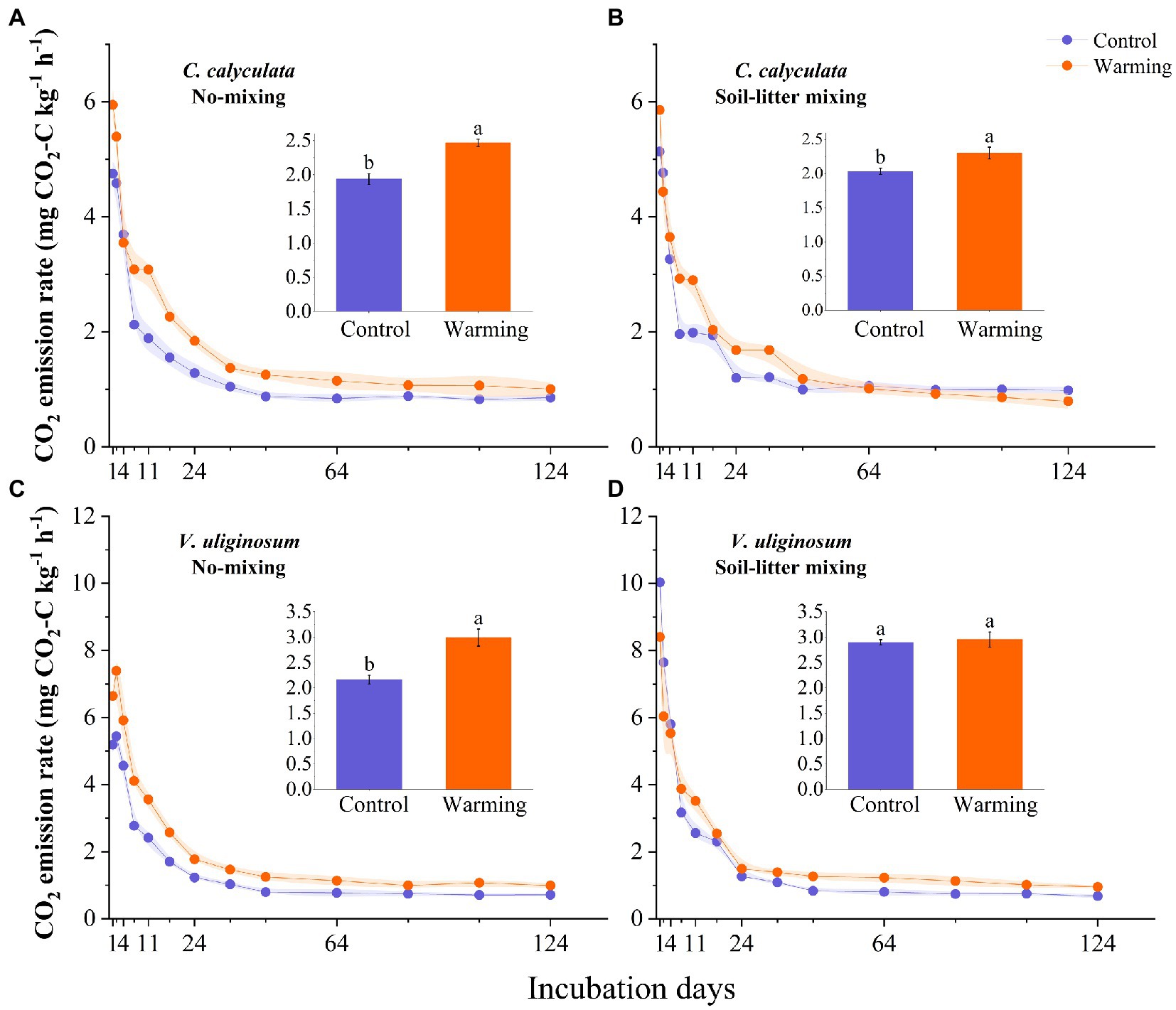
Figure 1. The dynamic CO2 emissions rate for the C. calyculata (A,B) and V. uliginosum (C,D) litter decomposition under warming and treatments with different positions. On each solid line, the shading around the points represents the mean ± standard deviation (n = 4 replicates). The panel inset shows the average CO2 emission rate throughout the incubation period, under the different treatments (the different letters in the insets represent statistical differences (p < 0.05) based on a Tukey’s multiple comparison test).
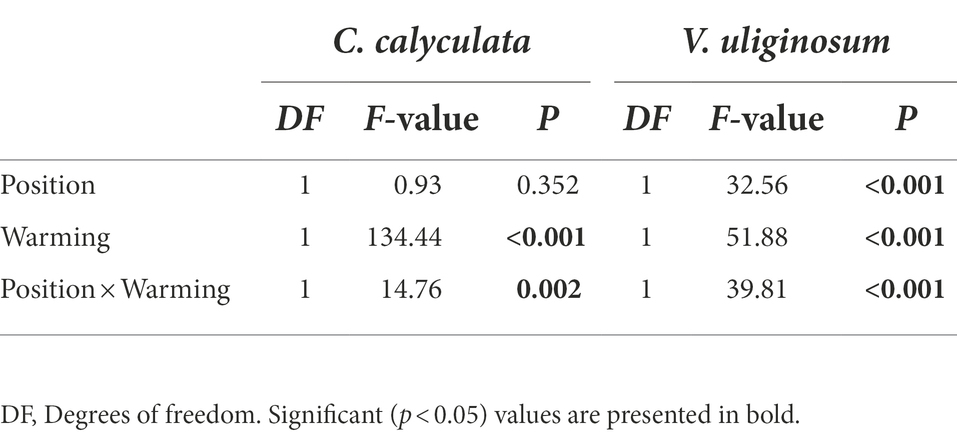
Table 2. A repeated measures analysis of variance shows the effects of climate warming and litter location and the interactions between them on the CO2 emission rates from the different plant litter (C. calyculata and V. uliginosum).
Treatment effects on the control factors of the CO2 emission rate
Enzyme activities
Warming increased the enzyme activities of βG, βX, CBH, and NAG for C. calyculata by 69.1 (p < 0.05), 26.9 (p > 0.05), 164.5 (p < 0.05), and 66.2% (p < 0.05) relative to the control but laccase decreased by 20.0% (p > 0.05; Figure 2). The responses of the enzyme activities to warming were regulated by the litter positions. In the no-mixing treatment, warming significantly increased the activities of βG and CBH for C. calyculata (p < 0.05; Figure 2). The increase in the βG and CBH activities due to warming under the soil-litter mixing treatment was less than that of the no-mixing treatment (Figure 2). In contrast to βG and CBH, which warmed slightly, and NAG which increased in the no-mixing treatment (p > 0.05), significant increases were observed in the soil-litter mixing treatment (p < 0.05; Figure 2). Additionally, the activities of laccase and βX for C. calyculata showed non-significant changes with warming under the no-mixing and soil-litter mixing treatments (p > 0.05; Figure 2).
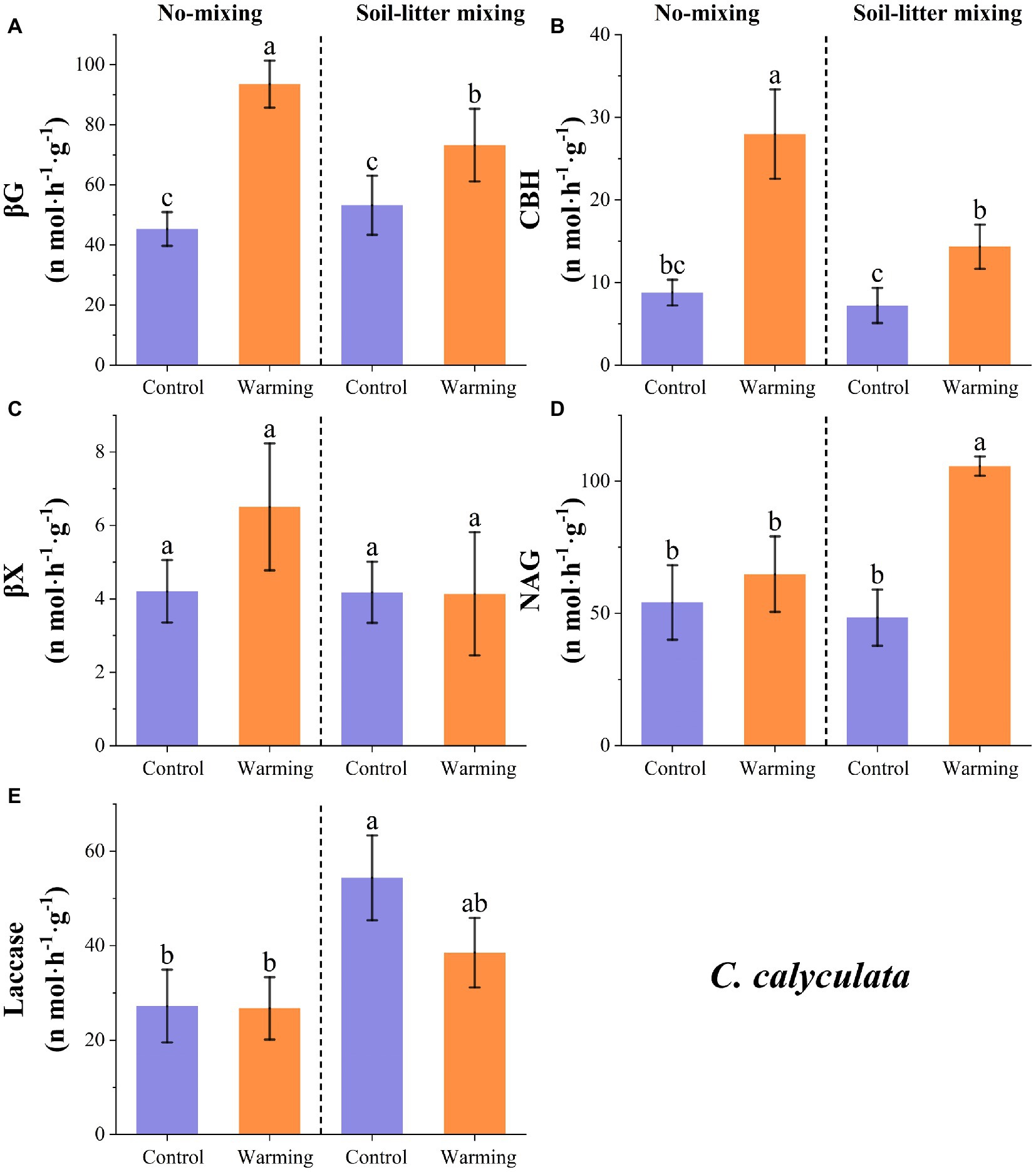
Figure 2. The responses of the potential enzyme activities of C. calyculata to warming under the no-mixing and soil-litter mixing treatments. The enzymes are (A) β-glucosidase (βG), (B) Cellobiohydrolase (CBH), (C) β-xylosidase (βX), (D) N-acetyl-glucosaminidase (NAG), and (E) Laccase. The values are the mean ± standard deviation of each treatment (n = 4). The different letters indicate significant differences (p < 0.05) in the enzyme activities under the different treatments.
The enzyme activities of βG, CBH, and NAG for V. uliginosum were, on average, 24.5 (p < 0.05), 57.9 (p < 0.05), and 16.3% (p > 0.05) lower, respectively, in the warmed treatment than in the control treatment but increases were observed for βX and laccase, which increased by 10.8 (p > 0.05) and 25.6%, respectively (p < 0.05; Figure 3). The sensitivity of the enzyme activities to warming varied with the litter positions. Warming induced significant increases (and insignificant reductions) in laccase (βG and CBH) for V. uliginosum in the no-mixing treatment but this significant difference was not detected in the soil-litter mixing treatment (Figure 3; p > 0.05). Both NAG and βX showed insignificant (p > 0.05) changes in response to warming under either the no-mixing or soil-litter mixing treatments (Figure 3).
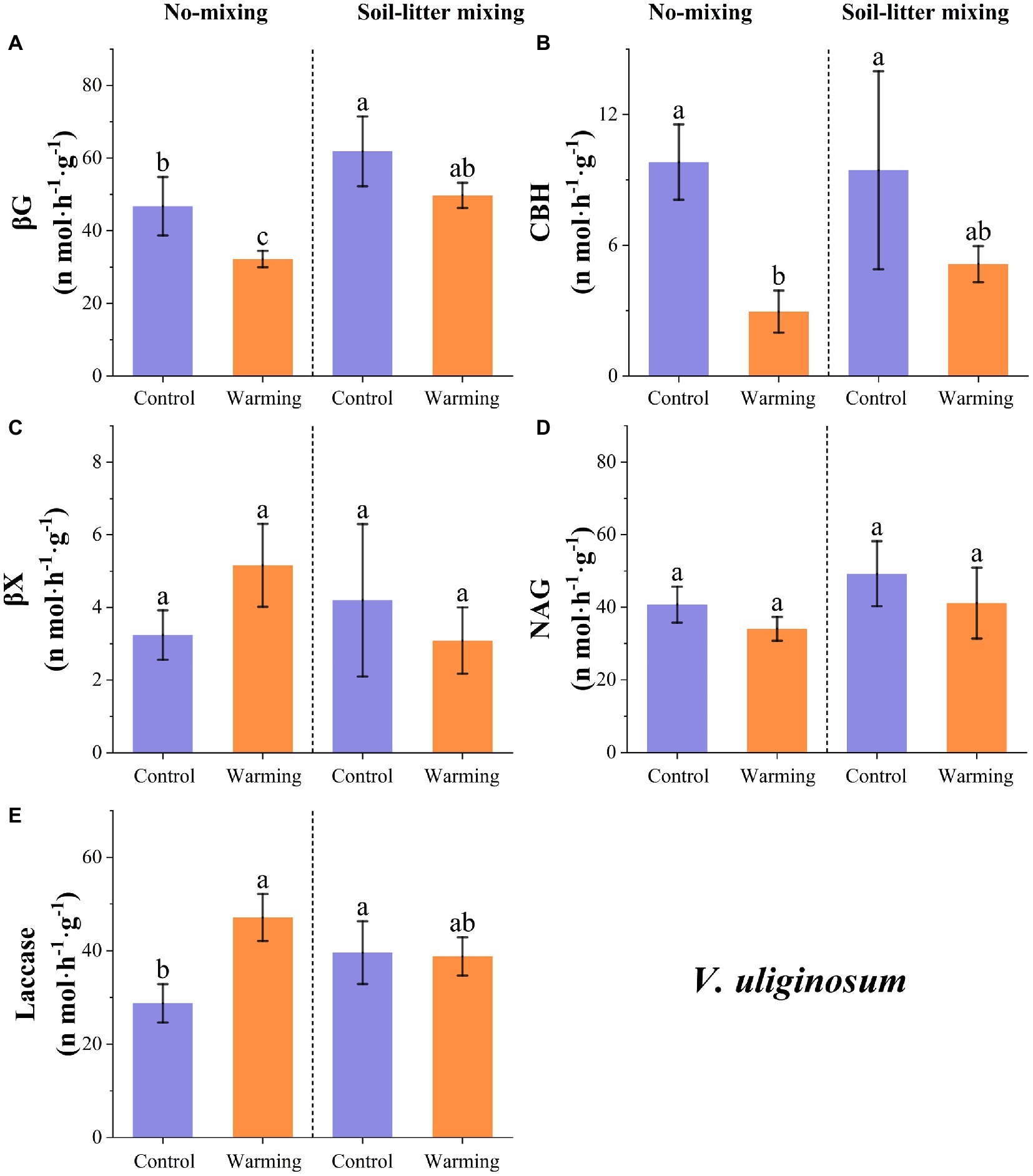
Figure 3. The responses of the potential enzyme activities of V. uliginosum to warming under the no-mixing and soil-litter mixing treatments. The enzymes are (A) β-glucosidase (βG), (B) Cellobiohydrolase (CBH), (C) β-xylosidase (βX), (D) N-acetyl-glucosaminidase (NAG), and (E) Laccase. The values are the mean ± standard deviation of each treatment (n = 4). The different letters indicate significant differences (p < 0.05) in the enzyme activities under the different treatments.
Chemical properties of the litter
Compared with the control treatment, warming decreased the C concentrations, C/N, C/P, N/P, and phenolics of C. calyculata by 2.9 (p > 0.05), 16.6 (p > 0.05), 18.2 (p > 0.05), 1.0 (p > 0.05), and 22.1% (p > 0.05), respectively, and increased the N and P concentrations by 10.8 (p > 0.05) and 11.9% (p > 0.05), respectively (Table 3). The warming effects on the chemical properties differed under the different litter positions. Warming significantly increased the N and P concentrations of C. calyculata (p < 0.05) and significantly decreased the C/N, C/P, and phenolics of C. calyculata in the no-mixing treatment (p < 0.05), whereas significant responses were not observed in the soil-litter mixing treatment (Table 3). Additionally, warming did not significantly alter the C concentrations of C. calyculata in the no-mixing treatment (p > 0.05) but significant decreases were found in the soil-litter mixing treatment (p < 0.05; Table 3). Also, N/P did not show significant changes in response to warming in comparison to the control under both the no-mixing and soil-litter mixing treatments (p > 0.05; Table 3).
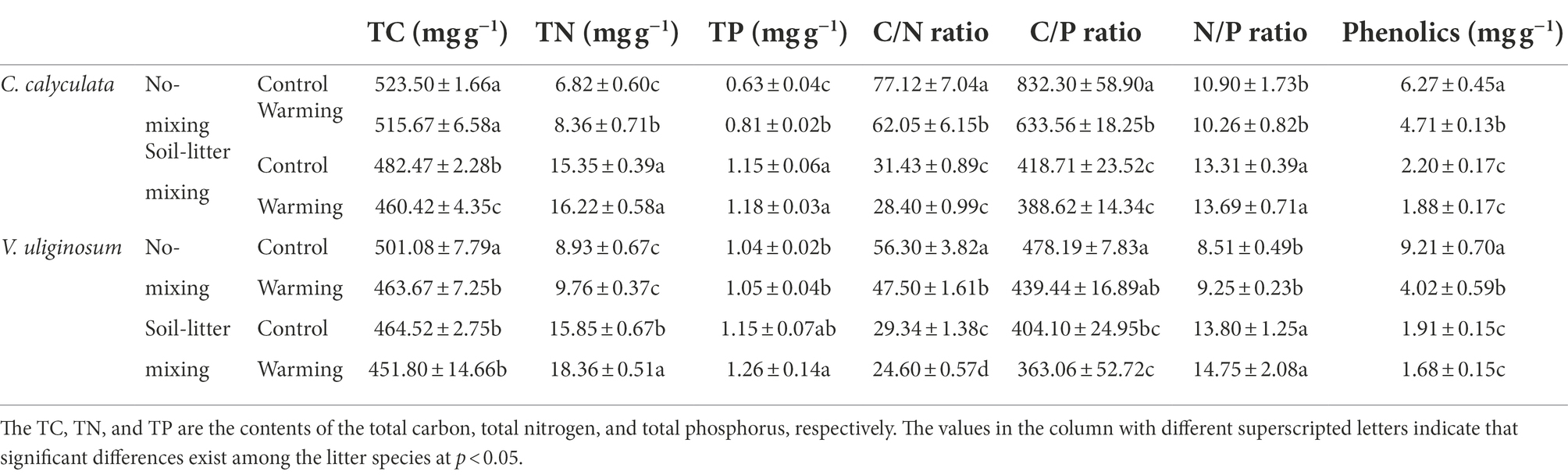
Table 3. The effects of warming and the litter positions on the TC, TN, TP, and phenolics contents of the C. calyculata and V. uliginosum litter.
The C concentrations, C/N, C/P, and phenolics of V. uliginosum were, on average, 5.1 (p > 0.05), 15.8 (p > 0.05), 9.0 (p > 0.05), and 48.6% lower (p > 0.05), and the N concentrations, P concentrations, and N/P were 13.5 (p > 0.05), 5.2 (p > 0.05), and 7.5% (p > 0.05) higher, respectively, in the warmed subplots than those in the control subplots (Table 3). Overall, the responses of the C and N concentrations and phenolics to warming varied with the litter positions. Additionally, warming did not induce significant changes in the P, C/P, and N/P values when compared to those of the control in both the no-mixing and soil-litter mixing treatments (p > 0.05), whereas warming significantly decreased C/N relative to that of the control under both litter positions (p < 0.05; Table 3).
Impacts of the enzyme activities and litter chemistries on the CO2 emission rate
We used a PCA and relative weight analysis to explore the correlation between the CO2 emission rate and soil enzymes and litter chemistries. Our analyses showed that the enzyme activities of βG, βX, and CBH were positively and significantly correlated with the average rate of CO2 emission from C. calyculata but these significant correlations were not observed for the other variables (Figure 4). More than 60.0% of the variability in the CO2 emissions from C. calyculata can be explained by the enzyme activities of βG and CBH when using the relative weight analysis (Figure 4). In contrast to C. calyculata, laccase and the phenolic compounds exerted significant negative effects on the CO2 emission rate of V. uliginosum, and the remaining chemical indicators were not significant (Figure 4). The relative contributions of laccase and the phenolic compounds to the CO2 emissions of V. uliginosum were 35.2 and 38.8%, respectively (Figure 4).
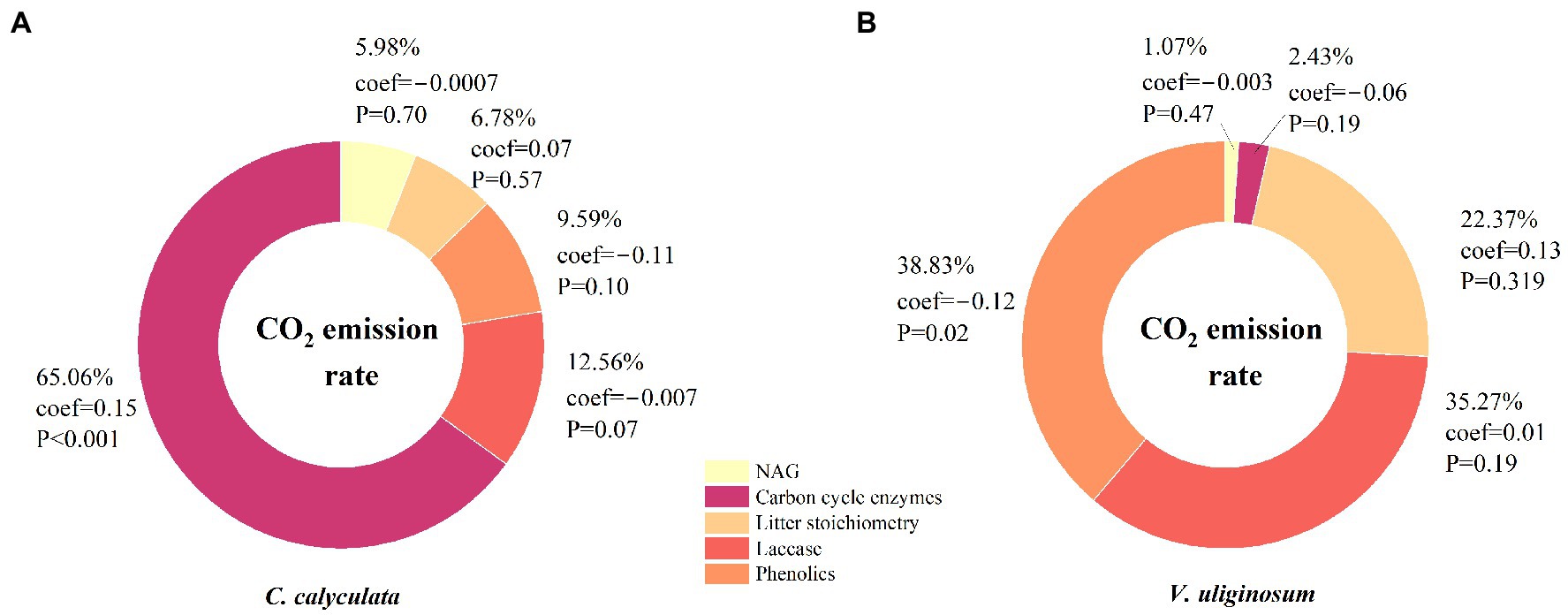
Figure 4. The relative weights of the effects of the soil enzyme activity and litter chemistry on the rate of CO2 emissions from the C. calyculata (A) and V. uliginosum (B) litter under the different treatments. A principal component analysis (PCA) was used, resulting in five explanatory variables: the litter stoichiometry, soil carbon cycle enzymes, soil nitrogen cycle enzymes, laccase, and phenolics. The percentages show the relative weight of these variables, and coef refers to the standardized regression coefficient. The p-values represent the effects of the explanatory variables on the CO2 emission rates.
Discussion
It is commonly reported that litter decomposition responds to climate warming and its underlying mechanisms when litter is located on the soil surface (Hough et al., 2022; Yang et al., 2022; Zhang, X. H. et al., 2022). However, this is not always the case. The litter can be mixed into the soil due to wind and water movement with changes in the peat soil porosity under a warming climate (Kechavarzi et al., 2010; Lee et al., 2014). Studies have shown that the response of CO2 emissions to soil-litter mixing differs from no-mixing (Hewins et al., 2013; Erdenebileg et al., 2018). However, in the northern peatland ecosystem, these differences were previously unobserved. Our study found that warming and litter positions greatly altered the CO2 emission rates of C. calyculata and V. uliginosum. More importantly, soil-litter mixing significantly restrained the warming-induced positive effects on the CO2 emission rates of C. calyculata and V. uliginosum when compared to no-mixing. The observed negative effects of the litter positions of C. calyculata were stronger than those of V. uliginosum. These response processes are mainly attributed to the chemical properties of the litter and microcosm soil enzyme activities (Figure 5).
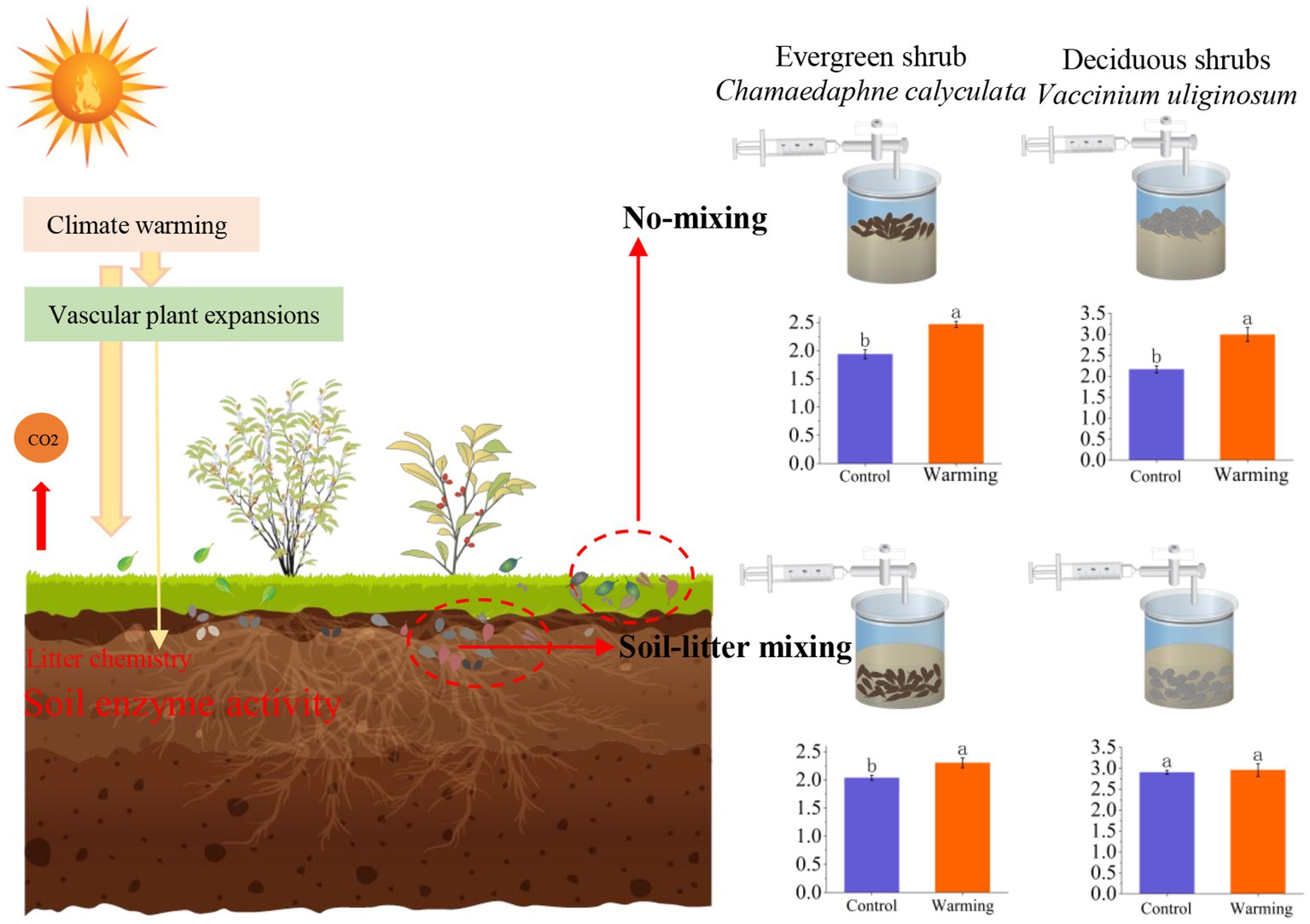
Figure 5. A mechanism framework for the response of leaf litter decomposition to warming at different positions. The two plants in the left half of the figure represent plants C. calyculata and V. uliginosum. The two red dashed boxes, from left to right, represent litter mixed with soil (Soil-litter mixing) and litter on the soil surface (No-mixing), respectively. The bar graphs in the right half of the figure shows the average CO2 emission rate throughout the incubation period, under the different treatments (the different letters in the insets represent statistical differences (p < 0.05) based on a Tukey’s multiple comparison test).
Evergreen shrub litter in response to warming and the litter positions
The variability in the CO2 emission rates of C. calyculata depended on the enzymatic activities. It has been commonly observed that warming significantly accelerates litter decomposition (Mao et al., 2018; Zhang et al., 2019) and increases CO2 release (Gao et al., 2022). Similarly, we found that the CO2 emission rate of the evergreen shrub was significantly increased by warming under the same litter positions. Increases in the CO2 emission rates in response to warming could be attributed to the increased microbial abundance and enzymatic activities of CBH and βG (Meyer et al., 2022). Warming can influence enzymatic activities in two different ways. First, higher temperatures can enhance soil microbial activity and biomass by accelerating the rate of microbial metabolism (Chen et al., 2015). Second, decreasing labile C with time at higher temperatures leads to increasing C limitations for the microorganisms, resulting in the production of more C-acquiring enzymes at later stages of initiation.
When compared to the no-mixing treatment, soil-litter mixing significantly decreased the sensitivity of the CO2 emission rate of C. calyculata’s litter to warming. This negative effect could be because of decreased enzymatic activities driven by the litter water content under soil-litter mixing (Zhao et al., 2021). Our study showed that warming significantly reduced the moisture content of the litter by 28.3% when compared to that of the control in the no-mixing treatment but no significant changes were observed in the soil-litter mixing treatment (Figure 6). This could lead to decreases in the sensitivity of βG and CBH to warming with soil-litter mixing relative to no-mixing, supporting the findings of previous studies. Microorganisms in relatively dry litter need to excrete more enzymes to obtain sufficient resources to compensate for the moisture-limiting effects of warming (Allison et al., 2010). Furthermore, water limitations can increase the fungal biomass and, in turn, exert strong impacts on enzyme activities, especially in terms of degrading more complex polymers (e.g., cellulose; Schneider et al., 2012). Thus, when compared to no-mixing, soil-litter mixing decreased the response of βG and CBH to warming, and, thus, the sensitivity of the rates of the CO2 emissions to warming under no-mixing was higher than that under soil-litter mixing.
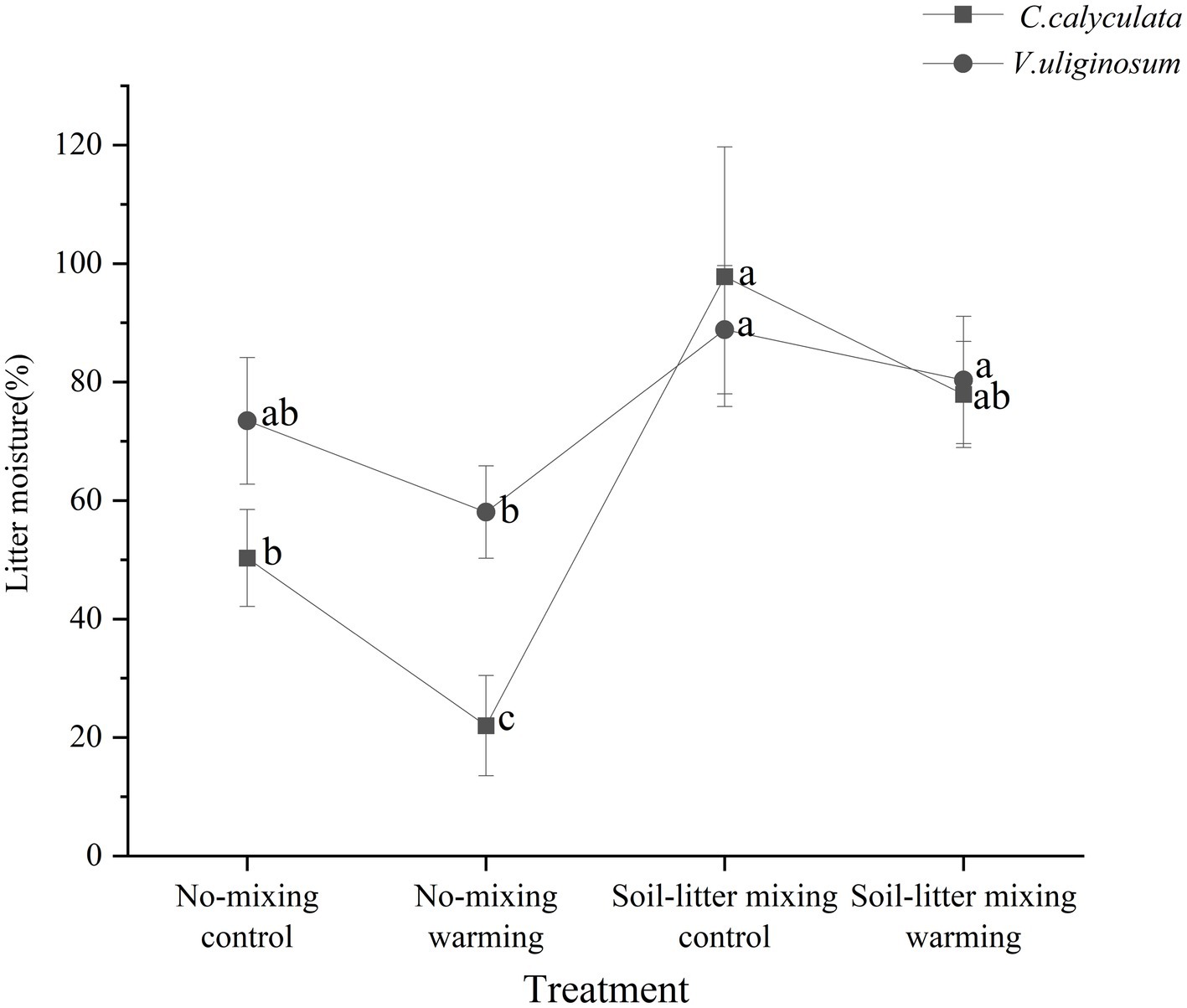
Figure 6. The moisture content of C. calyculata (square) and V. uliginosum (circle) litter at the end of the incubation. The letters represent significant differences among the four treatments (Bonferroni multiple comparison tests, p < 0.05). The error bars indicate the standard deviation.
Deciduous shrub litter in response to warming and the litter positions
The response of the litter decomposition of V. uliginosum to warming was attributable to changes in the soluble phenolic compounds. In the peatlands, the concept of “enzyme latch” is believed to be the main constraint of carbon decomposition. The lack of oxygen in water-saturated peat inhibits phenol oxidase activity, and, thus, leads to the accumulation of soluble phenolic compounds in the peatland, which, in turn, interact with proteins and inhibit microbial activity (Freeman et al., 2001, 2004). In this study, the relative weight was used to determine that the soluble phenolic compounds play an important role in driving changes in the CO2 emission rate. Further, a significant negative correlation was found between the phenolic compound concentrations and CO2 emission rates, which is consistent with the findings of Fenner and Freeman (2011) in peatland ecosystems. The results show that warming significantly reduces the phenolic concentration of V. uliginosum’s litter, resulting in higher CO2 emissions.
The response of V. uliginosum’s litter decomposition to warming varied with the litter positions. Under the no-mixing treatment, warming significantly decreased the CO2 emissions rate by 38.8% but an insignificant decrease of 1.9% was observed under-litter mixing. Laccase and the phenolic compounds were important factors influencing the decomposition of V. uliginosum’s litter (Zhao et al., 2019; Fenner and Freeman, 2020). The oxidase activities are limited by oxygen under soil-litter mixing (Ellis et al., 2009), partly counteracting the positive response of laccase activity to warming. Thus, compared with no-mixing, warming did not significantly increase laccase activities under the soil-litter mixing treatment, in turn, leading to a stronger response of the phenolic compounds to warming in the no-mixing treatment than that in the soil-litter mixing treatment.
Differences in the CO2 emission rates between the species
Litter quality is considered to be an important factor that directly affects the decomposition rate at the regional and global scales (Aerts, 1997). It is commonly accepted that litter with high N concentrations and low C/N is easily decomposed (Hong et al., 2021). The tissue N content is a major factor that controls the rate of litter decomposition on a global scale, and the rate of decay of the soil organic matter is usually positively correlated with the effectiveness of N (Zhang et al., 2008). Decomposers tend to increase following relatively strict stoichiometric requirements (Cleveland and Liptzin, 2007), and higher litter quality (low C/N) will support a higher total microbial biomass and, correspondingly, have more bacteria (Bray et al., 2012). In high N litter, more resources may be allocated to C-acquiring enzymes such as cellulases, resulting in higher C decomposition rates in low C/N litter (Tan et al., 2020). In this study, we found that the deciduous shrub of V. uliginosum had higher N content and lower C/N than those of the evergreen shrub C. calyculata. These differences resulted in the CO2 emission rate of V. uliginosum being significantly higher than that of C. calyculata. Similarly, the observations of the decomposition of two dominant plants in a typical alpine tundra ecosystem showed that low N content and high C/N resulted in lower decomposition rates and soil respiration (Zhang, Y. J. et al., 2022).
Conclusion
Based on a 124-day incubation experiment, this study focused on the differences in the response of evergreen and deciduous shrub litter to warming, litter positions, and their interactions. Warming and litter positions significantly altered the CO2 emission rates of C. calyculata and V. uliginosum. More importantly, soil-litter mixing significantly restrained the warming-induced positive effects on the CO2 emission rates of C. calyculata and V. uliginosum when compared to no-mixing. The observed negative effects of C. calyculata were stronger than those of V. uliginosum. Exploring different plant leaf litter in peatland ecosystems in response to warming and decomposition positions may help broaden our understanding of the contribution of peatland ecosystems to terrestrial C sinks. There is also a need to expand this work from the laboratory to the field to confirm these laboratory-based findings in future research.
Data availability statement
The original contributions presented in the study are included in the article/supplementary material, further inquiries can be directed to the corresponding authors.
Author contributions
XW developed the theoretical consideration and planned the study. YD and JJ were responsible for the sample collection. XW and XS provides funding. GM wrote the manuscript. All authors contributed to reviewing the analyses and the final text.
Funding
The work was supported by the National Natural Science Foundation of China no. 41971143, 42271111 and 41861134029.
Acknowledgments
We wish to acknowledge Zhenling Gao for the field work and Jinli Gao for the laboratory analyses.
Conflict of interest
The authors declare that the research was conducted in the absence of any commercial or financial relationships that could be construed as a potential conflict of interest.
The AE IBN and RE1 GZ declared a shared affiliation with the authors XW and YD at the time of review.
Publisher’s note
All claims expressed in this article are solely those of the authors and do not necessarily represent those of their affiliated organizations, or those of the publisher, the editors and the reviewers. Any product that may be evaluated in this article, or claim that may be made by its manufacturer, is not guaranteed or endorsed by the publisher.
References
Aerts, R. J. O. (1997). Climate, leaf litter chemistry and leaf litter decomposition in terrestrial ecosystems: A triangular relationship. Oikos 79, 439–449. doi: 10.2307/3546886
Aerts, R. (2006). The freezer defrosting: Global warming and litter decomposition rates in cold biomes. J. Ecol. 94, 713–724. doi: 10.1111/j.1365-2745.2006.01142.x
Allison, S. D., Wallenstein, M. D., and Bradford, M. A. (2010). Soil-carbon response to warming dependent on microbial physiology. Nat. Geosci. 3, 336–340. doi: 10.1038/ngeo846
Bärlocher, F., Gessner, M. O., and Garca, M. (2020). Methods to study litter decomposition: A practical guide. Dordrecht: Springer.
Boyero, L., Pearson, R. G., Gessner, M. O., Barmuta, L. A., Ferreira, V., Graça, M. A., et al. (2011). A global experiment suggests climate warming will not accelerate litter decomposition in streams but might reduce carbon sequestration. Ecol. Lett. 14, 289–294. doi: 10.1111/j.1461-0248.2010.01578.x
Bray, S. R., Kitajima, K., and Mack, M. C. (2012). Temporal dynamics of microbial communities on decomposing leaf litter of 10 plant species in relation to decomposition rate. Soil Biol. Biochem. 49, 30–37. doi: 10.1016/j.soilbio.2012.02.009
Butenschoen, O., Scheu, S., and Eisenhauer, N. (2011). Interactive effects of warming, soil humidity and plant diversity on litter decomposition and microbial activity. Soil Biol. Biochem. 43, 1902–1907. doi: 10.1016/j.soilbio.2011.05.011
Cardenas, R. E., Hattenschwiler, S., Valencia, R., Argoti, A., and Dangles, O. (2015). Plant herbivory responses through changes in leaf quality have no effect on subsequent leaf-litter decomposition in a neotropical rain forest tree community. New Phytol. 207, 817–829. doi: 10.1111/nph.13368
Chen, J., Luo, Y. Q., Xia, J. Y., Jiang, L. F., Zhou, X. H., Lu, M., et al. (2015). Stronger warming effects on microbial abundances in colder regions. Sci. Rep. 5:18032. doi: 10.1038/srep18032
Chen, N., Song, C. C., Xu, X. F., Wang, X. W., Cong, N., Jiang, P. P., et al. (2021). Divergent impacts of atmospheric water demand on gross primary productivity in three typical ecosystems in China. Agric. For. Meteorol. 307:108527. doi: 10.1016/j.agrformet.2021.108527
Cleveland, C. C., and Liptzin, D. (2007). C: N: P stoichiometry in soil: is there a “Redfield ratio” for the microbial biomass? Biogeochemistry 85, 235–252. doi: 10.1007/s10533-007-9132-0
Corteselli, E. M., Burtis, J. C., Heinz, A. K., and Yavitt, J. B. (2017). Leaf litter fuels Methanogenesis throughout decomposition in a forested peatland. Ecosystems 20, 1217–1232. doi: 10.1007/s10021-016-0105-9
Dieleman, C. M., Branfireun, B. A., McLaughlin, J. W., and Lindo, Z. (2015). Climate change drives a shift in peatland ecosystem plant community: Implications for ecosystem function and stability. Globl. Change Biol. 21, 388–395. doi: 10.1111/gcb.12643
Eichlerova, I., Snajdr, J., and Baldrian, P. (2012). Laccase activity in soils: Considerations for the measurement of enzyme activity. Chemosphere 88, 1154–1160. doi: 10.1016/j.chemosphere.2012.03.019
Ellis, T., Hill, P. W., Fenner, N., Williams, G. G., Godbold, D., and Freeman, C. (2009). The interactive effects of elevated carbon dioxide and water table draw-down on carbon cycling in a Welsh ombrotrophic bog. Ecol. Eng. 35, 978–986. doi: 10.1016/j.ecoleng.2008.10.011
Elmendorf, S. C., Henry, G. H. R., Hollister, R. D., Bjork, R. G., Boulanger-Lapointe, N., Cooper, E. J., et al. (2012). Plot-scale evidence of tundra vegetation change and links to recent summer warming. Nat. Clim. Chang. 2, 453–457. doi: 10.1038/nclimate1465
Erdenebileg, E., Ye, X. H., Wang, C. W., Huang, Z. Y., Liu, G. F., and Cornelissen, J. H. C. (2018). Positive and negative effects of UV irradiance explain interaction of litter position and UV exposure on litter decomposition and nutrient dynamics in a semi-arid dune ecosystem. Soil Biol. Biochem. 124, 245–254. doi: 10.1016/j.soilbio.2018.06.013
Fenner, N., and Freeman, C. (2011). Drought-induced carbon loss in peatlands. Nat. Geosci. 4, 895–900. doi: 10.1038/ngeo1323
Fenner, N., and Freeman, C. (2020). Woody litter protects peat carbon stocks during drought. Nature. Climate Change 10:363. doi: 10.1038/s41558-020-0727-y
Ferreira, V., Chauvet, E., and Canhoto, C. (2015). Effects of experimental warming, litter species, and presence of macroinvertebrates on litter decomposition and associated decomposers in a temperate mountain stream. Can. J. Fish. Aquat. Sci. 72, 206–216. doi: 10.1139/cjfas-2014-0119
Freeman, C., Fenner, N., Ostle, N. J., Kang, H., Dowrick, D. J., Reynolds, B., et al. (2004). Export of dissolved organic carbon from peatlands under elevated carbon dioxide levels. Nature 430, 195–198. doi: 10.1038/nature02707
Freeman, C., Ostle, N., and Kang, H. (2001). An enzymic ‘latch’ on a global carbon store - a shortage of oxygen locks up carbon in peatlands by restraining a single enzyme. Nature 409:149. doi: 10.1038/35051650
Gao, S. Q., Song, Y. Y., Song, C. C., Wang, X. W., Ma, X. Y., Gao, J. L., et al. (2022). Effects of temperature increase and nitrogen addition on the early litter decomposition in permafrost peatlands. Catena 209:105801. doi: 10.1016/j.catena.2021.105801
Hewins, D. B., Archer, S. R., Okin, G. S., McCulley, R. L., and Throop, H. L. (2013). Soil-litter mixing accelerates decomposition in a Chihuahuan Desert grassland. Ecosystems 16, 183–195. doi: 10.1007/s10021-012-9604-5
Hewins, D. B., Sinsabaugh, R. L., Archer, S. R., and Throop, H. L. (2017). Soil-litter mixing and microbial activity mediate decomposition and soil aggregate formation in a sandy shrub-invaded Chihuahuan Desert grassland. Plant Ecol. 218, 459–474. doi: 10.1007/s11258-017-0703-4
Hong, J. T., Lu, X. Y., Ma, X. X., and Wang, X. D. (2021). Five-year study on the effects of warming and plant litter quality on litter decomposition rate in a Tibetan alpine grassland. Sci. Total Environ. 750:142306. doi: 10.1016/j.scitotenv.2020.142306
Hough, M., McCabe, S., Vining, S. R., Pedersen, E. P., Wilson, R. M., Lawrence, R., et al. (2022). Coupling plant litter quantity to a novel metric for litter quality explains C storage changes in a thawing permafrost peatland. Globl. Change Biol. 28, 950–968. doi: 10.1111/gcb.15970
Kechavarzi, C., Dawson, Q., and Leeds-Harrison, P. B. (2010). Physical properties of low-lying agricultural peat soils in England. Geoderma 154, 196–202. doi: 10.1016/j.geoderma.2009.08.018
LeBreton, J. M., and Tonidandel, S. (2008). Multivariate relative importance: extending relative weight analysis to multivariate criterion spaces. J. Appl. Psychol. 93, 329–345. doi: 10.1037/0021-9010.93.2.329
Lee, H., Fitzgerald, J., Hewins, D. B., McCulley, R. L., Archer, S. R., Rahn, T., et al. (2014). Soil moisture and soil-litter mixing effects on surface litter decomposition: A controlled environment assessment. Soil Biol. Biochem. 72, 123–132. doi: 10.1016/j.soilbio.2014.01.027
Liu, G. D., Sun, J. F., Tian, K., Xiao, D. R., and Yuan, X. Z. (2017). Long-term responses of leaf litter decomposition to temperature, litter quality and litter mixing in plateau wetlands. Freshw. Biol. 62, 178–190. doi: 10.1111/fwb.12860
Luan, J. W., Wu, J. H., Liu, S. R., Roulet, N., and Wang, M. (2019). Soil nitrogen determines greenhouse gas emissions from northern peatlands under concurrent warming and vegetation shifting. Commun. Biol. 2:132. doi: 10.1038/s42003-019-0370-1
Mao, R., Zhang, X. H., Song, C. C., Wang, X. W., and Finnegan, P. M. (2018). Plant functional group controls litter decomposition rate and its temperature sensitivity: An incubation experiment on litters from a boreal peatland in Northeast China. Sci. Total Environ. 626, 678–683. doi: 10.1016/j.scitotenv.2018.01.162
McLaren, J. R., Buckeridge, K. M., van de Weg, M. J., Shaver, G. R., Schimel, J. P., and Gough, L. (2017). Shrub encroachment in Arctic tundra: Betula nana effects on above- and belowground litter decomposition. Ecology 98, 1361–1376. doi: 10.1002/ecy.1790
Meyer, N., Xu, Y., Karjalainen, K., Adamczyk, S., Biasi, C., van Delden, L., et al. (2022). Living, dead, and absent trees-how do moth outbreaks shape small-scale patterns of soil organic matter stocks and dynamics at the subarctic mountain birch treeline? Globl. Change Biol. 28, 441–462. doi: 10.1111/gcb.15951
Moore, T., and Basiliko, N. (2006). “Decomposition in boreal peatlands,” in Boreal Peatland Ecosystems (Berlin: Springer-Verlag), 125–143.
Rinkes, Z. L., Sinsabaugh, R. L., Moorhead, D. L., Grandy, A. S., and Weintraub, M. N. (2013). Field and lab conditions alter microbial enzyme and biomass dynamics driving decomposition of the same leaf litter. Front. Microbiol. 4:260. doi: 10.3389/fmicb.2013.00260
Robertson, G. P., Wedin, D., Groffman, P., Blair, J., Holland, E., Nedelhoffer, K., et al. (1999). “Soil carbon and nitrogen availability. Nitrogen mineralization, nitrification and soil respiration potentials,” in Standard Soil Methods for Long-Term Ecological Research. eds. G. P. Robertson, C. S. Bledsoe, D. C. Coleman, and P. Sollins (New York: Oxford University Press Oxford University Press), 258–271.
Schneider, T., Keiblinger, K. M., Schmid, E., Sterflinger-Gleixner, K., Ellersdorfer, G., Roschitzki, B., et al. (2012). Who is who in litter decomposition? Metaproteomics reveals major microbial players and their biogeochemical functions. ISME J. 6, 1749–1762. doi: 10.1038/ismej.2012.11
Siegenthaler, A., Buttler, A., Bragazza, L., van der Heijden, E., Grosvernier, P., Gobat, J. M., et al. (2010). Litter- and ecosystem-driven decomposition under elevated CO2 and enhanced N deposition in a sphagnum peatland. Soil Biol. Biochem. 42, 968–977. doi: 10.1016/j.soilbio.2010.02.016
Sistla, S. A., Moore, J. C., Simpson, R. T., Gough, L., Shaver, G. R., and Schimel, J. P. (2013). Long-term warming restructures Arctic tundra without changing net soil carbon storage. Nature 497:615. doi: 10.1038/nature12129
Song, Y. Y., Song, C. C., Hou, A. X., Ren, J. S., Wang, X. W., Cui, Q., et al. (2018). Effects of temperature and root additions on soil carbon and nitrogen mineralization in a predominantly permafrost peatland. Catena 165, 381–389. doi: 10.1016/j.catena.2018.02.026
Song, Y., Zou, Y. C., Wang, G. P., and Yu, X. F. (2017). Altered soil carbon and nitrogen cycles due to the freeze-thaw effect: A meta-analysis. Soil Biol. Biochem. 109, 35–49. doi: 10.1016/j.soilbio.2017.01.020
Strakova, P., Penttila, T., Laine, J., and Laiho, R. (2012). Disentangling direct and indirect effects of water table drawdown on above- and belowground plant litter decomposition: Consequences for accumulation of organic matter in boreal peatlands. Globl. Change Biol. 18, 322–335. doi: 10.1111/j.1365-2486.2011.02503.x
Tan, X. P., Machmuller, M. B., Huang, F., He, J. H., Chen, J., Cotrufo, M. F., et al. (2020). Temperature sensitivity of ecoenzyme kinetics driving litter decomposition: the effects of nitrogen enrichment, litter chemistry, and decomposer community. Soil Biol. Biochem. 148:107878. doi: 10.1016/j.soilbio.2020.107878
Throop, H. L., and Archer, S. R. (2007). Interrelationships among shrub encroachment, land management, and litter decomposition in a semidesert grassland. Ecol. Appl. 17, 1809–1823. doi: 10.1890/06-0889.1
Yang, K., Zhu, J. J., Zhang, W. W., Zhang, Q., Lu, D. L., Zhang, Y. K., et al. (2022). Litter decomposition and nutrient release from monospecific and mixed litters: Comparisons of litter quality, fauna and decomposition site effects. J. Ecol. 110, 1673–1686. doi: 10.1111/1365-2745.13902
Yu, Z. C., Loisel, J., Brosseau, D. P., Beilman, D. W., and Hunt, S. J. (2010). Global peatland dynamics since the last glacial maximum. Geophys. Res. Lett. 37:L13402. doi: 10.1029/2010gl043584
Zhang, D. Q., Hui, D. F., Luo, Y. Q., and Zhou, G. Y. (2008). Rates of litter decomposition in terrestrial ecosystems: global patterns and controlling factors. J. Plant Ecol. 1, 85–93. doi: 10.1093/jpe/rtn002
Zhang, Y. J., Jin, Y. H., Xu, J. W., He, H. S., Tao, Y., Yang, Z. P., et al. (2022). Effects of exogenous N and endogenous nutrients on alpine tundra litter decomposition in an area of high nitrogen deposition. Sci. Total Environ. 805:150388. doi: 10.1016/j.scitotenv.2021.150388
Zhang, Y., Song, C., Wang, X., Chen, N., Zhang, H., Du, Y., et al. (2022). Warming effects on the flux of CH4 from peatland mesocosms are regulated by plant species composition: Richness and functional types. Sci. Total Environ. 806:150831. doi: 10.1016/j.scitotenv.2021.150831
Zhang, X. H., Wang, X. W., Finnegan, P. M., Tan, W. W., and Mao, R. (2019). Effects of litter mixtures on aerobic decomposition rate and its temperature sensitivity in a boreal peatland. Geoderma 354:113890. doi: 10.1016/j.geoderma.2019.113890
Zhang, X. H., Zhang, Y. H., Jiang, S. S., Song, C. C., Zhang, J. B., and Mao, R. (2022). Dominant species and evenness level co-regulate litter mixture decomposition in a boreal peatland. Plant Soil 474, 423–436. doi: 10.1007/s11104-022-05346-z
Zhao, Y. P., Xiang, W., Yan, S., Huang, Y. B., and Fan, W. G. (2019). Laccase activity in sphagnum-dominated peatland: A study based on a novel measurement of delay dynamics (MDD) for determining laccase activity. Soil Biol. Biochem. 133, 108–115. doi: 10.1016/j.soilbio.2019.03.003
Keywords: boreal peatland, litter decomposition, soil-litter incubation, CO2 fluxes, enzyme activity
Citation: Ma G, Wang X, Sun X, Wang S, Du Y and Jiang J (2022) Effects of warming and litter positions on litter decomposition in a boreal peatland. Front. Ecol. Evol. 10:1078104. doi: 10.3389/fevo.2022.1078104
Edited by:
Ben Niu, Institute of Geographic Sciences and Natural Resources Research (CAS), ChinaReviewed by:
Guang Zhao, Institute of Geographic Sciences and Natural Resources Research (CAS), ChinaFeng Li, Institute of Subtropical Agriculture (CAS), China
Copyright © 2022 Ma, Wang, Sun, Wang, Du and Jiang. This is an open-access article distributed under the terms of the Creative Commons Attribution License (CC BY). The use, distribution or reproduction in other forums is permitted, provided the original author(s) and the copyright owner(s) are credited and that the original publication in this journal is cited, in accordance with accepted academic practice. No use, distribution or reproduction is permitted which does not comply with these terms.
*Correspondence: Xianwei Wang, wangxianwei@iga.ac.cn; Xiaoxin Sun, sunxiaoxin@nefu.edu.cn