- 1Department of Biochemistry, Institute of Cell Differentiation and Aging, College of Medicine, Hallym University, Chuncheon, Republic of Korea
- 2Biomedical Science Core-Facility, Soonchunhyang Institute of Medi-Bio Science, Soonchunhyang University, Cheonan, Republic of Korea
- 3Department of Biological Sciences, Sookmyung Women’s University, Seoul, Republic of Korea
- 4Department of Electrical Engineering, Hallym University, Chuncheon, Republic of Korea
Cellular aging is the most severe risk factor for neurodegenerative disease. Simultaneously, oxidative stress (OS) is a critical factor in the aging process, resulting from an imbalance between reactive oxygen and nitrogen species and the antioxidant defense system. Emerging evidence indicates that OS is a common cause of several age-related brain pathologies, including cerebrovascular diseases. Elevated OS disrupts endothelial functional ability by diminishing the bioavailability of nitric oxide (a vascular dilator), induces atherosclerosis, and impairs vasculature, which are all common characteristics of cerebrovascular disease. In this review, we summarize evidence supporting an active role of OS in cerebrovascular disease progression, focusing primarily on stroke pathogenesis. We briefly discuss hypertension, diabetes, heart disease, and genetic factors that are often linked to OS and are considered associated factors influencing stroke pathology. Finally, we discuss the current pharmaceutics/therapeutics available for treating several cerebrovascular diseases.
1 Introduction
Cerebrovascular diseases (CeVDs) are diverse and multifactorial diseases that include stenosis (stenosis of the carotid, vertebral stenosis, or intracranial stenosis) and aneurysms; these conditions generally result from the blockage (embolism) or rupture (hemorrhage) of blood vessels, ultimately leading to stroke (ischemic or hemorrhagic, respectively) (1–4). There are several well-known risk factors for stroke, including hypertension, obesity, high cholesterol, dyslipidemia, and diabetes; however, high levels of cellular reactive oxygen/nitrogen species (ROS/RNS) also contribute significantly to stroke/cardiovascular disease (CVD) pathogenesis. In healthy cells, ROS/RNS is maintained at a low concentration. It is important for normal cellular metabolism and contributes to normal physiological processes, including cell proliferation, differentiation, and apoptosis. However, elevated ROS/RNS, referred to as oxidative stress (OS), promotes many pathological conditions, including CVDs (2, 3). In the last decade, it has been repeatedly reported that increasing free radical activity plays an important role in the pathogenesis of vascular disorders, aging processes, and neurodegenerative diseases (5, 6).
As the brain is susceptible to OS, increasing amounts of ROS/RNS, impaired oxidants/antioxidant systems, and mitochondrial dysfunction together initiate neurodegenerative processes. In contrast, a lack of blood circulation (ischemia) to the brain (or any other region) limits the energy supply, which further elevates free radical concentrations and leads to neuron death (7). Additionally, a state of high OS induces several cellular and biochemical reactions, for example endothelial dysfunction, vasculitis (vascular inflammation), arterial remodeling, and blood–brain barrier impairment (8). Further consequences can include cerebral reperfusion injury, the blockage of cerebral blood flow, and cerebral bleeding or blood spillage. Considerable progress has been made in understanding brain functionality, and the importance of a balanced oxidant/antioxidant system for brain function is well-understood; however, how exactly elevated free radicals or OS cause CeVDs is ambiguous. Several studies have reported that ischemic stroke is a result of increased levels of cellular ROS, such as superoxide anions (O2−), hydrogen peroxide (H2O2), and hydroxyl radicals (OH−) (9–13). Here, we review the evidence on the contributions of free radicals/OS to stroke pathology. Additionally, we briefly discuss other associated factors and their relationships with stroke. Lastly, we review currently available pharmaceutic/therapeutic agents used to treat stroke.
2 Epidemiology of stroke
On average, approximately 85% of strokes are ischemic and 15% are hemorrhagic. Ischemic strokes can be classified into large-artery atherosclerosis, small-vessel occlusion, cardioembolism, or cryptogenic ischemic stroke, among others. Hemorrhagic strokes can be classified into two groups: intracerebral hemorrhage or subarachnoid hemorrhage (Figure 1) (13, 14). CeVD has a severe impact on a patient’s quality of life, often resulting in long-term disability or death. For this reason, CeVD is listed as the second leading cause of death worldwide (15, 16). In 2019, 6.6 million deaths were caused by CeVD worldwide. Of these, 3.3 million were attributable to ischemic stroke, 2.9 million to intracerebral hemorrhage, and 0.4 million to subarachnoid hemorrhage (13). A previous study demonstrated that a total of 12.2 million stroke incidents and 6.55 million resulting deaths were recorded globally between 1990 and 1990 (17). In the United States alone, more than 795,000 people suffer stroke annually, resulting in a mortality rate of 48.6 deaths per 100,000 persons (18). According to an estimate (Centers for Disease Control and Prevention, U.S.A.), a 20.5% increase in the number of adults in the United States who will experience stroke is expected by 2030. A recent study demonstrated that type 2 diabetes mellitus and hypertension increase stroke occurrence by about 12.1% in patients with no stroke history. Moreover, recurrence of stroke within the 1 year follow-up period increased by 26.5% in patients with a history of stroke (19). High rates of ischemic stroke have been reported in North America, Southeast Asia, southern sub-Saharan Africa, North Africa, and the Middle East. Intracerebral hemorrhage shows a high prevalence in Oceania and Southeast Asia, while subarachnoid hemorrhage is prevalent in high-income regions of the Asian Pacific, North America, parts of Eastern Europe, and Oceania (13).
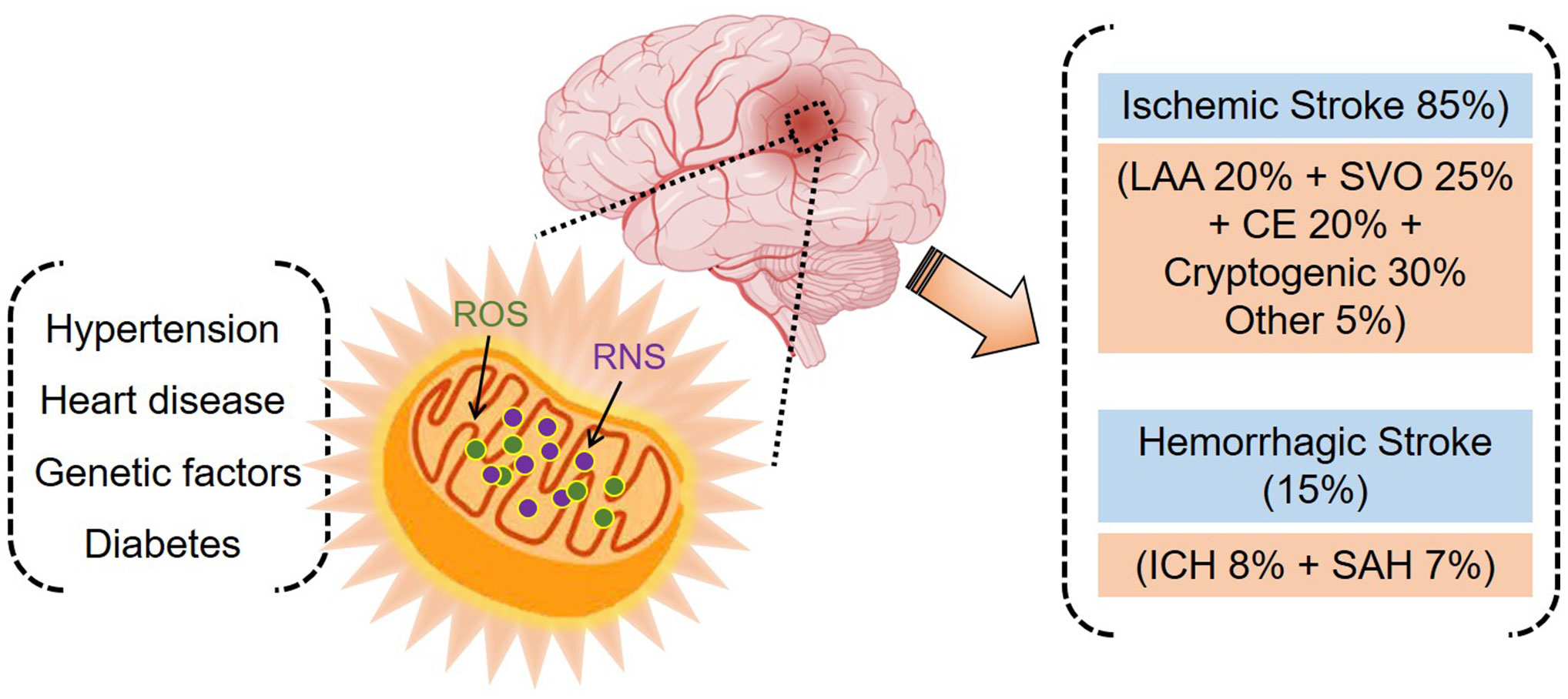
Figure 1 Stroke and associated risk factors. Oxidative stress plays a central role in stroke pathogenesis (ischemic and hemorrhagic) and is implicated in hypertension, heart disease, genetic factors, and diabetes.
3 Oxidative stress in the progression of stroke
3.1 Oxidants and the antioxidant defense system
In a healthy brain cell, up to 1% of mitochondrial electron flow generates O2−, which is consumed by a variety of superoxide dismutases (SOD1, SOD2, and SOD3) to produce H2O2 molecules (20). H2O2 is less toxic than O2− but can react to generate even more dangerous compounds; for example, H2O2 can generate hydroxyl radicals (−OH) by reacting with Fenton’s reagent, and peroxynitrite anions (ONOO–) by reacting with nitric oxide (11). Several components of the antioxidant defense system, such as glutathione (non-enzymatic), thioredoxin, α-tocopherol (vitamin E), carotenoids, and ascorbic acid (vitamin C), interact with ROS to detoxify cells. Furthermore, the critical intracellular antioxidant enzymes glutathione peroxidase-1 and catalase convert H2O2 to water (11, 21, 22). Failure of this antioxidant defense system is the leading cause of aging and neurodegeneration and has been reported in all types of stroke (23–26).
3.2 The compromised antioxidant defense system in stroke
Increased levels of OS (free radicals) in the brain lead to cerebrovascular and neurodegenerative diseases. The mechanism of OS elevation often results from a compromised antioxidant defense system. In the brain’s blood vessels, endothelial cells make up the innermost layer and are tightly surrounded by pericytes, followed by the basement membrane and astrocytic endfeet (27). Emerging evidence suggests that OS plays a central role in endothelial dysfunction and inflammation. The mechanism of ROS formation can be described as the blockage of cerebral blood flow that generates oxygen/glucose deficiency in neurons, with hypoxia ultimately triggering the decoupling of mitochondrial respiration (28, 29). This decoupling results in the production and accumulation of ROS and RNS products and the activation of xanthine oxidase. The increase in ROS/RNS products and ATP deficiency combine to initiate neurodegeneration and tissue oxygenation, leading to ischemia-reperfusion injury (28, 29). At the cellular level, OS can be measured by detecting the expression of crucial biomarkers (oxidative byproducts of different types of molecules); for example, DNA oxidation generates 8-hydroxydeoxyguanosine (8-OHdG), polyunsaturated fatty acid oxidation generates malondialdehyde (MDA), and lipid peroxidation generates 4-hydroxy-2-noneal (26, 30). Marker expression over a particular cellular concentration is generally estimated as an exalted or low state of OS. Several previous studies have demonstrated that patients with acute ischemic stroke express high levels of MDA (31–33). It is important to note, however, that MDA is not solely an OS marker; it is also a toxic molecule that can interact with DNA and protein and create mutagenic and atherogenic environments that can reduce cell survival (reviewed in (34)). Similarly, in a recent study, Liu Zhihua et al. established a relationship between high expression of the OS markers 8-OHdG and MDA and post-stroke cognitive impairment 1 month after acute ischemic stroke (35). The prolonged elevation of OS subsequently induces the accumulation of nitric oxide, an important vasodilator compound and a key player in many physiological processes (36), to a toxic level, causing inflammation and cytotoxicity and leading to mitochondrial dysfunction and neuronal death (25, 36). Additionally, previous studies have demonstrated that NADPH oxidase (NOX) plays a significant role in generating ROS in the brain. NOX gene deficiency or the inhibition of NOX activity significantly improves brain injury and neuronal function [reviewed in (37)].
In the antioxidant system, three SOD isoforms (SOD1, SOD2, and SOD3) scavenge ROS in different locations: SOD1 is cytoplasmic, SOD2 is located in the mitochondria, and SOD3 is extracellular. Combined, SOD1–3 establish the primary defense against ROS and functionally protect cells from free radicals and OS (38, 39). Recent studies indicate that SOD loss of function or genetic variation is associated with severe pathological diseases, including CVD and CeVDs (stroke) (10). In a previous study, six single-nucleotide polymorphisms (SNPs) within SOD genes were investigated to determine their association with ischemic stroke: rs17880487 and rs80265967 in SOD1, rs4880 and rs2842960 in SOD2, and rs2695232 and rs7655372 in SOD3. The SNP rs7655372 in the SOD3 gene was associated with an increased risk of ischemic stroke, and the SNP rs17880487 in the SOD1 gene was associated with an increased risk of cardiovascular mortality (10, 40). Furthermore, a mouse model with depletion of the key metabolic enzyme cystathionine beta-synthase or SOD1 developed hypertrophy without hypertension (41). Moreover, a cohort study demonstrated that deletion of 50 bp from the promoter region of SOD1 increased the risk of CVD and atherosclerotic heart disorder (42). Thus, taken together, these studies suggest that the absence or dysfunction of SOD1 disturbs the oxidant/antioxidant balance, leading to heart disease and CeVDs.
Similarly, Gpx-1, an intracellular antioxidant enzyme, reduces H2O2 to water and is implicated in neural cell viability in OS-induced neural degeneration. It has been reported that Gpx-1 knockout mice (Gpx-1−/−) exhibit a significant reduction in cerebral blood flow that induces susceptibility to cerebral injury and cerebral ischemia (43). However, it was previously reported that Gpx-1 knockout mice displayed a three-fold increase in infarct volume compared to normal mice. Moreover, pre-activation of the apoptosis marker, caspase 3, was reported in the stroke model, suggesting that the neuronal protective activity against the oxidative state and Gpx-1 deficiency may initiate the neuronal apoptosis program (43). This finding supports the importance of Gpx-1-mediated ROS scavenging, which is essential for neuronal viability, maintaining cerebral flow, and reducing microvascular perfusion that can lead to stroke.
It has been reported that oxidative/nitrosative stress is central to several neurodegenerative diseases (26). Gorg et al. (44) investigated the post-mortem cortical brain tissues of cirrhosis patients with or without hepatic encephalopathy (HE) and found that the HE brain expressed elevated levels of tyrosine-nitrated proteins, heat shock protein-27, and 8-hydroxyguanosine (a marker of RNA oxidation) with reduced activity of glutamine synthetase (44). This indicates that oxidative/nitrosative stress may be involved in HE pathogenesis, which is another risk factor for CeVDs. However, the authors did not discover an active association between oxidative/nitrosative stress and cirrhosis (44).
Additionally, hydrogen sulfide (H2S) is a gaseous signaling molecule that plays an important role in several physiological and pathophysiological processes and is a well-known essential protector against cellular OS (45). H2S is a marker of endothelial inflammation and dysfunction (reviewed in (46)), and its production in endothelial cells requires three key enzymes, cystathionine gamma-lyase (CSE), cystathionine beta-synthase, and 3-mercaptopyruvate sulfurtransferase. The CSE/H2S pathway has been reported to block atherosclerosis formation, and CSE-knockout mice exhibit severe atherosclerosis, supporting an anti-atherosclerosis role of H2S in the vascular system (45, 47). However, H2S also promotes antioxidant production by modifying the cysteine residues of upstream signaling molecules, including keap1/Nrf2, NFκB, and HIF-1α (45). Thus, H2S plays a dual role in the inhibition of atherosclerosis and induces the production of oxidant scavengers. Collectively, these findings indicate that compromised antioxidant defense systems or excessive ROS/RNS levels drive neuronal degeneration, which further leads to stroke or other brain-related diseases.
4 Oxidative stress and other factors associated with stroke
Risk factors often associated with CeVDs (stroke) are generally divided into two groups: lifestyle-related risk factors and health/medical risk factors. Lifestyle-related risk factors may include a lack of physical exercise, heavy drinking, obesity, and excessive use of drugs (such as cocaine). Health/medical risk factors include high blood pressure, high cholesterol, diabetes, CVDs, and a family history of CeVDs or genetic background. This section briefly summarizes the four common medical risk factors, in which OS is a common pathogenetic link.
4.1 Hypertension
High blood pressure can functionally or structurally affect the arteries of the body; this is known as hypertension. In a healthy adult, the normal blood pressure range is systolic ≤120 mmHg and diastolic ≤80 mmHg (120/80 mmHg). Hypertension is diagnosed when blood pressure increases to 130/90 mmHg (48). Hypertension is the number one risk factor for stroke, accounting for over 50% of ischemic and 70% of hemorrhagic stroke incidents, and is also a leading cause of dementia (35). Primarily, hypertension alters the structural and functional capabilities of cerebral blood vessels via hypertrophic and eutrophic remodeling. Hypertrophic remodeling triggers hypertrophy or hyperplasia of smooth muscles in an artery, leading to wall thickening and narrowing of the lumen space, further impairing essential blood supply (based on new guidelines from the American Heart Association, 2017) (41). In addition, hypertension initiates fibrinoid necrosis (lipohyalinosis) of the lenticulostriate arteries and causes intracerebral bleeding or hemorrhage (49). Increasing evidence supports the strong involvement of OS in hypertension and its deleterious effects on individuals’ health, as OS markers are elevated in hypertension (renovascular or malignant) and preeclampsia (12, 41, 49).
4.2 Diabetes
Diabetes mellitus (DM), a chronic metabolic disease caused by insulin resistance, leads to elevated blood glucose levels. An average of 20–40% of patients with diabetes have CeVDs, with asymptomatic cerebral atherosclerosis being among the most common (50). It has been reported that human patients and animal models of DM (type 1 or 2) exhibit accelerated atherosclerosis (51). The coronary plaque in DM patients contains high macrophage counts and larger necrotic core areas compared with non-DM subjects, which is similar to the high rate of calcification in DM patients (51). Similarly, these observations have been further validated in a mouse model that lacks the insulin receptor (−/−) in macrophages, which caused the progression of necrotic cores to advanced plaques (52). The larger size of necrotic cores reduces macrophage efferocytosis in obese (ob/ob) mouse models of DM (53) (see (51, 54) for targeted articles on the connections of diabetes in the pathogenesis of atherosclerosis). Thus, evidence suggests that DM leads to faster progression of atherosclerosis and promotes CeVD pathology.
4.3 Heart diseases
CVD is a major cause of death and a major risk factor for human health worldwide (55). Moreover, CVD has been a leading cause of death among noncommunicable diseases in the last two decades, causing 17.9 million deaths in 2019 (55). Several heart-related problems, such as atrial fibrillation, ischemic heart disease, cardioembolic infarction, vascular peripheral disease, chronic obstructive pulmonary disease, and atherothrombotic infarction, are strongly associated with stroke (or stroke subtypes) (56). This topic has been discussed in detail in (13, 39, 54–57).
4.4 Genetic factors
Identifying hereditary (genetic) factors underlying stroke pathology is essential to deciphering the molecular mechanism. In recent years, progress has been made with modern genotyping technologies. French et al. (58) postulated the involvement of the PITX2 locus in stroke pathogenesis; indeed, Pitx2−/− mice exhibited a severe reduction in smooth muscle in cerebral vessels with increased vessel density. Similarly, the ABO locus has been associated with cardioembolic ischemic stroke (59). Several genetic factors have been identified that contribute to stroke risk at different levels, such as small-artery disease, large-artery atheroma, cardioembolism, hypertension, and dyslipidemia (60). For further reading, we direct readers to targeted articles in which known genetic factors have been classified and summarized (60, 61).
5 Diagnosis and treatment
The timely management of acute stroke after onset and selecting appropriate diagnostic/treatment methods are extremely important factors in stabilizing the patient. The accurate and timely recognition of stroke symptoms and first aid intervention can have a substantial impact on the outcome of the patient, potentially reducing mortality and stroke severity (62, 63). Several medical techniques have been developed to diagnose severe brain diseases, including stroke. For rapid clinical assessment, advanced brain scanning/imaging or neuroimaging tools are promising and required for precise and final confirmation of stroke after primary symptoms and blood analysis. Neuroimaging helps physicians develop timely treatment/surgery strategies. The most common neuroimaging technique is computed tomography (CT); CT scans can show subtle changes in cytotoxic edema, alterations in the gray-white matter, the hypoattenuation of basal ganglion, swelling, and blood vessel occlusion (64, 65). Advanced CT perfusion requires bolus administration (intravenous injection) to enhance the tissue visualization output. In addition to CT, magnetic resonance imaging or its more advanced version, magnetic resonance perfusion, are also commonly used; we direct readers to (66) for a review on this topic.
Modern treatment modalities play an important role in the management and treatment of stroke. There are several strategies to prevent or cure stroke in patients. The most effective way to prevent CeVDs is to control associated diseases, including hypertension, diabetes, and heart disease (67). Presently available drugs are classified into five groups based on molecular mechanisms and have overall effects on inhibiting vascular thrombosis, improving vascular function (or reducing tone), and lowering blood lipid concentration; some common drug names are shown in Figure 2 (68). As most of these drugs mechanistically act on blood flow, they may have several side effects; for example, antiplatelet aggregation can cause severe bleeding problems (69). Targeting free radicals by inhibiting their production is an effective strategy. The ideal targets are ROS-producing enzymes; for example, administration of allopurinol (metabolite; oxypurinol) to target xanthine oxidase and NS-398 and nimesulide to target cyclooxygenase2 (COX2) produced neuroprotective effects in transient and permanent ischemia (reviewed in (70)). However, herbal medicines (derived from natural products) offer alternative stroke treatment or prevention options, typically with fewer side effects (71). Currently, a wide variety of herbs, including Astragalus membranaceus, Angelica sinensis, Ligusticum chuanxiong, Paeonia lactiflora, Prunus persica, and Carthamus tinctorius, may be effective against stroke and CeVDs as they exhibit solid antioxidant, anti-inflammatory, anti-apoptosis, and other neuroprotective properties (71–73). Bioactive compounds isolated from natural sources have been reported to reduce OS via many pathways. For example, leonurine (from Leonotis leonurus) and cornin (from Cornus florida) induced SOD1 and GPx activity, resulting in deceased ROS levels in a stroke model [reviewed in (74)]. Additionally, in a recent study, Deng et al. (75) investigated the neuroprotective activity of leonurine (an alkaloid compound) against the OS and nitric oxide/NOS pathways. PC12 cells treated with leonurine showed a significant reduction in OS (measured via MDA expression) and improved activity of the ROS scavenger enzymes SOD and GSH, indicating that leonurine may be a promising natural option for protecting neurons against OS.
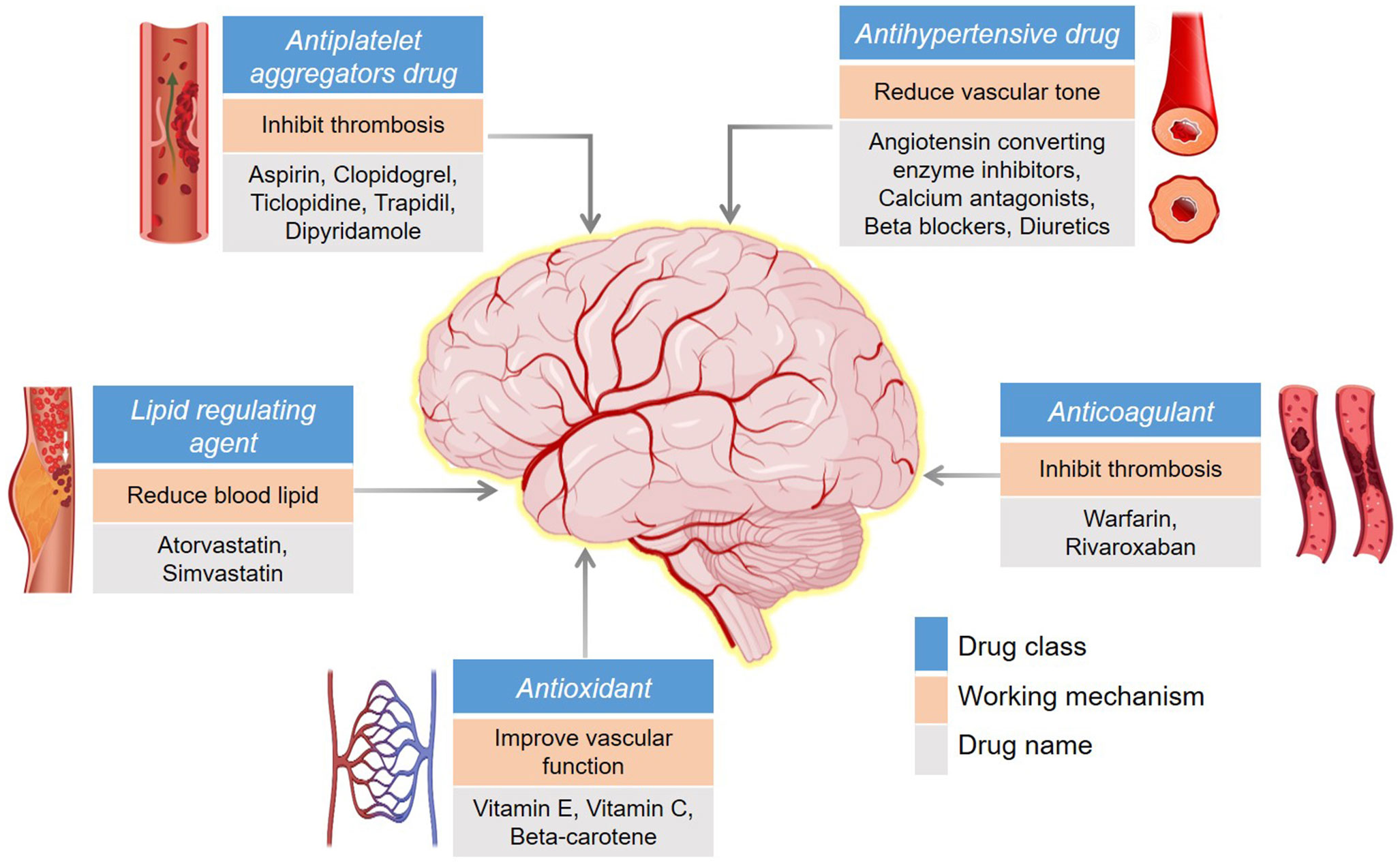
Figure 2 Common drugs for the prevention/treatment of stroke. The background color differentiates the drug class, working mechanism, and drug name as indicated.
6 Conclusions and prospects
The prevalence of CeVDs is increasing every year, including in younger populations, and thus demands urgent and effective treatment and prevention strategies. The occurrence of different metabolic diseases, CVDs, and hypertension significantly increases the life-long disability and death rates. Therefore, targeting a common cause may represent the most effective way to address this health issue worldwide. In the present review, we have discussed OS as a common root cause of pleotropic effects in several life-threatening illnesses, including stroke.
Significant progress has been made in understanding the mechanisms and molecular pathways that switch on ROS/RNS production or negatively regulate the antioxidant defense system. Over the past couple of decades, several mechanisms have been discovered to re-establish the antioxidant defense system and cellular homeostasis using different drugs or genetic manipulations. However, the exact molecular markers or diagnostic techniques used to detect mitochondrial dysfunction or OS at an early stage (before the onset of a severe condition) remain elusive. Further research efforts are necessary to develop accurate and effective treatment and prevention strategies for stroke and other diseases.
Author contributions
VK: Original draft preparation. KB: Review and editing. SP, UL and JK: Supervision. All authors have read and agreed to the published version of the manuscript. All authors contributed to the article and approved the submitted version.
Funding
This study was supported by the Basic Science Research Program of the National Research Foundation of Korea (NRF), funded by the Ministry of Education, Science, and Technology of Korea (2016R1D1A1B02008770, 2018M3C7A1056285, 2021R1A4A1027355, 2021M3H9A1097557, and 2022R1I1A3066646).
Conflict of interest
The authors declare that the research was conducted in the absence of any commercial or financial relationships that could be construed as a potential conflict of interest.
Publisher’s note
All claims expressed in this article are solely those of the authors and do not necessarily represent those of their affiliated organizations, or those of the publisher, the editors and the reviewers. Any product that may be evaluated in this article, or claim that may be made by its manufacturer, is not guaranteed or endorsed by the publisher.
References
1. Antognelli C, Perrelli A, Armeni T, Nicola Talesa V, Retta SF. Dicarbonyl stress and s-glutathionylation in cerebrovascular diseases: A focus on cerebral cavernous malformations. Antioxidants (Basel) (2020) 9:124. doi: 10.3390/antiox9020124
2. Chrissobolis S, Miller AA, Drummond GR, Kemp-Harper BK, Sobey CG. Oxidative stress and endothelial dysfunction in cerebrovascular disease. Front Biosci (Landmark Ed) (2011) 16:1733–45. doi: 10.2741/3816
3. Retta SF, Glading AJ. Oxidative stress and inflammation in cerebral cavernous malformation disease pathogenesis: Two sides of the same coin. Int J Biochem Cell Biol (2016) 81:254–70. doi: 10.1016/j.biocel.2016.09.011
4. Scicchitano P, Cortese F, Gesualdo M, De Palo M, Massari F, Giordano P, et al. The role of endothelial dysfunction and oxidative stress in cerebrovascular diseases. Free Radic Res (2019) 53:579–95. doi: 10.1080/10715762.2019.1620939
5. Chen X, Guo C, Kong J. Oxidative stress in neurodegenerative diseases. Neural Regener Res (2012) 7:376–85. doi: 10.3969/j.issn.1673-5374.2012.05.009
6. Singh A, Kukreti R, Saso L, Kukreti S. Oxidative stress: A key modulator in neurodegenerative diseases. Molecules (2019) 24:1583. doi: 10.3390/molecules24081583
7. Cobley JN, Fiorello ML, Bailey DM. 13 reasons why the brain is susceptible to oxidative stress. Redox Biol (2018) 15:490–503. doi: 10.1016/j.redox.2018.01.008
8. Olmez I, Ozyurt H. Reactive oxygen species and ischemic cerebrovascular disease. Neurochem Int (2012) 60:208–12. doi: 10.1016/j.neuint.2011.11.009
9. Broughton BR, Reutens DC, Sobey CG. Apoptotic mechanisms after cerebral ischemia. Stroke (2009) 40:e331–9. doi: 10.1161/STROKEAHA.108.531632
10. Yang X, Yang S, Xu H, Liu D, Zhang Y, Wang G. Superoxide dismutase gene polymorphism is associated with ischemic stroke risk in the China dali region han population. Neurologist (2021) 26:27–31. doi: 10.1097/NRL.0000000000000301
11. Klein JA, Ackerman SL. Oxidative stress, cell cycle, and neurodegeneration. J Clin Invest (2003) 111:785–93. doi: 10.1172/JCI200318182
12. Touyz RM. Reactive oxygen species, vascular oxidative stress, and redox signaling in hypertension: What is the clinical significance? Hypertension (2004) 44:248–52. doi: 10.1161/01.HYP.0000138070.47616.9d
13. Virani SS, Alonso A, Aparicio HJ, Benjamin EJ, Bittencourt MS, Callaway CW, et al. Heart disease and stroke statistics–2021 update: A report from the American heart association. Circulation (2021) 143:e254–743. doi: 10.1161/CIR.0000000000000950
14. Barzegar M, Stokes KY, Chernyshev O, Kelley RE, Alexander JS. The role of the ACE2/MasR axis in ischemic stroke: New insights for therapy. Biomedicines (2021) 9:1667. doi: 10.3390/biomedicines9111667
15. National Center for Health Statistics, Center for Disease Control & Prevention. Health, united states, 2016, with chartbook on long-term trends in health. Hyattsville, MD: U.S. Government Printing Office (2017). p. 570.
16. Zhu Y, Zhang H, Zhang Y, Wu H, Wei L, Zhou G, et al. Endovascular metal devices for the treatment of cerebrovascular diseases. Adv Mater (2019) 31:e1805452. doi: 10.1002/adma.201805452
17. Global Burden of Disease 2019 Stroke Collaborators. Global, regional, and national burden of stroke and its risk factors, 1990–2019: A systematic analysis for the global burden of disease study 2019. Lancet Neurol (2021) 20:795–820.
18. NCfHS Centers for Disease Control and Prevention. National vital statistics system, mortality 1999–2020 (2020). CDC WONDER Online Database. Available at: https://wonder.cdc.gov/mcd.html (Accessed March 20, 2021).
19. Chang WW, Fei SZ, Pan N, Yao YS, Jin YL. Incident stroke and its influencing factors in patients with type 2 diabetes mellitus and/or hypertension: A prospective cohort study. Front Cardiovasc Med (2022) 9:770025. doi: 10.3389/fcvm.2022.770025
20. Zorov DB, Juhaszova M, Sollott SJ. Mitochondrial reactive oxygen species (ROS) and ROS-induced ROS release. Physiol Rev (2014) 94:909–50. doi: 10.1152/physrev.00026.2013
21. Lubos E, Loscalzo J, Handy DE. Glutathione peroxidase-1 in health and disease: from molecular mechanisms to therapeutic opportunities. Antioxid Redox Signal (2011) 15:1957–97. doi: 10.1089/ars.2010.3586
22. Birben E, Sahiner UM, Sackesen C, Erzurum S, Kalayci O. Oxidative stress and antioxidant defense. World Allergy Organ J (2012) 5:9–19. doi: 10.1097/WOX.0b013e3182439613
23. Allen CL, Bayraktutan U. Oxidative stress and its role in the pathogenesis of ischaemic stroke. Int J Stroke (2009) 4:461–70. doi: 10.1111/j.1747-4949.2009.00387.x
24. Seet RC, Lee CY, Chan BP, Sharma VK, Teoh HL, Venketasubramanian N, et al. Oxidative damage in ischemic stroke revealed using multiple biomarkers. Stroke (2011) 42:2326–9. doi: 10.1161/STROKEAHA.111.618835
25. Warner DS, Sheng H, Batinic-Haberle I. Oxidants, antioxidants and the ischemic brain. J Exp Biol (2004) 207:3221–31. doi: 10.1242/jeb.01022
26. Cenini G, Lloret A, Cascella R. Oxidative stress in neurodegenerative diseases: From a mitochondrial point of view. Oxid Med Cell Longev (2019) 2019:2105607. doi: 10.1155/2019/2105607
27. Langen UH, Ayloo S, Gu C. Development and cell biology of the blood-brain barrier. Annu Rev Cell Dev Biol (2019) 35:591–613. doi: 10.1146/annurev-cellbio-100617-062608
28. Orellana-Urzua S, Rojas I, Libano L, Rodrigo R. Pathophysiology of ischemic stroke: role of oxidative stress. Curr Pharm Des (2020) 26:4246–60. doi: 10.2174/1381612826666200708133912
29. Abramov AY, Scorziello A, Duchen MR. Three distinct mechanisms generate oxygen free radicals in neurons and contribute to cell death during anoxia and reoxygenation. J Neurosci (2007) 27:1129–38. doi: 10.1523/JNEUROSCI.4468-06.2007
30. Sidorova Y, Domanskyi A. Detecting oxidative stress biomarkers in neurodegenerative disease models and patients. Methods Protoc (2020) 3:66. doi: 10.3390/mps3040066
31. Elsayed WM, Abdel-Gawad E-HA, Mesallam DI, El-Serafy TS. The relationship between oxidative stress and acute ischemic stroke severity and functional outcome. Egyptian J Neurol Psychiatr Neurosurg (2020) 56:1–6. doi: 10.1186/s41983-020-00206-y
32. Lorente L, Martin MM, Perez-Cejas A, Abreu-Gonzalez P, Ramos L, Argueso M, et al. Association between total antioxidant capacity and mortality in ischemic stroke patients. Ann Intensive Care (2016) 6:39. doi: 10.1186/s13613-016-0143-7
33. Yaseen Z, Chowdhury D, Shantaram M, Agarwal S, Sheela K. Prognostic significance of plasma homocysteine and malondialdehyde in patients with acute ischemic stroke. Int J Pharma Res Health Sci (2015) 3:727–36.
34. Del Rio D, Stewart AJ, Pellegrini N. A review of recent studies on malondialdehyde as toxic molecule and biological marker of oxidative stress. Nutr Metab Cardiovasc Dis (2005) 15:316–28. doi: 10.1016/j.numecd.2005.05.003
35. Webb AJS, Werring DJ. New insights into cerebrovascular pathophysiology and hypertension. Stroke (2022) 53:1054–64. doi: 10.1161/STROKEAHA.121.035850
36. Garry PS, Ezra M, Rowland MJ, Westbrook J, Pattinson KT. The role of the nitric oxide pathway in brain injury and its treatment–from bench to bedside. Exp Neurol (2015) 263:235–43. doi: 10.1016/j.expneurol.2014.10.017
37. Zhang L, Wu J, Duan X, Tian X, Shen H, Sun Q, et al. NADPH oxidase: A potential target for treatment of stroke. Oxid Med Cell Longev (2016) 2016:5026984. doi: 10.1155/2016/5026984
38. Zhou L, Xiang W, Potts J, Floyd M, Sharan C, Yang H, et al. Reduction in extracellular superoxide dismutase activity in African-American patients with hypertension. Free Radic Biol Med (2006) 41:1384–91. doi: 10.1016/j.freeradbiomed.2006.07.019
39. Faraci FM, Didion SP. Vascular protection: Superoxide dismutase isoforms in the vessel wall. Arterioscler Thromb Vasc Biol (2004) 24:1367–73. doi: 10.1161/01.ATV.0000133604.20182.cf
40. Otaki Y, Watanabe T, Nishiyama S, Takahashi H, Arimoto T, Shishido T, et al. The impact of superoxide dismutase-1 genetic variation on cardiovascular and all-cause mortality in a prospective cohort study: The yamagata (Takahata) study. PLoS One (2016) 11:e0164732. doi: 10.1371/journal.pone.0164732
41. Iadecola C, Davisson RL. Hypertension and cerebrovascular dysfunction. Cell Metab (2008) 7:476–84. doi: 10.1016/j.cmet.2008.03.010
42. Darroudi S, Tajbakhsh A, Esmaily H, Ghazizadeh H, Zamani P, Sadabadi F, et al. 50 bp deletion in promoter superoxide dismutase 1 gene and increasing risk of cardiovascular disease in mashhad stroke and heart atherosclerotic disorder cohort study. Biofactors (2020) 46:55–63. doi: 10.1002/biof.1575
43. Wong CH, Bozinovski S, Hertzog PJ, Hickey MJ, Crack PJ. Absence of glutathione peroxidase-1 exacerbates cerebral ischemia-reperfusion injury by reducing post-ischemic microvascular perfusion. J Neurochem (2008) 107:241–52. doi: 10.1111/j.1471-4159.2008.05605.x
44. Gorg B, Qvartskhava N, Bidmon HJ, Palomero-Gallagher N, Kircheis G, Zilles K, et al. Oxidative stress markers in the brain of patients with cirrhosis and hepatic encephalopathy. Hepatology (2010) 52:256–65. doi: 10.1002/hep.23656
45. Scammahorn JJ, Nguyen ITN, Bos EM, Van Goor H, Joles JA. Fighting oxidative stress with sulfur: Hydrogen sulfide in the renal and cardiovascular systems. Antioxidants (Basel) (2021) 10:373. doi: 10.3390/antiox10030373
46. Sun HJ, Wu ZY, Nie XW, Bian JS. Role of endothelial dysfunction in cardiovascular diseases: The link between inflammation and hydrogen sulfide. Front Pharmacol (2019) 10:1568. doi: 10.3389/fphar.2019.01568
47. Mani S, Untereiner A, Wu L, Wang R. Hydrogen sulfide and the pathogenesis of atherosclerosis. Antioxid Redox Signal (2014) 20:805–17. doi: 10.1089/ars.2013.5324
48. Whelton PK, Carey RM, Aronow WS, Casey DE, Collins KJ, Dennison Himmelfarb C, et al. 2017 ACC/AHA/AAPA/ABC/ACPM/AGS/APhA/ASH/ASPC/NMA/PCNA guideline for the prevention, detection, evaluation, and management of high blood pressure in adults: A report of the American college of Cardiology/American heart association task force on clinical practice guidelines. J Am Coll Cardiol (2018) 71:e127–248. doi: 10.1016/j.jacc.2017.11.006
49. Lammie GA. Hypertensive cerebral small vessel disease and stroke. Brain Pathol (2002) 12:358–70. doi: 10.1111/j.1750-3639.2002.tb00450.x
50. Zhou H, Zhang X, Lu J. Progress on diabetic cerebrovascular diseases. Bosn J Basic Med Sci (2014) 14:185–90. doi: 10.17305/bjbms.2014.4.203
51. Yahagi K, Kolodgie FD, Lutter C, Mori H, Romero ME, Finn AV, et al. Pathology of human coronary and carotid artery atherosclerosis and vascular calcification in diabetes mellitus. Arterioscler Thromb Vasc Biol (2017) 37:191–204. doi: 10.1161/ATVBAHA.116.306256
52. Han S, Liang CP, DeVries-Seimon T, Ranalletta M, Welch CL, Collins-Fletcher K, et al. Macrophage insulin receptor deficiency increases ER stress-induced apoptosis and necrotic core formation in advanced atherosclerotic lesions. Cell Metab (2006) 3:257–66. doi: 10.1016/j.cmet.2006.02.008
53. Li S, Sun Y, Liang CP, Thorp EB, Han S, Jehle AW, et al. Defective phagocytosis of apoptotic cells by macrophages in atherosclerotic lesions of ob/ob mice and reversal by a fish oil diet. Circ Res (2009) 105:1072–82. doi: 10.1161/CIRCRESAHA.109.199570
54. Eckel RH, Bornfeldt KE, Goldberg IJ. Cardiovascular disease in diabetes, beyond glucose. Cell Metab (2021) 33:1519–45. doi: 10.1016/j.cmet.2021.07.001
55. Mendis S, Graham I, Narula J. Addressing the global burden of cardiovascular diseases; need for scalable and sustainable frameworks. Glob Heart (2022) 17:48. doi: 10.5334/gh.1139
56. Arboix A. Cardiovascular risk factors for acute stroke: Risk profiles in the different subtypes of ischemic stroke. World J Clin cases (2015) 3:418–29. doi: 10.12998/wjcc.v3.i5.418
57. Virani SS, Alonso A, Benjamin EJ, Bittencourt MS, Callaway CW, Carson AP, et al. American Heart association council on epidemiology and prevention statistics committee and stroke statistics subcommittee. heart disease and stroke statistics–2020 update: A report from the American heart association. Circulation (2020) 141:e139–596. doi: 10.1161/CIR.0000000000000757
58. French CR, Seshadri S, Destefano AL, Fornage M, Arnold CR, Gage PJ, et al. Mutation of FOXC1 and PITX2 induces cerebral small-vessel disease. J Clin Invest (2014) 124:4877–81. doi: 10.1172/JCI75109
59. Malik R, Traylor M, Pulit SL, Bevan S, Hopewell JC, Holliday EG, et al. METASTROKE collaboration, wellcome trust case control consortium 2, NINDS stroke genetics network. low-frequency and common genetic variation in ischemic stroke: The METASTROKE collaboration. Neurology (2016) 86:1217–26. doi: 10.1212/WNL.0000000000002528
60. Chauhan G, Debette S. Genetic risk factors for ischemic and hemorrhagic stroke. Curr Cardiol Rep (2016) 18:124. doi: 10.1007/s11886-016-0804-z
61. Boehme AK, Esenwa C, Elkind MS. Stroke risk factors, genetics, and prevention. Circ Res (2017) 120:472–95. doi: 10.1161/CIRCRESAHA.116.308398
62. Khatri R, Vellipuram AR, Maud A, Cruz-Flores S, Rodriguez GJ. Current endovascular approach to the management of acute ischemic stroke. Curr Cardiol Rep (2018) 20:46. doi: 10.1007/s11886-018-0989-4
63. Chugh C. Acute ischemic stroke: Management approach. Indian J Crit Care Med (2019) 23:S140–6. doi: 10.5005/jp-journals-10071-23192
64. Rosskopf J, Kloth C, Dreyhaupt J, Braun M, Schmitz BL, Graeter T. Thin slices and maximum intensity projection reconstructions increase sensitivity to hyperdense middle cerebral artery sign in acute ischemic stroke. Cerebrovasc Dis (2020) 49:437–41. doi: 10.1159/000509378
65. Radhiana H, Syazarina SO, Shahizon Azura MM, Hilwati H, Sobri MA. Non-contrast computed tomography in acute ischaemic stroke: A pictorial review. Med J Malaysia (2013) 68:93–100.
66. El-Wahsh S, Dunkerton S, Ang T, Winters HS, Delcourt C. Current perspectives on neuroimaging techniques used to identify stroke mimics in clinical practice. Expert Rev Neurother (2021) 21:517–31. doi: 10.1080/14737175.2021.1911650
67. de Havenon A, Meyer C, McNally JS, Alexander M, Chung L. Subclinical cerebrovascular disease: Epidemiology and treatment. Curr Atheroscler Rep (2019) 21:39. doi: 10.1007/s11883-019-0799-1
68. Liu C, Du L, Wang S, Kong L, Zhang S, Li S, et al. Differences in the prevention and control of cardiovascular and cerebrovascular diseases. Pharmacol Res (2021) 170:105737. doi: 10.1016/j.phrs.2021.105737
69. Zheng SL, Roddick AJ. Association of aspirin use for primary prevention with cardiovascular events and bleeding events: A systematic review and meta-analysis. JAMA (2019) 321:277–87. doi: 10.1001/jama.2018.20578
70. Margaill I, Plotkine M, Lerouet D. Antioxidant strategies in the treatment of stroke. Free Radic Biol Med (2005) 39:429–43. doi: 10.1016/j.freeradbiomed.2005.05.003
71. Gaire BP. Herbal medicine in ischemic stroke: Challenges and prospective. Chin J Integr Med (2018) 24:243–6. doi: 10.1007/s11655-018-2828-2
72. Zimmerman C, Yarnell E. Herbal medicine for stroke. Altern Complement Ther (2018) 24:232–9. doi: 10.1089/act.2018.29186.eya
73. Zhu T, Wang L, Feng Y, Sun G, Sun X. Classical active ingredients and extracts of Chinese herbal medicines: Pharmacokinetics, pharmacodynamics, and molecular mechanisms for ischemic stroke. Oxid Med Cell Longev (2021) 2021:8868941. doi: 10.1155/2021/8868941
74. Tao T, Liu M, Chen M, Luo Y, Wang C, Xu T, et al. Natural medicine in neuroprotection for ischemic stroke: Challenges and prospective. Pharmacol Ther (2020) 216:107695. doi: 10.1016/j.pharmthera.2020.107695
Keywords: oxidative stress, neurodegeneration, stroke, risk factors, hypertension
Citation: Kumar V, Bishayee K, Park S, Lee U and Kim J (2023) Oxidative stress in cerebrovascular disease and associated diseases. Front. Endocrinol. 14:1124419. doi: 10.3389/fendo.2023.1124419
Received: 15 December 2022; Accepted: 02 February 2023;
Published: 17 February 2023.
Edited by:
Aleksandra Klisic, Primary Health Care Center Podgorica, MontenegroReviewed by:
Esma R. Isenovic, University of Belgrade, SerbiaCopyright © 2023 Kumar, Bishayee, Park, Lee and Kim. This is an open-access article distributed under the terms of the Creative Commons Attribution License (CC BY). The use, distribution or reproduction in other forums is permitted, provided the original author(s) and the copyright owner(s) are credited and that the original publication in this journal is cited, in accordance with accepted academic practice. No use, distribution or reproduction is permitted which does not comply with these terms.
*Correspondence: Jaebong Kim, jbkim@hallym.ac.kr