Macrophage-extracellular matrix interactions: Perspectives for tissue engineered heart valve remodeling
- 1Institute for Regenerative Medicine, University of Zurich, Schlieren, Switzerland
- 2Wyss Zurich, University and Swiss Federal Institute of Technology (ETH) Zurich, Zurich, Switzerland
- 3Department of Cardiovascular Surgery, Charité Universitätsmedizin Berlin, Berlin, Germany
- 4Department of Cardiothoracic and Vascular Surgery, German Heart Center Berlin, Berlin, Germany
In situ heart valve tissue engineering approaches have been proposed as promising strategies to overcome the limitations of current heart valve replacements. Tissue engineered heart valves (TEHVs) generated from in vitro grown tissue engineered matrices (TEMs) aim at mimicking the microenvironmental cues from the extracellular matrix (ECM) to favor integration and remodeling of the implant. A key role of the ECM is to provide mechanical support to and attract host cells into the construct. Additionally, each ECM component plays a critical role in regulating cell adhesion, growth, migration, and differentiation potential. Importantly, the immune response to the implanted TEHV is also modulated biophysically via macrophage-ECM protein interactions. Therefore, the aim of this review is to summarize what is currently known about the interactions and signaling networks occurring between ECM proteins and macrophages, and how these interactions may impact the long-term in situ remodeling outcomes of TEMs. First, we provide an overview of in situ tissue engineering approaches and their clinical relevance, followed by a discussion on the fundamentals of the remodeling cascades. We then focus on the role of circulation-derived and resident tissue macrophages, with particular emphasis on the ramifications that ECM proteins and peptides may have in regulating the host immune response. Finally, the relevance of these findings for heart valve tissue engineering applications is discussed.
Introduction
Every tissue in the body has distinct extracellular matrix (ECM) composition, that arises from a unique combination of up to ∼300 different ECM (e.g., collagen subunits, proteoglycans and glycoproteins) and ECM-related protein components (e.g., secreted factors and ECM regulators) (1). The main role of the ECM is to provide structural support to each tissue type and organ. In addition, each ECM component also plays a direct role in controlling cell adhesion, regulating cell growth, migration, proliferation, and differentiation potential (2); the native heart valve ECM being no exception.
Due to the distinct three-layer ECM composition, the heart valve leaflet structure is able to withstand constant pressure changes during the cardiac cycle (3, 4). The ventricularis or atrial is side of the leaflets, those which are exposed to pulsatile shear stress, are composed of radially oriented elastin fibers and provide the elastic recoil needed for when the valve opens and closes (5). The middle layer, or spongiosa of the valve, is comprised of proteoglycans such as chondroitin sulfate, glycosaminoglycans, and sparsely packed collagen fibers, all of which mitigate compression forces when the valve is closed (6). The circumferentially aligned, densely packed, collagen-rich (mainly collagen 1 (COL1), but also COL3) fibrosa layer is situated at the outflow tract and is the main structure that provides strength and stiffness necessary for valve sufficiency (7).
Because the ECM is the core component of heart valve functionality, diseases that abrogate ECM function, such as genetic conditions (8–11) and calcific aortic valve disease (CAVD) (12, 13), can disrupt normal valve performance and often warrants valve replacement. CAVD is the most common valve disease and is a progressive degeneration ranging from non-obstructive valve thickening to severe valve calcification, which may result in impaired leaflet movement and eventually leading to valve stenosis (12, 13). CAVD development is an active inflammatory process that culminate with the release of matrix metalloproteinases (MMPs) and cathepsins that drive pathogenic ECM remodeling and calcium deposition (14, 15). This may result in severe valve insufficiency and/or stenosis that will ultimately necessitate replacement.
In most cases, treating these severe conditions requires a valve replacement procedure (16), either using mechanical or bioprosthetic valves, as extensively reviewed elsewhere (17, 18). Despite the remarkable heart valve prosthesis evolution (19), in particular with the advent of transcatheter techniques (20), several clinical and societal dilemmas remain (Box 1). Importantly, current clinical-grade heart valve replacement solutions lack the ability to remodel, repair and/or grow upon implantation, determining a high incidence of reoperation to replace the valve implant, in particular in the young cohort (<60 years old) (21).
BOX 1 Remaining clinical and societal implications for heart valve therapies. |
• Heart valve replacement procedures of severely dysfunctional valves, either using mechanical or bioprosthetic valves, is expected to reach 850,000 implants annually by 2050 (22). |
• Patients receiving a mechanical valve are subjected to life-long anticoagulant treatment to prevent thrombosis (23). |
• For elderly patients, current guidelines recommend a bioprosthetic valve generated from glutaraldehyde-fixed xenogenic tissue (e.g., porcine valves or bovine pericardium). This prosthesis has an improved hemodynamic profile, reducing the need for anti-coagulation therapy (24). |
• Bioprostheses have residual immunogenicity (i.e., xenogenic alpha-gal epitopes) that can cause chronic inflammation upon implantation (25, 26). This leads to degenerative failure, and limited durability of the prosthesis, causing the need for multiple re-interventions in the young (21, 24, 27). |
• European health care costs for patients having a heart valve replacement exceed €1 billion annually (28). |
In situ heart valve tissue engineering has been proposed as a promising solution to achieve “next-generation” heart valve prostheses with the potential for life-long durability (18). Remarkably, tissue engineered heart valves (TEHVs), manufactured using either decellularized allogenic/xenogeneic valves or bioresorbable polymer-based valves, have reached clinical translation (29–35). While wound healing and the foreign body response are amongst the most hypothesized mechanisms behind the integration and remodeling of TEHVs (36–38), less attention has been given to the role of ECM proteins in regulating remodeling upon implantation. Therefore, the aim of this review is to describe what is currently known about the interactions and signaling networks occurring between ECM proteins and macrophages, and how these interactions may impact the long-term in situ remodeling outcomes of tissue engineered ECM (TEM)-based implants. First, we provide an overview of in situ heart valve tissue engineering approaches with a particular focus on TEM-based TEHVs (section “In situ heart valve tissue engineering”). Then, we briefly summarize the fundamentals of the wound healing and remodeling cascades, specifically examining two of the first responders to injury, circulation-derived macrophages and resident tissue macrophages (RTMs) (section “The role of macrophages in tissue remodeling”). We then discuss the role of ECM proteins, such as collagens (COL), fibronectin (FN), and their corresponding peptides, in regulating the macrophage response, with particular emphasis on the implications for remodeling mechanisms (section “Immunoregulation of extracellular matrix proteins”). Finally, the relevance of these findings for heart valve tissue engineering applications is discussed by proposing examples of scaffold functionalization using ECM or ECM-related proteins to regulate the host immune response toward adaptive remodeling (section “Discussion: Relevance for in situ heart valve tissue engineering”).
In situ heart valve tissue engineering
In situ tissue engineering relies on the regenerative potential of the recipient’s body to integrate and remodel an implanted off-the-shelf available construct. The scaffold used for this approach should withstand the native mechanical environment immediately upon implantation, favor host cell adhesion, migration and proliferation, support ECM production, and allow for adaptive remodeling toward a native-like functional living tissue (18, 36).
To achieve this, a multitude of different tissue engineering strategies have investigated distinct scaffold materials that ensure functionality and remodeling of the implanted TEHV, as extensively reviewed elsewhere (18). Briefly, TEHVs utilizing decellularized homografts have shown favorable long-term performance and limited in situ cell repopulation in clinical trials (30, 39–42). However, maladaptive remodeling phenomena (i.e., fibrosis and calcification) and not favorable long-term outcomes (i.e., 51% of freedom from reoperation at 10 years compared to 80% for standard cryopreserved allografts) have been reported (43–45).
TEHVs manufactured from decellularized xenografts have been associated with controversial results, with marked discrepancy between preclinical and clinical studies. Briefly, preclinical investigations in large animal models showed promising performance of xenograft-based TEHVs, with cellular infiltration throughout the tissue thickness (46). However, clinical trials were mostly unsuccessful, with signs of maladaptive remodeling [i.e., fibrosis, leaflet thickening, calcification, lack of cellularization, and chronic inflammation (47–53)], that may have caused the observed valve insufficiency and/or stenosis.
The functionality and remodeling potential of bioresorbable polymeric valves, which are compatible with surgical and transcatheter implantation techniques, were first demonstrated in large animal models (54–59). Within these studies, the valves showed acceptable functionality for up to 12 months, rapid cellularization, ECM deposition and progressive scaffold reabsorption (56, 58). Clinical translation of this approach is currently ongoing. A first pulmonary valve conduit design was evaluated in 12 pediatric patients [Xplore-1 study (34)], but moderate-to-severe pulmonary valve regurgitation was observed in 11 out of 12 patients (60). A retrospective analysis of the implanted conduits revealed leaflet thickness heterogenicity, with the leaflets being thinner in the commissural area. Therefore, the leaflet design was modified to achieve a more homogeneous thickness distribution. The improved of pulmonary valve conduit design has been then implanted in six children [Xplore-2 study (35)]. In this trial, moderate pulmonary valve regurgitation was reported for only 1 patient at the 12 months follow-up (60). In addition, 1 patient developed rapidly progressing stenosis and required conduit replacement (60). Given the mixed outcomes of these two clinical trials, as well as the reported intra-valve and inter-valve differences in remodeling and incomplete scaffold reabsorption after 1 year in preclinical studies (56, 58, 61–63), further data with longer follow-up time points are needed to determine the true clinical value of this approach. Alternatively, in vitro grown human cell-derived tissue engineered matrices (TEMs) have been recently proposed as a promising material to fulfill the need for an easily accessible and an off-the-shelf option for TEHV development (64, 65).
TEMs are obtained by in vitro culture of human fibroblast-like cells [e.g., human dermal fibroblasts or vascular-derived myofibroblasts (64–68)] on a biodegradable scaffold [i.e., fibrin gel or poly-4-hydroxybutirate (P4HB) coated polyglycolic acid (PGA) (64, 67–70)] for a pre-determined amount of time to have sufficient deposition of ECM proteins. After culture, the tissue is decellularized to ensure immunocompatibility and grant off-the-shelf availability, while also preserving the ECM structure (66). The resulting TEM can then be used to manufacture off-the-shelf available TEHVs to replace (pulmonary or aortic) heart valves with promising acute performance (64, 65), and sustained functionality, up to 1 year (67), with host cell repopulation, endothelialization, integration, and remodeling potential over time being consistently observed (67, 68, 70–74).
TEMs can be described as complex materials, comprised of a multitude of ECM proteins, minimal amounts of residual DNA that may remain from the decellularization process, and possibly bioresorbable polymer remnants (64, 65, 67, 68, 70, 71, 74). Upon implantation, each of these TEM components have a potential impact on the immune response to the implant and, thus, will contribute to its remodeling process (75); the ECM proteins being no exception. Generally, the ECM components of a TEM implant are considered for their mechanical properties (64, 71). Importantly, ECM proteins also have significant biocompatible properties that can guide cell behavior (76), and can potentially regulate macrophage polarization upon implantation.
The role of macrophages in tissue remodeling
The remodeling of a tissue engineered construct is a highly complex and dynamic process characterized by the interaction of different immune cells with the implant, followed by the release of cytokines to further attract immune and specialized cells to the injury/implantation site (75). Little is known about the remodeling following an injury in native heart valve leaflets. Animal studies indicate surgically wounded valves remodel by recruiting activated macrophages, myofibroblasts, and endothelial cells that contribute to the deposition of proteoglycans and secretion of MMPs (77–79). Eventually, as a result of this healing cascade, a collagen-rich fibrous tissue is formed and, over time, the valve leaflet is re-endothelialized (79). Macrophages have been repeatedly reported as early responders that govern wound healing and remodeling processes by secreting growth factors, chemokines and cytokines [e.g., transforming growth factor beta (TGFβ), fibroblast growth factor (FGF), platelet derived growth factor (PDGF) and vascular endothelial growth factor (VEGF) (80)] as extensively reviewed elsewhere (81, 82). This occurs also in the heart valves, where by secreting TGFβ and FGF, macrophages play a key role in activating valvular interstitial cells (VICs), to favor proliferation and migration during valve repair and remodeling (83). However, aberrant TGFβ stimulation is also the first step to pathogenic fibrosis and/or osteogenesis, potentially leading to fibrotic valve stenosis and calcific valve disease (84). Therefore, there is a fine balance between the signaling molecules secreted by macrophages that are needed to decide the fate between fibrosis and regeneration of both native and TEHVs, making these cells one of the key players in the repair and remodeling cascades.
Importantly, it is very likely that the host response to an implanted engineered valve will substantially differ from the innate remodeling following an injury, in particular if an implant leads to the chronic activation of immune cells and foreign body response. After 2–4 weeks from implantation, the surface properties of the implant will modulate the foreign body reaction, by affecting protein adsorption and early responding immune cells adhesion, thereby affecting the inflammatory cascade and the subsequent cellular recruitment and activation on the implant (85). Although it is currently difficult to predict the human immune response to implanted material, we present an overview of circulation-derived and resident tissue macrophages’ role in host immune response in the following sections. Traditionally, all macrophages were considered to be derived from the bone marrow through circulating monocytes (section “Circulation-derived macrophages”) (Figure 1A). More recently, it has been established that resident tissue macrophages (section “Resident tissue macrophages”) are derived from embryonic progenitors and can persist and proliferate throughout adulthood (86) (Figure 1B).
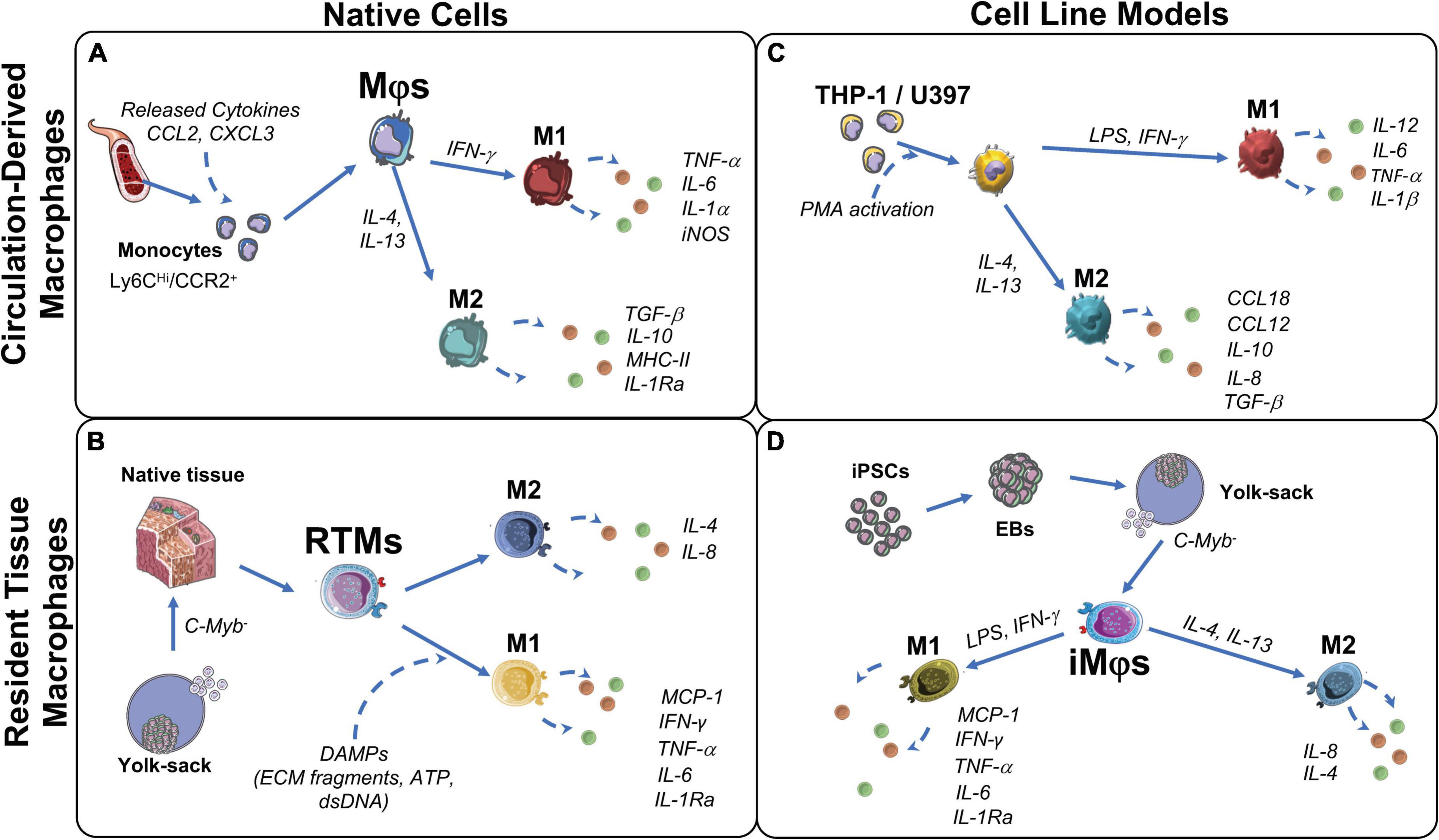
Figure 1. Origin and function of macrophages and their corresponding cell models. (A) Circulation-derived monocytes (CD14Hi/CCR2+), activated by C-C motif chemokine ligand 2 (CCL2) and C-X-C chemokine ligand 3 (CXCL3) from cells at the wound site, differentiate to macrophages (Mφs). When in contact with cell debris and ECM fragments, Mφs polarize to M1 to induce clearance of the damaged cells/proteins. M1- Mφs secrete pro-inflammatory factors to attract ECM producing stromal cells and restore tissue integrity. Over time, to resolve inflammation, Mφs polarize to M2. M2-Mφs secrete anti-inflammatory factors that favor remodeling and restore tissue integrity. (B) Resident tissue macrophages (RTMs) are derived from C-Myb– hematopoiesis in the yolk-sac and reside in native fetal tissues, but persists into adulthood. Upon tissue damage, RTMs are activated by damage associated molecular patterns (DAMPs) and polarize to M1. M1-RTMs secret pro-inflammatory cytokines that recruit circulating monocytes and neutrophils, but then quickly polarize to the M2 state to promote tissue restoration and homeostasis. (C) Immortalized leukemic cells THP-1 and U937 are used to model peripheral blood-derived monocytes and Mφs. M1 polarization is induced by lipopolysaccharides (LPS), interferon (IFN)-γ with subsequent pro-inflammatory cytokine production interleukin (IL)-12, IL-6, IL-1β, and tissue necrosis factor (TNF)-α. M2 polarization is induced by IL-4 and IL-13 with subsequent production of IL-10, IL-8, CCL18, CCL12 and transforming growth factor (TGF)β. (D) iMφs are a proposed model of RTMs in vitro. Their C-Myb independent ontogeny is similar to RTMs and have shown to polarize in a similar manner to the M1 (LPS, IFN-γ) and M2 (IL-4, IL-13) states. EB, embryoid body; iNOS, inducible nitric oxide synthase; iPSCs, induced pluripotent stem cells; LPS, lipopolysaccharides; MCP-1, monocyte chemoattractant protein 1; MHC-II, major histocompatibility complex class II; PMA, protein kinase C activator.
Circulation-derived macrophages
Circulation-derived macrophages originate from monocytes (carrying markers CD14Hi, CCR2+ and CSFR2+) that circulate in a dormant steady state and are products of hematopoiesis (87, 88). Circulating monocytes are attracted to the surface of a wounded area and/or implant, where they differentiate to macrophages (87, 88) (Figure 1A). These macrophages remove cell debris as well as clear foreign materials (82). In addition, they enable collagen deposition in the injured area, or on the implanted biomaterial, in order to induce tissue restoration and support further immune cell and fibroblast recruitment via cytokine release (88, 89).
The cytokines secreted in the early stages of wound healing are highly dependent on the biochemical cues present in the wound or on the surface of the implanted material. Several determinants, such as the presence of cell debris, external pathogens, ECM proteins, and foreign materials (i.e., bioresorbable polymers), can impact whether the circulation-derived macrophages display a pro- or anti-inflammatory phenotype (90). Pro-inflammatory polarization, primarily comprised of M1 sub-populations, enables the formation of multinucleated foreign body giant cells (FBGCs) with the intent to engulf and clear any foreign material and remove cellular debris. Normally, when M1 macrophages have concluded the clearance of cell debris and foreign bodies, further M1 polarization is not required and the cells repolarize into an anti-inflammatory state, generally known as the M2 state. Anti-inflammatory polarization is comprised primarily of M2 (e.g., M2a, M2b, M2c, and M2d) macrophage subgroups, and promotes tissue regeneration by secreting anti-inflammatory cytokines such as interleukin 10 (IL-10), IL-4, IL-1Ra and growth factors like TGFβ1, thereby attracting fibroblasts to restore tissue integrity (91, 92). Both M1 and M2 are considered dynamic states and with inherent plasticity that depends on the stimulation they receive from their surrounding environment or the presence of paracrine factors (92), thereby enabling the spontaneous initiation and resolution of inflammation. Importantly, macrophages guide de novo ECM synthesis by recruiting ECM producing α-smooth muscle actin (αSMA)-positive cells, but also by directly depositing ECM proteins (89, 93). ECM production by macrophages is primarily influenced by polarization toward the M2 subgroups (92). In vitro studies have showcased the direct production of collagens (COLVI and COLVIII) from stimulated monocytes and macrophages (94), as well as glycosaminoglycans and α-elastin in a strain-dependent manner, identifying macrophages as direct regulator of ECM turnover and synthesis (95). In vivo studies focusing on the cardiac wound healing response demonstrated that macrophages can directly deposit collagen in scar formation upon cardiac injury (96), further highlighting the role of macrophage-ECM crosstalk that should to be taken into consideration for TEHV implantation. M2 macrophages also enable ECM production and turnover at the wound/implantation site indirectly. For example, IL-10 and TGFβ1 secretion recruits and activates fibroblasts leading to ECM deposition in a myocardial infarction model (97). Furthermore, the paracrine effects of TGFβ1, PDGF, and MMP secretion have been shown to activate ECM producing cells, such as fibroblasts, to favor tissue repair and remodeling (96). However, the regulation of direct collagen synthesis from M2 macrophages remains unclear.
Thus, adaptive or maladaptive remodeling of a tissue engineered implant is a complex process highly dependent on the inflammatory response and, more specifically, on macrophage polarization. In the case of TEM-based cardiovascular implants, a multitude of ECM proteins such collagens, glycoproteins and proteoglycans are in direct contact with the circulation-derived macrophages through a network of signaling proteins such as integrins (85). These interactions may impact the long-term remodeling outcomes of in situ regeneration, and will be further detailed in section “Immunoregulation of extracellular matrix proteins.”
Resident tissue macrophages
While circulation-derived macrophages originate from blood monocytes, resident tissue macrophages (RTMs) can originate from: (a) embryonic development, independent of hematopoiesis, and from (b) infiltrating monocytes that arise from bone marrow hematopoiesis in adulthood (98). Compared to circulation-derived macrophages, for RTMs from embryonic origin, primitive and transient hematopoiesis is c-Myb independent (99) (Figure 1B). In adulthood, RTMs reside in all tissues and are responsible for tissue homeostasis and immune surveillance with distinct functions specific for each tissue’s microenvironment (100). However, the exact origin of adult RTMs and the mechanisms of RTMs maintenance within the adult tissue are currently not clearly defined (101).
RTMs possess self-renewal capacity and, upon tissue injury, are rapidly activated by signals released from damaged cells (i.e., calcium, ATP, H2O2, and DNA) (100). As the first responders in the wound healing cascade due to their proximity to injured tissue, activation of RTMs enables immediate pro-inflammatory cytokine release (i.e., tissue necrosis factor (TNF)-α, inducible nitric oxide synthase (iNOS), and IL-1β), which then recruits neutrophils and monocytes (102). As soon as RTMs initiate the pro-inflammatory response, they start producing ECM in order to recover tissue integrity and provide supportive matrix for cell infiltration (102). Due to their constant interaction with ECM, RTMs play an integral role in tissue remodeling for in situ regeneration (88). In addition to circulation-derived macrophages, RTMs may also play a role in remodeling implanted materials. When a foreign material, like the hTEM, is implanted, RTMs may be capable of infiltrating into the implant from adjacent tissues, including the heart muscle and vascular wall. Whether RTMs promote or resolve rapid inflammation and signal adaptive tissue remodeling upon contact with TEM-based implants, still remains to be further explored in the future. In terms of TEHVs, cells are known to infiltrate the implant from the arterial wall (38). However, whether RTMs may undergo such migration has not been elucidated to this date.
The role of macrophages in cardiovascular tissue development, homeostasis, and disease, has been recently examined mostly murine models, as reviewed elsewhere (103). In regards to heart tissue, cardiac-resident macrophages play a key role in homeostasis as well as in limiting damage extension following a cardiac injury, resulting in necrotic and apoptotic cell clearance, promotion of angiogenesis and reduction of inflammation (98). In the heart valve, CD45+ cells identified in the leaflet were originally hypothesized to differentiate toward VICs (104). However, further analyses have identified an immune population with macrophage characteristics in these CD45+ cells (105, 106). In addition, adult and developing heart valves have revealed the presence of multiple immune cell populations with characteristic markers of RTMs (106–108). Similar to cardiac RTMs, valvular RTMs have a role in tissue homeostasis, and have been associated to ECM remodeling, aging, and disease (106–108). Harnessing the characterization of these cell types in native heart valves, as well as in TEHVs, would greatly improve our understanding of in situ regeneration and remodeling.
Although RTMs have been thoroughly investigated and characterized through lineage tracing experiments in mouse models, their detailed function has not been elucidated for every organ system in humans (109). In humans, the functional characterization of these cells has been limited primarily because RTMs are challenging to isolate, due to the minimal number available in the tissues (109). The use of macrophage cell models is therefore currently being proposed as a solution to combat these challenges (section “In vitro macrophage models”).
In vitro macrophage models
Macrophage behavior in vitro has been investigated through a variety of cell lines isolated from human donors. Peripheral blood mononuclear cells (PBMCs) are the most accessible source of macrophages and have been studied extensively to assess the early steps of the remodeling cascade, early ECM production as well as material immunocompatibility in the cardiovascular tissue engineering field (95, 110–115); their advantages and disadvantages have been extensively reviewed elsewhere (116). However, human monocytes and monocyte-derived macrophages lack in vitro proliferation potential, leading to the constant need for new donor material (87). This disadvantage coupled with the inter-donor variability prompted researchers to use immortalized cell lines from a similar origin, such as leukemic immortalized monocytes THP-1 and U937 cells (87, 117) (Figure 1C). Compared to PBMCs, the high proliferation rate of these cell lines make them a practical source to study monocyte and macrophage function and differentiation, manifested by their broad in vitro applications for immune modulation approaches and in the cardiovascular tissue engineering field (117–119). However, there have been highlighted differences between THP-1 and PBMCs. Some suggest to limit the use of THP-1 cells when studying polarization, as THP-1 cells have a bias toward phagocytosis and the M1 phenotype (116, 120). Moreover, the malignant genetic background of these immortalized cells puts their application relevance into question. Especially as in vivo studies would still be required to validate any in vitro experiments using immortalized cell lines.
Due to RTMs paucity in tissues and limited availability of human donor materials, isolation in large numbers has been an unconquered milestone. Recently, iPSC-derived macrophages (iMφs) produce c-Myb independent macrophage-like cells from yolk sac-like structures (Figure 1D) and have been proposed to accurately mimic RTM biology in vitro (121–124). So far, due to their plasticity and adaptation potential to environmental stimuli, tissue specific resident-like iMφs such as alveolar-like, brain tissue resident-like (microglia-like), skin resident Langerhans-like, and liver resident Kuppfer-like iMφs have been successfully produced (123, 125, 126). However, research is still ongoing into iMφs that are functionally similar to cardiovascular RTMs. The use of iMφs may prove to be valuable for in vitro modeling of RTMs in wound healing and remodeling processes, specifically for in situ regeneration approaches.
Immunoregulation of extracellular matrix proteins
The multi-step process of remodeling is not only controlled biochemically by cytokines, but also biophysically by cell-ECM interactions (76, 127). Hence, implants manufactured from decellularized ECM, such as TEMs, may actively influence immune cell (i.e., macrophages) behavior via integrin- or non-integrin mediated signaling.
Integrin-mediated cell-ECM interactions occur when transmembrane integrin receptors, such as focal adhesions, physically bind to different ECM components and lead to signal transduction mechanisms (2, 128, 129). These connections allow cells to perceive changes in the ECM microenvironment (e.g., composition, stiffness, and orientation), as well as to migrate through the ECM (130). TEM-based TEHVs have been reported to contain ECM proteins like collagens (COLI and COLIII), FN, as well as glycosaminoglycans (64, 65), therefore having a potential role in regulating macrophage response (64, 65), which should be further investigated.
Non-integrin mediated cell-ECM interactions are observed in the presence of bioactive ECM peptides or epitopes, also known as matrikines or matricryptins, generated by ECM fragmentation (2, 131). Both matrikines and matricryptins refer to ECM peptides that are usually not exposed in intact and mature ECM fibers, but that are able to regulate cellular activity when exposed after degeneration. These ECM sites become available only after structural or conformational alterations (i.e., enzymatic degradation, self-assembly, denaturation, cell-mediated mechanical forces, and adsorption to surfaces) to the ECM proteins (132). In the field of tissue repair and regeneration, it is possible to identify these ECM transformations at the site of tissue injury, suggesting that the consequent exposure of specific ECM peptides may provide new signaling cues to regulate immune cells and tissue restoration (132). It is to be expected that these protein and relative fragments are also similarly present at the site of implantation of a tissue engineered implant, as well as they may be contained in a TEM-based valve replacement. Importantly, these molecules form a class of damage-associated molecular patterns (DAMPs) that activate immune (e.g., macrophages) and differentiated (e.g., fibroblasts) cells via toll-like receptor (TLR2 and TLR4) signaling (133). This alerts the immune system to tissue damage and initiates tissue repair (2, 76, 134, 135). Among the ECM peptides discovered to be capable of acting as DAMPs, there are those derived by FN, fibrinogen, versican, and heparan sulfate. Overall, these peptides engage with multiple pattern recognition receptors and initiate a pro-inflammatory response (136). As an example, biglycan and decorin have been reported to be capable of both generating a pro-inflammatory response in macrophages by interacting with TLR2 and TLR4, as well as promoting an anti-inflammatory response critical for the resolution of inflammation (137–139). In addition, bioactive ECM fragments from the basement membrane have been identified in both healthy and diseased hearts, and associated to cardiac disease (140). An example is endostatin, one of the most investigated peptides derived from enzymatical cleavage of COLXVIII. Endostatin is mostly known for its anti-angiogenic properties, but also anti-fibrotic and anti-tumor effects, via the reduction of anti-inflammatory cytokine (e.g., IL-4, IL-10, IL-13, and VEGF) expression and of M2 polarization (141).
In the following sections, we will discuss what is known about the effect that ECM proteins (such as collagen and FN, that have been found in TEM-based TEHVs) and ECM-derived peptides have on macrophages and their relevance for repair and remodeling mechanisms (Figure 2).
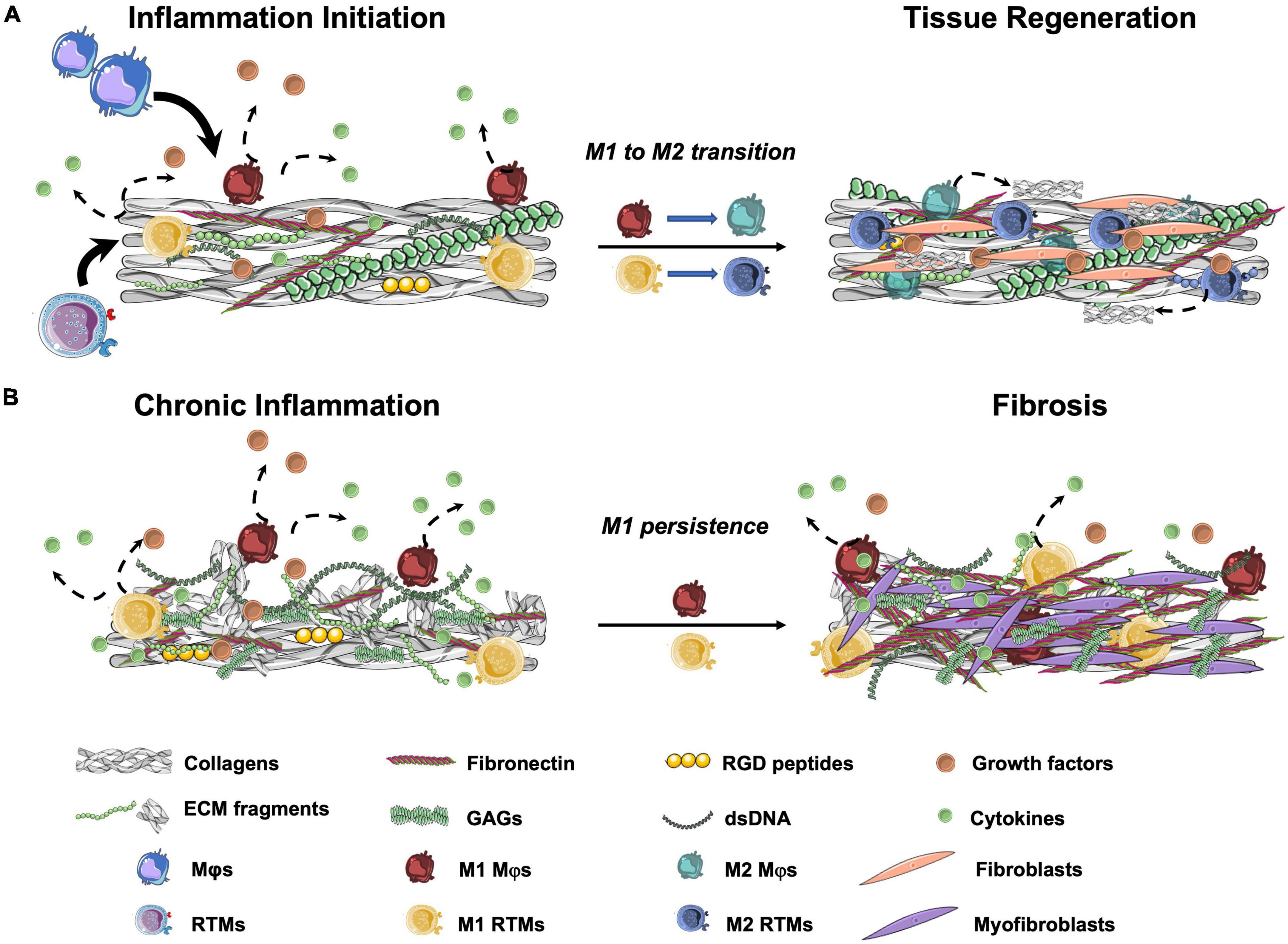
Figure 2. Schematic representation of wound healing outcomes after tissue injury. (A) In normal wound healing cascades, resident tissue macrophages (RTMs) and circulation-derived macrophages (Mφs) are attracted to the damaged area. When in contact with cell debris and ECM fragments, RTMs and Mφs polarize to the M1 state and produce pro-inflammatory cytokines. After the initial pro-inflammatory response, M2 polarization with progressive resolution of the inflammation occurs. Among other stromal cells, fibroblasts are recruited to site to restore tissue integrity. (B) In the presence of a high amounts of ECM fragments, peptides, cell debris and/or DNA remnants, prolonged M1 polarization with limited or absent M2 transition occurs. This results in a chronic pro-inflammatory stimulation that leads to the production of unorganized collagen-rich ECM by the activated fibroblasts (myofibroblasts) and may cause tissue fibrosis. GAGs, glycosaminoglycans; RGD, Arg-Gly-Asp.
Macrophage/collagen interactions
Collagens are the most abundant proteins in the human body (142) and one of the major components of in vitro grown TEMs. Collagens provide structural and mechanical properties to the tissue, while also playing an important role in controlling and regulating immune cells (143). In this regard, macrophages have been shown to be key players in controlling collagen homeostasis by contributing to collagen degradation and turnover (144), but also collagen synthesis in vitro and in vivo (e.g., tissue fibrosis during heart repair) (96, 144).
The immunomodulatory effect of collagen is a consequence of the different ligands that can be recognized by immune cells [e.g., integrins, discoidin domain receptors, immunoglobulin-like receptors, and mannose receptors (88, 96)]. These interactions have been reported to increase cell adhesion and integrin expression in both innate and adaptive immune cells (145), and reduce the inflammatory response of macrophages via the leukocyte-associated immunoglobulin-like receptor 1 (LAIR-1 or CD305) (146, 147). In addition, macrophage migration and mechanosensing in a fibrillar collagenous matrix is controlled by α2β1 integrin binding and stretch-activated channels, resulting in macrophage migration toward the source of a dynamic force, such as the substrate deformation caused by contractile fibroblasts in the tissue (146, 148, 149). Finally, in vitro studies showed that macrophage infiltration into collagen-based substrates is impacted by collagen architecture (150). Taken together, these findings suggest that collagen structure and organization in tissue engineered products, such as TEM-based TEHVs, may influence adhesion, infiltration and migration of macrophages, and potentially mediating the host’s inflammatory response.
Macrophage/fibronectin interactions
FN is a large glycoprotein that can be either present in a soluble [e.g., plasma FN (129)] or non-soluble form (e.g., cellular or tissue FN) that is produced by cells such as fibroblasts and endothelial cells (151). FN fibrils are required for the deposition of collagen and for the binding of glycosaminoglycans, thereby becoming a fundamental protein to ensure ECM remodeling during the wound healing cascade (152). FN, also one of the main ECM components of TEM-based implants, has been reported to influence the adhesion, migration, and apoptosis of monocytes and fibroblasts, among other cells (151, 153). Monocyte differentiation into macrophages was enhanced when FN-coated surfaces were used, suggesting an important role for integrin-mediated adhesion in macrophage differentiation (154, 155). FN has also demonstrated influence of macrophage polarization toward a pro-inflammatory state, characterized by increased phagocytosis activity (155, 156). In addition, the pro-inflammatory effect was further elicited in macrophages that interacted with both collagen and FN, a situation that simulates cases of tissue damage where numerous ECM proteins are exposed or fragmented (157).
Based on these results, it is expected that TEM-based TEHVs, derived by in vitro culture of fibroblast-like cells, have high non-soluble FN content that may therefore influence macrophage polarization toward a pro-inflammatory status. This will lead to the recruitment and activation of more immune cells and ECM-producing αSMA+ cells, similar to what is commonly observed in a wound healing response (81, 82). In theory, macrophages will then play a key role in attracting fibroblast-like cells to stimulate TEM-based TEHV remodeling (83).
Macrophage/extracellular matrix-derived peptide interactions
ECM-derived peptides are bioactive sites of ECM proteins such as collagens, FN, and elastin, also referred to as matrikines and matricryptins. Peptides may either be generated by direct ECM damage (e.g., upon injury), or be exposed in immature ECM proteins (e.g., in a remodeling tissue) (2, 131). Importantly, these peptides are considered DAMP molecules that may have the potential to activate immune cells and/or stromal cells (e.g., fibroblasts) to initiate tissue repair through influencing cell migration, adhesion, and differentiation both in vitro and in vivo (2, 76, 134, 135).
RGD (Arg-Gly-Asp) is an integrin-binding peptide that results from exposed collagen, FN, vitronectin, and osteopontin proteins upon conformational changes as its active domain becomes apparent only upon substrate denaturation of collagens (158, 159), or absorption of FN and vitronectin (160). In areas of tissue injury, FN fibrils were shown to have an increased affinity for the exposed RGD sites of denatured collagens. It is hypothesized that this specific ECM composition, with abundance of exposed RGD sites as both proteins carry this sequence, is recognized by the immune system as a unique wound signal (161). Hence, it is not surprising that macrophages are affected by the presence of RGD domains, which stimulate the adhesion and M2 polarization both in vitro and in vivo (162). On the other hand, macrophage adhesion and fusion to FBGCs is supported by the presence of RGD peptides in combination with another FN-derived peptide, PHSRN (Pro-His-Ser-Arg-Asn) (163), which suggests that a combination of matricryptins—that could be found in a site of tissue injury—may be important in regulating a pro-inflammatory response. Finally, the inflammatory profile of macrophages and consequent fibrotic tissue formation could be mitigated when the RGD-binding integrins were blocked using specific antibodies (147), once again suggesting a pro-inflammatory effect of this peptide. Taken together, these results indicate a very active but controversial role of the RGD peptide in modulating macrophage response and polarization, making this peptide an interesting target for biomaterial functionalization (149).
VGVAPG (Val-Gly-Val-Ala-Pro-Gly) is an elastin-derived peptide that becomes exposed upon elastase and MMP12 digestion of elastin fibers. This peptide has repeatedly been shown to have chemotactic properties for monocytes, macrophages, and fibroblasts (164–166), and is also involved in ECM degradation via regulation of MMP expression (167). Specifically, the presence of elastin-derived fragments is associated with increased proliferation and decreased elastin synthesis in vascular smooth muscle cells (168). In addition, the VGVAPG peptide increased smooth muscle cell migration through the elastic lamina, thereby leading to intimal hyperplasia (168, 169). Elastin is one of the key components of vascular, valvular and heart tissue, highlighting the importance of these results for clinical translation in the cardiovascular field.
PGP (Pro-Gly-Pro) is a matricryptin derived from COL1 that plays an important role in mediating inflammation. This peptide has sequence and structural homology with one of the domains on alpha chemokines and, therefore, can mimic their chemotactic effect in inflammation models (160, 170, 171). Some bioactive ECM peptides are also involved in ECM synthesis and remodeling. Among these, the peptide GHK (Gly-His-Lys) was reported to favor wound healing and skin regeneration when combined with copper ions (Cu2+), by stimulating collagen turnover, modulating MMP activity, and attracting immune cells to the wound (172).
Taken together, these studies highlight the importance of ECM and ECM-derived peptides in regulating the early steps of the inflammatory response by directly affecting macrophages (summarized in Figure 2). Because collagens and FN are among the main components of the TEM, the presence of such peptides should be carefully evaluated to better understand the intricated steps of tissue remodeling upon implantation. The possibility of using ECM proteins and fragments to favor the TEHVs in situ remodeling potential may be an interesting strategy to influence the remodeling cascade as well as favor tissue integration and adaptive remodeling of the implant, as further discussed in section “Discussion: Relevance for in situ heart valve tissue engineering.”
Discussion: Relevance for in situ heart valve tissue engineering
The importance of ECM proteins in regulating macrophage behavior and, therefore, the consequent remodeling cascade should be considered when developing a TEHV. Macrophages are the primary mediators of host engraftment and will drive the response to the different biomaterials implanted (142), potentially deciding the fate of TEHV remodeling. Based on this, researchers are continuing to investigate ways to improve the remodeling of TEHVs with in situ regenerative potential.
Improving the characterization of decellularized tissue engineered matrices-based tissue engineered heart valves
Originally developed to achieve an off-the-shelf available immunocompatible product and to limit leaflet retraction observed in autologous cell-based TEHVs (173–175), decellularized TEM-based TEHVs have been developed. However, the TEM-based TEHVs have been reported to undergo adverse remodeling in chronic studies implanted in sheep, with leaflet thickening and shortening caused by fusion of the leaflet to the wall, and resulting in severe valvular insufficiency within 24 weeks after implantation (68, 73, 74). A possible explanation for this outcome was obtained by using computational modeling to simulate stress and strain distribution on the valve leaflet. Sanders et al. showed that the simplified geometry of TEM-based TEHVs led to radial leaflet compression when subjected to physiological (pulmonary) pressure conditions (176). Based on this, further computational simulation was used to identify an improved valve geometry that could counteract the observed leaflet retraction (176). To impose this geometry, TEM-based TEHVs were manufactured using a constraining bioreactor insert during tissue culture and tested in a preclinical sheep model (67). Upon implantation, the TEHVs demonstrated in vivo performance for up to 1 year, and underwent native-like remodeling. Remarkably, no signs of adverse remodeling (i.e., leaflet thickening or leaflet-wall fusion phenomena) were observed (67). The morphological evaluation was indicative of functional native-like remodeling, with thin and shiny leaflets, complete integration within the adjacent native pulmonary artery wall, and formation of a neo-sinus. On a microscopic scale, the authors reported extensive cellular repopulation of the entire valve, improved matrix composition with elastin deposition, as well as reorganization of the collagen fibers (67). Taken together, these results indicate that valve geometry is one of the key factors in ensuring long-term TEHV function. In a subsequent study, Motta et al. investigated how the computational-inspired TEHV geometry could impact the host cell response and, therefore, tissue remodeling (177). Focused on macrophages, αSMA-positive cells, and endothelial cells as key players of the remodeling cascade, the authors found that compared to the first simple, non-physiological geometry (74), the remodeling of computational modeling-inspired TEHVs having a physiological-like design had negligible amounts of macrophages and αSMA-positive cell infiltration, had the absence of thickening, and showed rapid endothelialization (177).
However, we can hypothesize that, other than TEHV geometry, also TEM composition may influence macrophage response and, therefore, the remodeling cascade, as summarized in Figure 3. We have previously described how ECM composition and integrity can play a role in regulating macrophage response (section “Immunoregulation of extracellular matrix proteins”). In addition, the presence of scaffold remnants may induce a foreign body response, with macrophage activation and possibly fusion to form FBGCs, as reviewed elsewhere (178). Finally, residual cellular components (i.e., DNA), may influence macrophage response and, therefore, the remodeling cascade, as detailed below.
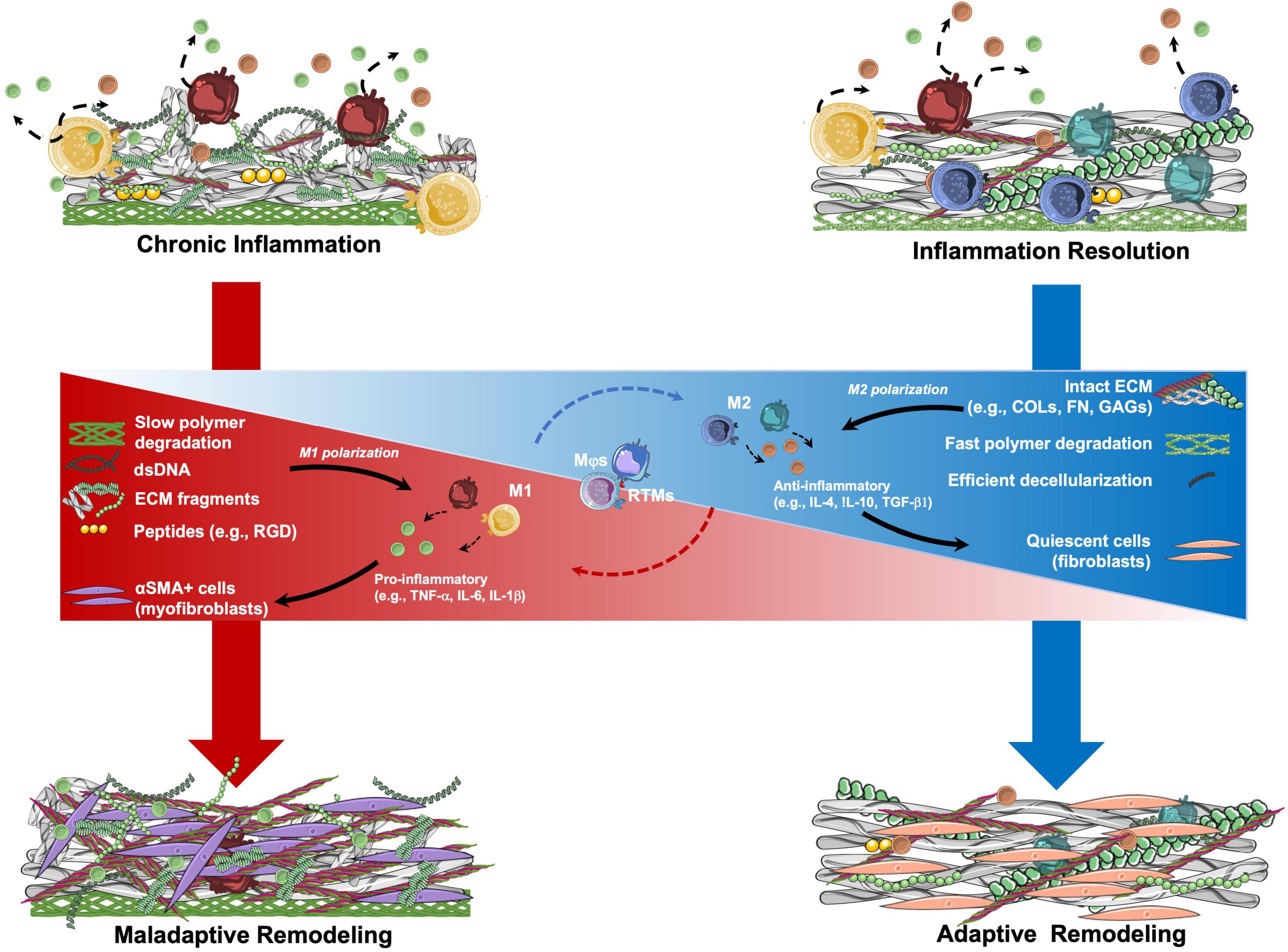
Figure 3. Hypothetical mechanisms involved in the remodeling of tissue engineered matrix (TEM)-based implants. TEM composition may be a crucial parameter in determining the remodeling potential of TEM-based implants. Resident tissue macrophages (RTMs) and circulation-derived macrophages (Mφs) are key mediators of the inflammatory process. Once in contact with TEMs, RTMs and Mφs may start a pro- or anti-inflammatory signaling by polarizing between M1 and M2 states, respectively. The sustained presence of polymer remnants, ECM fragments, and double stranded DNA (dsDNA) residues from incomplete decellularization, may lead to pro-inflammatory stimulation of RTMs and Mφs with consequent M1 polarization with the release of pro-inflammatory factors. This pro-inflammatory state may lead to the activation of fibroblasts into myofibroblasts, with the consequent production of a collagen-rich ECM, similarly to what is observed in tissue fibrosis (maladaptive remodeling). In contrast, TEMs having intact ECM proteins (e.g., COLs, FN, GAGs) as well as low amounts of dsDNA after optimal decellularization, may determine a short pro-inflammatory phase followed by M1 polarization resolution and transition to the M2 state. M2 RTMs and Mφs may help with provisional matrix formation. Recruited fibroblasts populate the implant and produce organized ECM until resolution of the inflammation occurs (adaptive remodeling). αSMA, alpha smooth muscle actin; COLs, collagens; FN, fibronectin; GAGs, glycosaminoglycans; IL, interleukin; RGD, Arg-Gly-Asp; TGF, transforming growth factor; TNF, tissue necrosis factor.
The immunocompatibility of biomaterials made from decellularized (native or in vitro grown) tissues may be affected by the degree of decellularization, as the presence of cell debris may influence the immune response upon implantation. Decellularization protocols, as reviewed elsewhere (179, 180), generally aim at lysing resident cells to drastically reduce the immunogenic components of the starting tissue (i.e., DNA remnants) using a combination of chemical, physical, and enzymatic reactions, all while preserving the ECM. However, complete removal of all cellular components has not been shown so far. The presence of several antigens and/or DAMPs released from lysed cells (e.g., calcium-binding proteins, DNA, ATP, chromatin, nuclear proteins), which has been reported to potentially cause an adverse immune response upon implantation (181). Therefore, decellularized tissues should comply with the quantitative criteria described in 2011 (182): no visible cell nuclei in H&E or DAPI staining; double stranded DNA content < 50 ng/mg of dry tissue; and DNA remnant size < 300 base pairs. It has been shown that residual cellular debris, such as DNA, mitochondria and cell membrane proteins, can promote a pro-inflammatory M1 macrophage phenotype both in vitro and in vivo (183). This is particularly striking as clinical-grade biological decellularized tissues have great variations in the amount of retained cell remnants, a parameter that may cause differences in the tissue remodeling outcome upon implantation and device efficacy (184). Indeed, the DNA amount and degree of fragmentation within decellularized tissues was reported to influence macrophage phenotype both in vitro and in vivo, with a more effective decellularization being associated with a shift from an M1 to M2-like phenotype (185, 186).
On the other hand, extensive decellularization protocols can alter the three-dimensional structure of the ECM proteins [i.e., glycoproteins, proteoglycans, fibrinogen and fibronectin domains, tenascin c, etc. (136)], thereby creating further DAMPs (187). Therefore, maintaining the integrity of ECM proteins upon decellularization is important to ensure not only sufficient mechanical properties of the tissue, but also to promote an anti-inflammatory effect (185).
Several in vitro studies have investigated how decellularized native tissues can modulate macrophages polarization. For example, ECM scaffolds obtained from decellularized porcine small intestine submucosa (SIS) proved to favor a M2-like macrophage phenotype, with anti-inflammatory and pro-remodeling characteristics (183, 186, 188). On the other hand, dermal tissue-derived ECM scaffolds promoted a pro-inflammatory M1-like phenotype (183). However, the cause of macrophage polarization variation on the different substrates was not clarified. Importantly, macrophage polarization is often not quantitatively apparent in vivo and results may differ significantly between pre-clinical and clinical data (186). In this regard, the clinical use of decellularized porcine, SIS-based or valve-based, TEHVs resulted in severe adverse events for pediatric applications (47, 53). Remarkably, such an adverse effect was not observed during pre-clinical investigations in sheep models (189–192), as extensively reviewed elsewhere (18). These results suggest that decellularized xenograft-based valvular replacements may still contain α-galactose (25), a pro-inflammatory epitope that, particularly in pediatric patients, may have caused the adverse inflammatory response resulting in valve calcification and degeneration (47, 53). Therefore, independent of tissue origin, xenograft material may elicit a strong inflammatory response with detrimental consequences.
Taken together, these results highlight the limitations of both in vitro and in vivo preclinical models and suggest the need for an improved testing platform to assess immunocompatibility of decellularized products.
Extracellular matrix-based functionalization of bioresorbable polymeric tissue engineered heart valves
Among the different types of TEHVs with in situ remodeling potential, polymer-based TEHVs have generated considerable interest and their clinical translation is currently ongoing with however, mixed outcomes so far, as discussed in section “In situ heart valve tissue engineering.” While conceptually, such materials may have multiple advantages (e.g., reasonable cost, scalability, tenability of degradation, mechanical properties and architecture), long-term safety and efficacy of this concept, especially when the initial polymer is fully resorbed, remains to be elucidated.
Nevertheless, researchers are constantly trying to improve their polymer-based scaffolds by promoting cell recruitment and endogenous tissue formation. One way to achieve this goal is to implement microstructural design features (i.e., pore size or polymer fiber orientation) to resemble native ECM architecture of the heart valve (62, 193), as reviewed elsewhere (38). Scaffold microarchitecture can be further combined with polymer functionalization using ECM proteins and ECM-derived molecules (55, 194). It was found that non-covalent scaffold functionalization was successfully achieved by developing a hybrid polymer, which combined the durability of synthetic polymers with the biocompatibility of gelatin (55). By using a cell-free rapid jet-spinning manufacturing process, the resulting TEHV had not only mimicked the fibrous, anisotropic architecture of the native leaflet with the synthetic polymers, but also had enhanced implant biocompatibility that favored cellular attachment and infiltration with the use of gelatin. These gelatin-functionalized TEHVs have been successfully implanted in an acute sheep model, demonstrating functionality in vitro as well as in vivo (55). However, long-term comparative studies are needed to understand the benefits of gelatin functionalization may have on the remodeling potential of this hybrid TEHV.
Considering its significant role in mediating macrophage function, FN has been frequently used in biomaterial surface modification to favor implant integration and cell adhesion (142). However, the impact of FN pre-adsorption in controlling macrophage adhesion and cytokine release is often overruled by the material surface chemistry, thereby suggesting the need to better understand the macrophage response to both the material and the proteins that are immobilized on the material (195).
To date, there are no other studies where bioresorbable polymeric TEHV functionalization was evaluated in large animal models. However, in the cardiovascular field, a multitude of growth factors in combination with ECM proteins have been proposed to favor tissue integration and remodeling, as extensively reviewed elsewhere (194, 196). For example, VEGF was demonstrated to significantly inhibit the formation of calcifications in valve interstitial cells, whereas TGFβ1 stimulated calcific nodules in vitro (197). Remarkably, the use of FN coating proved to significantly reduce calcification formation despite TGFβ1 administration (197). These results suggest that, to limit the risk of calcifications, specific ECM proteins and growth factors, like FN and VEGF, can be combined to functionalize scaffolds for TEHV applications. Heparin and IL-4 have also been proposed to functionalize polymer. In vitro results showed that this functionalization effectively promoted M2 macrophage polarization and created an anti-inflammatory environment in electrospun scaffolds (112). Similarly, the use of a cytokine cocktail (i.e., IL-10 and prostaglandin-E2) was shown to promote tissue integration and to polarize macrophages into M2 pro-healing phenotype, thereby decreasing adverse immune reactions (198), suggesting a potential use of an IL-4 and IL-10 combination to improve material integration and performance.
The use of peptides to functionalize scaffolds for cardiovascular applications has been extensively reviewed elsewhere (149, 199, 200). Peptides such as RGD and REDV are mostly used to favor endothelialization of the construct, as the sequences can be specifically recognized by the endothelial cells (199, 200). However, as discussed in section “Macrophage/extracellular matrix-derived peptide interactions,” their impact on macrophage adhesion and polarization should be further investigated. A stromal cell derived factor 1α (SDF1α)-derived peptide has been proposed as a potential chemokine to attract monocytes and progenitor cells and to modulate tissue remodeling. In vitro studies using SDF1α-derived peptides for polymer functionalization showed reduced expression of inflammatory factors, indicating a reduction in inflammatory signaling. In vivo implantation of these scaffolds as rat abdominal aorta interposition grafts showed increased presence of macrophages after 7 days, thereby suggesting the potential role SDF1α-derived peptides may have in modulating the immune response (201). However, scaffold functionalization still comes with some limitations, such as the need to ensure the functionality of the bioactive molecule included and the reduced shelf-life of the product due to diffusion of the included protein (55).
Conclusion
ECM proteins are a potent tool for the immunoregulatory function upon implantation of a tissue engineered heart valve. A multitude of studies suggest the potential role of ECM and ECM-related proteins in regulating macrophage polarization both in vitro and in vivo. However, their effect on the adaptive or maladaptive remodeling of TEM-based TEHVs should be further considered. Based on these observations, several functionalization approaches for bioresorbable polymeric TEHVs, either using ECM proteins, cytokine/growth factor cocktails, cells capable of secreting ECM-related cytokines, or ECM-derived peptides, have been evaluated both in vitro and in vivo. However, to this date, none of these approaches have reached clinical translation.
The pre-clinical use of TEM-based TEHVs for pulmonary applications has shown great potential, and their translation to aortic application is awaited. A full understanding of how ECM-based biomaterials specifically engage with the host tissue has not yet been fully elucidated. However, it is crucial to determine how the host responds to the implanted TEM-based biomaterial based on its protein composition, ECM architecture, and 3D structure, to ensure safe clinical translation. In particular, guidelines on what potential contaminants should be completely eliminated or a threshold for potential immunogenic proteins should be outlined and standardized. In addition, extensive ECM characterization of the final product, including identification of pro-inflammatory ECM components and DAMPs, should be performed. With consistent material production and comprehensive regulations on decellularized biomaterials, TEM-based TEHVs may soon become the next-generation heart valve prosthesis.
Author contributions
NP, MM, and EF drafted the manuscript. All authors reviewed the text and provided critical input to the manuscript.
Funding
This manuscript received funding through the Swiss National Science Foundation (SNSF), (Grant no. PZ00P3_180138) (MIRAvalve). EF and NP were supported by the SNSF MIRAvalve grant. ME received funding from the European Research Council (ERC) under the European Union’s Horizon 2020 Research and Innovation Program (Grant no. 852814) (TAVI4Life).
Conflict of interest
Author SH was a shareholder at Xeltis BV and LifeMatrix AG. Author ME was a shareholder at LifeMatrix AG.
The remaining authors declare that the research was conducted in the absence of any commercial or financial relationships that could be construed as a potential conflict of interest.
Publisher’s note
All claims expressed in this article are solely those of the authors and do not necessarily represent those of their affiliated organizations, or those of the publisher, the editors and the reviewers. Any product that may be evaluated in this article, or claim that may be made by its manufacturer, is not guaranteed or endorsed by the publisher.
Abbreviations
αSMA, α-smooth muscle actin; CAVD, calcific aortic valve disease; COL, collagen; DAMP, damage-associated molecular pattern; ECM, extracellular matrix; FGF, fibroblast growth factor; FN, fibronectin; IL, interleukin; MMP, matrix metalloproteinases; P4HB, poly-4-hydroxybutyrate; PDGF, platelet derived growth factor; PGA, polyglycolic acid; RTM, resident tissue macrophage; TEHV, tissue engineered heart valves; TEM, tissue engineered matrix; TGFβ1, transforming growth factor-β1; VEGF, vascular endothelial growth factor.
References
1. Werner S, Grose R. Regulation of wound healing by growth factors and cytokines. Physiol Rev. (2003) 83:835–70. doi: 10.1152/physrev.2003.83.3.835
2. Tomlin H, Piccinini AM. A complex interplay between the extracellular matrix and the innate immune response to microbial pathogens. Immunology. (2018) 155:186–201. doi: 10.1111/imm.12972
3. Balachandran K, Sucosky P, Yoganathan AP. Hemodynamics and mechanobiology of aortic valve inflammation and calcification. Int J Inflamm. (2011) 2011:1–15. doi: 10.4061/2011/263870
4. Lincoln J, Lange AW, Yutzey KE. Hearts and bones: shared regulatory mechanisms in heart valve, cartilage, tendon, and bone development. Dev Biol. (2006) 294:292–302. doi: 10.1016/j.ydbio.2006.03.027
5. Hinton RB, Yutzey KE. Heart valve structure and function in development and disease. Annu Rev Physiol. (2011) 73:29–46. doi: 10.1146/annurev-physiol-012110-142145
6. Sacks MS, David Merryman W, Schmidt DE. On the biomechanics of heart valve function. J Biomech. (2009) 42:1804–24. doi: 10.1016/j.jbiomech.2009.05.015
7. Hinton RB, Lincoln J, Deutsch GH, Osinska H, Manning PB, Benson DW, et al. Extracellular matrix remodeling and organization in developing and diseased aortic valves. Circ Res. (2006) 98:1431–8. doi: 10.1161/01.RES.0000224114.65109.4e
8. Ewart AK, Morris CA, Atkinson D, Jin W, Sternes K, Spallone P, et al. Hemizygosity at the elastin locus in a developmental disorder, Williams syndrome. Nat Genet. (1993) 5:11–6. doi: 10.1038/ng0993-11
9. Dietz HC, Cutting CR, Pyeritz RE, Maslen CL, Sakai LY, Corson GM, et al. Marfan syndrome caused by a recurrent de novo missense mutation in the fibrillin gene. Nature. (1991) 352:337–9. doi: 10.1038/352337a0
10. Ahmad NN, Ala-Kokko L, Knowlton RG, Jimenez SA, Weaver EJ, Maguire JI, et al. Stop codon in the procollagen II gene (COL2A1) in a family with the Stickler syndrome (arthro-ophthalmopathy). Proc Natl Acad Sci U.S.A. (1991) 88:6624–7. doi: 10.1073/pnas.88.15.6624
11. Byers PH. Ehlers-Danlos syndrome: recent advances and current understanding of the clinical and genetic heterogeneity. J Invest Dermatol. (1994) 103:47S–52S. doi: 10.1111/1523-1747.ep12399038
12. Lerman DA, Prasad S, Alotti N. Calcific aortic valve disease: molecular mechanisms and therapeutic approaches. Eur Cardiol Rev. (2015) 10:108–12. doi: 10.15420/ecr.2015.10.2.108
13. Alushi B, Vathie K, Thiele H, Lauten A. Transcatheter therapies for severe tricuspid regurgitation. Quo vadis? Herz. (2021) 46:234–41. doi: 10.1007/s00059-020-04941-z
14. Bartoli-Leonard F, Zimmer J, Aikawa E. Innate and adaptive immunity: the understudied driving force of heart valve disease. Cardiovasc Res. (2021) 117:2506–24. doi: 10.1093/cvr/cvab273
15. Perrotta I, Russo E, Camastra C, Filice G, di Mizio G, Colosimo F, et al. New evidence for a critical role of elastin in calcification of native heart valves: immunohistochemical and ultrastructural study with literature review. Histopathology. (2011) 59:504–13. doi: 10.1111/j.1365-2559.2011.03977.x
16. Schoen FJ. Mechanisms of function and disease of natural and replacement heart valves. Annu Rev Pathol Mech Dis. (2012) 7:161–83. doi: 10.1146/annurev-pathol-011110-130257
17. Fanning JP, Platts DG, Walters DL, Fraser JF. Transcatheter aortic valve implantation (TAVI): valve design and evolution. Int J Cardiol. (2013) 168:1822–31. doi: 10.1016/j.ijcard.2013.07.117
18. Fioretta ES, Motta SE, Lintas V, Loerakker S, Parker KK, Baaijens FPT, et al. Next-generation tissue-engineered heart valves with repair, remodelling and regeneration capacity. Nat Rev Cardiol. (2021) 18:92–116. doi: 10.1038/s41569-020-0422-8
19. Russo M, Taramasso M, Guidotti A, Pozzoli A, Nietilspach F, Von Segesser L, et al. The evolution of surgical valves. Cardiovasc Med. (2017) 20:285–92. doi: 10.4414/cvm.2017.00532
20. Cribier A, Eltchaninoff H, Bash A, Borenstein N, Tron C, Bauer F, et al. Percutaneous transcatheter implantation of an aortic valve prosthesis for calcific aortic stenosis: first human case description. Circulation. (2002) 106:3006–8. doi: 10.1161/01.cir.0000047200.36165.b8
21. Piazza N, Bleiziffer S, Brockmann G, Hendrick R, Deutsch M-A, Opitz A, et al. Transcatheter aortic valve implantation for failing surgical aortic bioprosthetic valve. JACC Cardiovasc Interv. (2011) 4:721–32. doi: 10.1016/j.jcin.2011.03.016
22. Yacoub MH, Takkenberg JJM. Will heart valve tissue engineering change the world? Nat Clin Pract Cardiovasc Med. (2005) 2:60–1. doi: 10.1038/ncpcardio0112
23. Soliman Hamad MA, van Eekelen E, van Agt T, van Straten AHM. Self-management program improves anticoagulation control and quality of life: a prospective randomized study. Eur J Cardio Thorac Surg. (2009) 35:265–9. doi: 10.1016/j.ejcts.2008.10.020
24. Rabkin-Aikawa E, Mayer JE, Schoen FJ. Heart valve regeneration. Adv Biochem Eng Biotechnol. (2005) 94:141–79.
25. Choi S-Y, Jeong H-J, Lim H-G, Park S-S, Kim S-H, Kim YJ. Elimination of alpha-gal xenoreactive epitope: alpha-galactosidase treatment of porcine heart valves. J Heart Valve Dis. (2012) 21:387–97.
26. Leopold JA. Cellular mechanisms of aortic valve calcification. Circ Cardiovasc Interv. (2012) 5:605–14. doi: 10.1161/CIRCINTERVENTIONS.112.971028
27. Head SJ, Çelik M, Kappetein AP. Mechanical versus bioprosthetic aortic valve replacement. Eur Heart J. (2017) 38:2183–91. doi: 10.1093/eurheartj/ehx141
28. Huygens SA, Goossens LMA, Van Erkelens JA, Takkenberg JJM, Rutten-Van Mölken MPMH. How much does a heart valve implantation cost and what are the health care costs afterwards? Open Heart. (2018) 5:e000672. doi: 10.1136/openhrt-2017-000672
29. Cebotari S, Lichtenberg A, Tudorache I, Hilfiker A, Mertsching H, Leyh R, et al. Clinical application of tissue engineered human heart valves using autologous progenitor cells. Circulation. (2006) 114:132–8. doi: 10.1161/CIRCULATIONAHA.105.001065
30. Dohmen PM, Lembcke A, Holinski S, Kivelitz D, Braun JP, Pruss A, et al. Mid-term clinical results using a tissue-engineered pulmonary valve to reconstruct the right ventricular outflow tract during the ross procedure. Ann Thorac Surg. (2007) 84:729–36. doi: 10.1016/j.athoracsur.2007.04.072
31. Dohmen P, da Costa F. An experimental study of decellularized xenografts implanted into the aortic position with 4 months of follow up. J Clin Exp Cardiol. (2012) 4:2. doi: 10.4172/2155-9880.s4-004
32. Erdbrügger W, Konertz W, Dohmen PM, Posner S, Ellerbrok H, Brodde O-E, et al. Decellularized xenogenic heart valves reveal remodeling and growth potential in vivo. Tissue Eng. (2006) 12:2059–68. doi: 10.1089/ten.2006.12.2059
33. Bennink G, Mroczek T, Sivalingam S, Prodan Z, Herrington C, Bacha E, et al. A Novel Pulmonary Valve Homing Device – Early Feasibility Study (EFS-US) and First in Man (FIM-EU/Asia) 1&2 Year Results. International Conference of Tissue Engineered Heart Valves. (2020). Available online at: https://ictehv.com/abstracts/a-novel-pulmonary-valve-homing-device-early-feasibility-study-efs-us-and-first-in-man-fim-euasia-year-results (accessed April 13, 2020).
34. US National Library of Medicine. Study to Assess Safety of the Pulmonary Valved Conduit (PV-001) in Subjects Undergoing Right Ventricular Outflow Tract Reconstruction. (2016). Available online at: https://clinicaltrials.gov/ct2/show/NCT02700100 (accessed April 13, 2020).
35. US National Library of Medicine. Xeltis Bioabsorbable Pulmonary Valved Conduit Pivotal Study (Xplore2). (2017). Available online at: https://clinicaltrials.gov/ct2/show/NCT03022708 (accessed April 13, 2020).
36. Wissing TB, Bonito V, Bouten CVC, Smits AIPM. Biomaterial-driven in situ cardiovascular tissue engineering—a multi-disciplinary perspective. NPJ Regen Med. (2017) 2:18. doi: 10.1038/s41536-017-0023-2
37. Smits AIPM, Bouten CVC. Tissue engineering meets immunoengineering: prospective on personalized in situ tissue engineering strategies. Curr Opin Biomed Eng. (2018) 6:17–26. doi: 10.1016/J.COBME.2018.02.006
38. de Kort BJ, Koch SE, Wissing TB, Krebber MM, Bouten CVC, Smits AIPM. Immuno-regenerative biomaterials for in situ cardiovascular tissue engineering – Do patient characteristics warrant precision engineering? Adv Drug Deliv Rev. (2021) 178:113960. doi: 10.1016/j.addr.2021.113960
39. Da Costa FDA, Costa ACBA, Prestes R, Domanski AC, Balbi EM, Ferreira ADA, et al. The early and midterm function of decellularized aortic valve allografts. Ann Thorac Surg. (2010) 90:1854–60. doi: 10.1016/j.athoracsur.2010.08.022
40. Miller DV, Edwards WD, Zehr KJ. Endothelial and smooth muscle cell populations in a decellularized cryopreserved aortic homograft (SynerGraft) 2 years after implantation. J Thorac Cardiovasc Surg. (2006) 132:175–6. doi: 10.1016/j.jtcvs.2006.02.038
41. Cebotari S, Tudorache I, Ciubotaru A, Boethig D, Sarikouch S, Goerler A, et al. Use of fresh decellularized allografts for pulmonary valve replacement may reduce the reoperation rate in children and young adults: early report. Circulation. (2011) 124:115–24. doi: 10.1161/CIRCULATIONAHA.110.012161
42. Sarikouch S, Horke A, Tudorache I, Beerbaum P, Westhoff-Bleck M, Boethig D, et al. Decellularized fresh homografts for pulmonary valve replacement: a decade of clinical experience. Eur J Cardio Thorac Surg. (2016) 50:281–90. doi: 10.1093/ejcts/ezw050
43. Helder MRKK, Kouchoukos NT, Zehr K, Dearani JA, Maleszewski JJ, Leduc C, et al. Late durability of decellularized allografts for aortic valve replacement: a word of caution. J Thorac Cardiovasc Surg. (2016) 152:1197–9. doi: 10.1016/j.jtcvs.2016.03.050
44. Konertz W, Angeli E, Tarusinov G, Christ T, Kroll J, Dohmen P, et al. Right ventricular outflow tract reconstruction with decellularized porcine xenografts in patients with congenital heart disease. J Hear Valve Dis. (2011) 20:341–7.
45. Sayk F, Bos I, Schubert U, Wedel T, Sievers H-H. Histopathologic findings in a novel decellularized pulmonary homograft: an autopsy study. Ann Thorac Surg. (2005) 79:1755–8. doi: 10.1016/j.athoracsur.2003.11.049
46. Zafar F, Hinton RB, Moore RA, Baker RS, Bryant R, Narmoneva DA, et al. Physiological growth, remodeling potential, and preserved function of a novel bioprosthetic tricuspid valve: tubular bioprosthesis made of small intestinal submucosa-derived extracellular matrix. J Am Coll Cardiol. (2015) 66:877–88. doi: 10.1016/j.jacc.2015.06.1091
47. Woo JS, Fishbein MC, Reemtsen B. Histologic examination of decellularized porcine intestinal submucosa extracellular matrix (CorMatrix) in pediatric congenital heart surgery. Cardiovasc Pathol. (2016) 25:12–7. doi: 10.1016/j.carpath.2015.08.007
48. Christ T, Paun AC, Grubitzsch H, Holinski S, Falk V, Dushe S. Long-term results after the ross procedure with the decellularized autotissue matrix P§bioprosthesis used for pulmonary valve replacement. Eur J Cardio Thorac Surg. (2019) 55:885–92. doi: 10.1093/ejcts/ezy377
49. Mosala Nezhad Z, Baldin P, Poncelet A, El Khoury G. Calcific degeneration of CorMatrix 4 years after bicuspidization of unicuspid aortic valve. Ann Thorac Surg. (2017) 104:e431–3. doi: 10.1016/j.athoracsur.2017.07.040
50. Padalino MA, Castaldi B, Fedrigo M, Gallo M, Zucchetta F, Vida VL, et al. Porcine intestinal submucosa (CorMatrix) for semilunar valve repair in children: a word of caution after midterm results. Semin Thorac Cardiovasc Surg. (2016) 28:436–45. doi: 10.1053/j.semtcvs.2016.04.015
51. Hofmann M, Schmiady MO, Burkhardt BE, Dave HH, Hübler M, Kretschmar O, et al. Congenital aortic valve repair using CorMatrix§: a histologic evaluation. Xenotransplantation. (2017) 24:e12341. doi: 10.1111/xen.12341
52. Zaidi AH, Nathan M, Emani S, Baird C, Del Nido PJ, Gauvreau K, et al. Preliminary experience with porcine intestinal submucosa (CorMatrix) for valve reconstruction in congenital heart disease: histologic evaluation of explanted valves. J Thorac Cardiovasc Surg. (2014) 148:2216–25.e1. doi: 10.1016/j.jtcvs.2014.02.081
53. Simon P, Kasimir MT, Seebacher G, Weigel G, Ullrich R, Salzer-Muhar U, et al. Early failure of the tissue engineered porcine heart valve SYNERGRAFTTM in pediatric patients. Eur J Cardiothorac Surg. (2003) 23:1002–6; discussion 1006. doi: 10.1016/S1010-7940(03)00094-0
54. Soliman OI, Miyazaki Y, Abdelghani M, Brugmans M, Witsenburg M, Onuma Y, et al. Midterm performance of a novel restorative pulmonary valved conduit: preclinical results. EuroIntervention. (2017) 13:e1418–27. doi: 10.4244/EIJ-D-17-00553
55. Capulli AK, Emmert MY, Pasqualini FS, Kehl D, Caliskan E, Lind JU, et al. JetValve: rapid manufacturing of biohybrid scaffolds for biomimetic heart valve replacement. Biomaterials. (2017) 133:229–41. doi: 10.1016/j.biomaterials.2017.04.033
56. Kluin J, Talacua H, Smits AIPM, Emmert MY, Brugmans MCP, Fioretta ES, et al. In situ heart valve tissue engineering using a bioresorbable elastomeric implant – From material design to 12 months follow-up in sheep. Biomaterials. (2017) 125:101–17. doi: 10.1016/j.biomaterials.2017.02.007
57. Miyazaki Y, Soliman O, Abdelghani M, Katsikis A, Naz C, Lopes S, et al. Acute performance of a novel restorative transcatheter aortic valve: preclinical results. EuroIntervention. (2017) 13:e1410–7. doi: 10.4244/EIJ-D-17-00554
58. Bennink G, Torii S, Brugmans M, Cox M, Svanidze O, Ladich E, et al. A novel restorative pulmonary valved conduit in a chronic sheep model: mid-term hemodynamic function and histologic assessment. J Thorac Cardiovasc Surg. (2018) 155:2591–601.e3. doi: 10.1016/j.jtcvs.2017.12.046
59. Coyan GN, D’Amore A, Matsumura Y, Pedersen DD, Luketich SK, Shanov V, et al. In vivo functional assessment of a novel degradable metal and elastomeric scaffold-based tissue engineered heart valve. J Thorac Cardiovasc Surg. (2019) 157:1809–16. doi: 10.1016/j.jtcvs.2018.09.128
60. Morales DL, Herrington C, Bacha EA, Morell VO, Prodán Z, Mroczek T, et al. Novel restorative pulmonary valve conduit: early outcomes of two clinical trials. Front Cardiovasc Med. (2021) 7:583360. doi: 10.3389/FCVM.2020.583360
61. Fioretta ES, Lintas V, Mallone A, Motta SE, von Boehmer L, Dijkman PE, et al. Differential leaflet remodeling of bone marrow cell pre-seeded versus nonseeded bioresorbable transcatheter pulmonary valve replacements. JACC Basic Transl Sci. (2020) 5:15–31. doi: 10.1016/j.jacbts.2019.09.008
62. Uiterwijk M, Smits AIPM, van Geemen D, van Klarenbosch B, Dekker S, Cramer MJ, et al. In situ remodeling overrules bioinspired scaffold architecture of supramolecular elastomeric tissue-engineered heart valves. JACC Basic Transl Sci. (2020) 5:1187–206. doi: 10.1016/j.jacbts.2020.09.011
63. De Kort BJ, Marzi J, Brauchle EM, Lichauco AM, Bauer HS, Serrero A, et al. Inflammatory and regenerative processes in bioresorbable synthetic pulmonary valves up to two years in sheep–spatiotemporal insights augmented by Raman microspectroscopy. Acta Biomater. (2021) 135:243–59. doi: 10.1016/j.actbio.2021.09.005
64. Lintas V, Fioretta ES, Motta SE, Dijkman PE, Pensalfini M, Mazza E, et al. Development of a novel human cell-derived tissue-engineered heart valve for transcatheter aortic valve replacement: an in vitro and in vivo feasibility study. J Cardiovasc Transl Res. (2018) 11:470–82. doi: 10.1007/s12265-018-9821-1
65. Motta SE, Lintas V, Fioretta ES, Dijkman PE, Putti M, Caliskan E, et al. Human cell-derived tissue-engineered heart valve with integrated valsalva sinuses: towards native-like transcatheter pulmonary valve replacements. NPJ Regen Med. (2019) 4:14. doi: 10.1038/s41536-019-0077-4
66. Dijkman PE, Driessen-Mol A, Frese L, Hoerstrup SP, Baaijens FPT. Decellularized homologous tissue-engineered heart valves as off-the-shelf alternatives to xeno- and homografts. Biomaterials. (2012) 33:4545–54. doi: 10.1016/j.biomaterials.2012.03.015
67. Emmert MY, Schmitt BA, Loerakker S, Sanders B, Spriestersbach H, Fioretta ES, et al. Computational modeling guides tissue-engineered heart valve design for long-term in vivo performance in a translational sheep model. Sci Transl Med. (2018) 10:eaan4587. doi: 10.1126/scitranslmed.aan4587
68. Motta SE, Fioretta ES, Dijkman PE, Lintas V, Behr L, Hoerstrup SP, et al. Development of an off-the-shelf tissue-engineered sinus valve for transcatheter pulmonary valve replacement: a proof-of-concept study. J Cardiovasc Transl Res. (2018) 11:182–91. doi: 10.1007/s12265-018-9800-6
69. Syedain ZH, Meier LA, Bjork JW, Lee A, Tranquillo RT. Implantable arterial grafts from human fibroblasts and fibrin using a multi-graft pulsed flow-stretch bioreactor with noninvasive strength monitoring. Biomaterials. (2011) 32:714–22. doi: 10.1016/j.biomaterials.2010.09.019
70. Syedain ZH, Graham ML, Dunn TB, O’Brien T, Johnson SL, Schumacher RJ, et al. A completely biological “off-the-shelf” arteriovenous graft that recellularizes in baboons. Sci Transl Med. (2017) 9:eaan4209. doi: 10.1126/scitranslmed.aan4209
71. Syedain Z, Reimer J, Schmidt J, Lahti M, Berry J, Bianco R, et al. 6-Month aortic valve implantation of an off-the-shelf tissue-engineered valve in sheep. Biomaterials. (2015) 73:175–84. doi: 10.1016/j.biomaterials.2015.09.016
72. Reimer JM, Syedain ZH, Haynie BHT, Tranquillo RT. Pediatric tubular pulmonary heart valve from decellularized engineered tissue tubes. Biomaterials. (2015) 62:88–94. doi: 10.1016/j.biomaterials.2015.05.009
73. Reimer J, Syedain Z, Haynie B, Lahti M, Berry J, Tranquillo R. Implantation of a tissue-engineered tubular heart valve in growing lambs. Ann Biomed Eng. (2017) 45:439–51. doi: 10.1007/s10439-016-1605-7
74. Driessen-Mol A, Emmert MY, Dijkman PE, Frese L, Sanders B, Weber B, et al. Transcatheter implantation of homologous “off-the-shelf” tissue-engineered heart valves with self-repair capacity: long-term functionality and rapid in vivo remodeling in sheep. J Am Coll Cardiol. (2014) 63:1320–9. doi: 10.1016/j.jacc.2013.09.082
75. Morris AH, Stamer DK, Kyriakides TR. The host response to naturally-derived extracellular matrix biomaterials. Semin Immunol. (2017) 29:72–91. doi: 10.1016/j.smim.2017.01.002
76. Barker TH. The role of ECM proteins and protein fragments in guiding cell behavior in regenerative medicine. Biomaterials. (2011) 32:4211–4. doi: 10.1016/j.biomaterials.2011.02.027
77. Stephens EH, Nguyen TC, Blazejewski JG, Vekilov DP, Connell JP, Itoh A, et al. Extracellular matrix remodeling in wound healing of critical size defects in the mitral valve leaflet. Heart Vessels. (2016) 31:1186–95. doi: 10.1007/s00380-015-0768-8
78. Han L, Gotlieb AI. Fibroblast growth factor-2 promotes in vitro heart valve interstitial cell repair through the Akt1 pathway. Cardiovasc Pathol. (2012) 21:382–9. doi: 10.1016/j.carpath.2011.12.001
79. Tamura K, Murakami M, Washizu M. Healing of wound sutures on the mitral valve: an experimental study. Gen Thorac Cardiovasc Surg. (2007) 55:98–104. doi: 10.1007/s11748-006-0085-3
80. Kao WJ. Evaluation of protein-modulated macrophage behavior on biomaterials: designing biomimetic materials for cellular engineering. Biomaterials. (1999) 20:2213–21. doi: 10.1016/S0142-9612(99)00152-0
81. Xia Z, Triffitt JT. A review on macrophage responses to biomaterials. Biomed Mater. (2006) 1:R1–9. doi: 10.1088/1748-6041/1/1/R01
82. Gurtner GC, Werner S, Barrandon Y, Longaker MT. Wound repair and regeneration. Nature. (2008) 453:314–21. doi: 10.1038/nature07039
83. Han L, Gotlieb AI. Fibroblast growth factor-2 promotes in vitro mitral valve interstitial cell repair through transforming growth factor-β/smad signaling. Am J Pathol. (2011) 178:119–27. doi: 10.1016/j.ajpath.2010.11.038
84. Liu AC, Joag VR, Gotlieb AI. The emerging role of valve interstitial cell phenotypes in regulating heart valve pathobiology. Am J Pathol. (2007) 171:1407–18. doi: 10.2353/ajpath.2007.070251
85. McNally AK, Anderson JM. β1 and β2 integrins mediate adhesion during macrophage fusion and multinucleated foreign body giant cell formation. Am J Pathol. (2002) 160:621–30. doi: 10.1016/S0002-9440(10)64882-1
86. Gentek R, Molawi K, Sieweke MH. Tissue macrophage identity and self-renewal. Immunol Rev. (2014) 262:56–73. doi: 10.1111/imr.12224
87. Lyadova I, Gerasimova T, Nenasheva T. Macrophages derived from human induced pluripotent stem cells: the diversity of protocols, future prospects, and outstanding questions. Front Cell Dev Biol. (2021) 9:924. doi: 10.3389/fcell.2021.640703
88. Wynn TA, Vannella KM. Macrophages in tissue repair, regeneration, and fibrosis. Immunity. (2016) 44:450–62. doi: 10.1016/j.immuni.2016.02.015
89. Olingy CE, San Emeterio CL, Ogle ME, Krieger JR, Bruce AC, Pfau DD, et al. Non-classical monocytes are biased progenitors of wound healing macrophages during soft tissue injury. Sci Rep. (2017) 7:447. doi: 10.1038/s41598-017-00477-1
90. Rayahin JE, Gemeinhart RA. Activation of macrophages in response to biomaterials. Results Probl Cell Differ. (2017) 62:317–51. doi: 10.1007/978-3-319-54090-0_13
91. Yao Y, Xu XH, Jin L. Macrophage polarization in physiological and pathological pregnancy. Front Immunol. (2019) 10:792. doi: 10.3389/fimmu.2019.00792
92. Roszer T. Understanding the mysterious M2 macrophage through activation markers and effector mechanisms. Mediators Inflamm. (2015) 2015:816460. doi: 10.1155/2015/816460
93. Darby IA, Zakuan N, Billet F, Desmoulière A. The myofibroblast, a key cell in normal and pathological tissue repair. Cell Mol Life Sci. (2016) 73:1145–57. doi: 10.1007/s00018-015-2110-0
94. Schnoor M, Cullen P, Lorkowski J, Stolle K, Robenek H, Troyer D, et al. Production of type VI collagen by human macrophages: a new dimension in macrophage functional heterogeneity. J Immunol. (2008) 180:5707–19. doi: 10.4049/jimmunol.180.8.5707
95. Bonito V, De Kort BJ, Bouten CVC, Smits AIPM. Cyclic strain affects macrophage cytokine secretion and extracellular matrix turnover in electrospun scaffolds. Tissue Eng Part A. (2019) 25:1310–25. doi: 10.1089/ten.tea.2018.0306
96. Simões FC, Cahill TJ, Kenyon A, Gavriouchkina D, Vieira JM, Sun X, et al. Macrophages directly contribute collagen to scar formation during zebrafish heart regeneration and mouse heart repair. Nat Commun. (2020) 11:1–17. doi: 10.1038/s41467-019-14263-2
97. O’Rourke SA, Dunne A, Monaghan MG. The role of macrophages in the infarcted myocardium: orchestrators of ECM remodeling. Front Cardiovasc Med. (2019) 6:101. doi: 10.3389/fcvm.2019.00101
98. Alvarez-Argote S, O’meara CC. The evolving roles of cardiac macrophages in homeostasis, regeneration, and repair. Int J Mol Sci. (2021) 22:7923. doi: 10.3390/ijms22157923
99. Hoeffel G, Ginhoux F. Fetal monocytes and the origins of tissue-resident macrophages. Cell Immunol. (2018) 330:5–15. doi: 10.1016/j.cellimm.2018.01.001
100. Minutti CM, Knipper JA, Allen JE, Zaiss DMW. Tissue-specific contribution of macrophages to wound healing. Semin Cell Dev Biol. (2017) 61:3–11. doi: 10.1016/j.semcdb.2016.08.006
101. Ginhoux F, Guilliams M. Tissue-resident macrophage ontogeny and homeostasis. Immunity. (2016) 44:439–49. doi: 10.1016/J.IMMUNI.2016.02.024
102. Davies LC, Jenkins SJ, Allen JE, Taylor PR. Tissue-resident macrophages. Nat Immunol. (2013) 14:986–95. doi: 10.1038/ni.2705
103. Kim AJ, Xu N, Yutzey KE. Macrophage lineages in heart valve development and disease. Cardiovasc Res. (2021) 117:663–73. doi: 10.1093/CVR/CVAA062
104. Visconti RP, Ebihara Y, LaRue AC, Fleming PA, McQuinn TC, Masuya M, et al. An in vivo analysis of hematopoietic stem cell potential: hematopoietic origin of cardiac valve interstitial cells. Circ Res. (2006) 98:690–6. doi: 10.1161/01.RES.0000207384.81818.D4
105. Hulin A, Anstine LJ, Kim AJ, Potter SJ, DeFalco T, Lincoln J, et al. Macrophage transitions in heart valve development and myxomatous valve disease. Arterioscler Thromb Vasc Biol. (2018) 38:636–44. doi: 10.1161/ATVBAHA.117.310667
106. Hulin A, Hortells L, Gomez-Stallons MV, O’Donnell A, Chetal K, Adam M, et al. Maturation of heart valve cell populations during postnatal remodeling. Development. (2019) 146:dev173047. doi: 10.1242/DEV.173047
107. Anstine LJ, Horne TE, Horwitz EM, Lincoln J. Contribution of extra-cardiac cells in murine heart valves is age-dependent. J Am Heart Assoc. (2017) 6:e007097. doi: 10.1161/JAHA.117.007097
108. Shigeta A, Huang V, Zuo J, Besada R, Nakashima Y, Lu Y, et al. Endocardially derived macrophages are essential for valvular remodeling. Dev Cell. (2019) 48:617–30.e3. doi: 10.1016/J.DEVCEL.2019.01.021
109. Wang X, Li Y, Feng Y, Cheng H, Li D. The role of macrophages in osseointegration of dental implants: an experimental study in vivo. J Biomed Mater Res Part A. (2020) 108:2206–16. doi: 10.1002/jbm.a.36978
110. Smits AIPM, Ballotta V, Driessen-Mol A, Bouten CVC, Baaijens FPT. Shear flow affects selective monocyte recruitment into MCP-1-loaded scaffolds. J Cell Mol Med. (2014) 18:2176–88. doi: 10.1111/jcmm.12330
111. Ballotta V, Smits AIPM, Driessen-Mol A, Bouten CVC, Baaijens FPT. Synergistic protein secretion by mesenchymal stromal cells seeded in 3D scaffolds and circulating leukocytes in physiological flow. Biomaterials. (2014) 35:9100–13. doi: 10.1016/j.biomaterials.2014.07.042
112. Bonito V, Smits AIPM, Goor OJGM, Ippel BD, Driessen-Mol A, Münker TJAG, et al. Modulation of macrophage phenotype and protein secretion via heparin-IL-4 functionalized supramolecular elastomers. Acta Biomater. (2018) 71:247–60. doi: 10.1016/j.actbio.2018.02.032
113. Battiston KG, Labow RS, Simmons CA, Santerre JP. Immunomodulatory polymeric scaffold enhances extracellular matrix production in cell co-cultures under dynamic mechanical stimulation. Acta Biomater. (2015) 24:74–86. doi: 10.1016/j.actbio.2015.05.038
114. Zhang X, Simmons CA, Paul Santerre J. Paracrine signalling from monocytes enables desirable extracellular matrix accumulation and temporally appropriate phenotype of vascular smooth muscle cell-like cells derived from adipose stromal cells. Acta Biomater. (2020) 103:129–41. doi: 10.1016/j.actbio.2019.12.006
115. Shrestha S, McFadden MJ, Gramolini AO, Santerre JP. Proteome analysis of secretions from human monocyte-derived macrophages post-exposure to biomaterials and the effect of secretions on cardiac fibroblast fibrotic character. Acta Biomater. (2020) 111:80–90. doi: 10.1016/j.actbio.2020.04.042
116. Bosshart H, Heinzelmann M. THP-1 cells as a model for human monocytes. Ann Transl Med. (2016) 4:4–7. doi: 10.21037/atm.2016.08.53
117. Chanput W, Mes JJ, Wichers HJ. THP-1 cell line: an in vitro cell model for immune modulation approach. Int Immunopharmacol. (2014) 23:37–45. doi: 10.1016/j.intimp.2014.08.002
118. Wissing TB, Van Haaften EE, Koch SE, Ippel BD, Kurniawan NA, Bouten CVC, et al. Hemodynamic loads distinctively impact the secretory profile of biomaterial-activated macrophages-implications for in situ vascular tissue engineering. Biomater Sci. (2020) 8:132–47. doi: 10.1039/c9bm01005j
119. Wissing TB, Bonito V, van Haaften EE, van Doeselaar M, Brugmans MM, Janssen HM, et al. Macrophage-driven biomaterial degradation depends on scaffold microarchitecture. Front Bioeng Biotechnol. (2019) 7:87. doi: 10.3389/fbioe.2019.00087
120. Shiratori H, Feinweber C, Luckhardt S, Linke B, Resch E, Geisslinger G, et al. THP-1 and human peripheral blood mononuclear cell-derived macrophages differ in their capacity to polarize in vitro. Mol Immunol. (2017) 88:58–68. doi: 10.1016/j.molimm.2017.05.027
121. Buchrieser J, James W, Moore MD. Human induced pluripotent stem cell-derived macrophages share ontogeny with MYB-independent tissue-resident macrophages. Stem Cell Rep. (2017) 8:334–45. doi: 10.1016/j.stemcr.2016.12.020
122. Takata K, Kozaki T, Lee CZW, Thion MS, Otsuka M, Lim S, et al. Induced-pluripotent-stem-cell-derived primitive macrophages provide a platform for modeling tissue-resident macrophage differentiation and function. Immunity. (2017) 47:183–98.e6. doi: 10.1016/j.immuni.2017.06.017
123. Lee CZW, Kozaki T, Ginhoux F. Studying tissue macrophages in vitro: are iPSC-derived cells the answer? Nat Rev Immunol. (2018) 18:716–25. doi: 10.1038/s41577-018-0054-y
124. Tasnim F, Xing J, Huang X, Mo S, Wei X, Tan MH, et al. Generation of mature kupffer cells from human induced pluripotent stem cells. Biomaterials. (2019) 192:377–91. doi: 10.1016/j.biomaterials.2018.11.016
125. Xu R, Li X, Boreland AJ, Posyton A, Kwan K, Hart RP, et al. Human iPSC-derived mature microglia retain their identity and functionally integrate in the chimeric mouse brain. Nat Commun. (2020) 11:1–16. doi: 10.1038/s41467-020-15411-9
126. Mucci A, Lopez-Rodriguez E, Hetzel M, Liu S, Suzuki T, Happle C, et al. iPSC-derived macrophages effectively treat pulmonary alveolar proteinosis in Csf2rb-deficient mice. Stem Cell Rep. (2018) 11:696–710. doi: 10.1016/j.stemcr.2018.07.006
127. Eckes B, Nischt R, Krieg T. Cell-matrix interactions in dermal repair and scarring. Fibrogenes Tissue Repair. (2010) 3:4. doi: 10.1186/1755-1536-3-4
128. Burridge K, Fath K, Kelly T, Nuckolls G, Turner C. Focal adhesions: transmembrane junctions between the extracellular matrix and the cytoskeleton. Annu Rev Cell Biol. (1988) 4:487–525. doi: 10.1146/annurev.cb.04.110188.002415
129. Yamada KM, Gailit J, Clark RAF. Integrins in wound repair. In: RAF Clark editor. The Molecular and Cellular Biology of Wound Repair. Boston, MA: Springer (1988). doi: 10.1007/978-1-4899-0185-9_9
130. Chester D, Brown AC. The role of biophysical properties of provisional matrix proteins in wound repair. Matrix Biol. (2017) 60–1:124–40. doi: 10.1016/J.MATBIO.2016.08.004
131. Simon T, Bromberg JS. Regulation of the immune system by laminins. Trends Immunol. (2017) 38:858–71. doi: 10.1016/j.it.2017.06.002
132. Davis GE, Bayless KJ, Davis MJ, Meininger GA. Regulation of tissue injury responses by the exposure of matricryptic sites within extracellular matrix molecules. Am J Pathol. (2000) 156:1489–98. doi: 10.1016/S0002-9440(10)65020-1
133. McQuitty CE, Williams R, Chokshi S, Urbani L. Immunomodulatory role of the extracellular matrix within the liver disease microenvironment. Front Immunol. (2020) 11:574276. doi: 10.3389/fimmu.2020.574276
134. Chen GY, Nuñez G. Sterile inflammation: sensing and reacting to damage. Nat Rev Immunol. (2010) 10:826–37. doi: 10.1038/nri2873
135. Adair-Kirk TL, Senior RM. Fragments of extracellular matrix as mediators of inflammation. Int J Biochem Cell Biol. (2008) 40:1101–10. doi: 10.1016/j.biocel.2007.12.005
136. Frevert CW, Felgenhauer J, Wygrecka M, Nastase MV, Schaefer L. Danger-associated molecular patterns derived from the extracellular matrix provide temporal control of innate immunity. J Histochem Cytochem. (2018) 66:213–27. doi: 10.1369/0022155417740880
137. Schaefer L, Babelova A, Kiss E, Hausser HJ, Baliova M, Krzyzankova M, et al. The matrix component biglycan is proinflammatory and signals through toll-like receptors 4 and 2 in macrophages. J Clin Invest. (2005) 115:2223–33. doi: 10.1172/JCI23755
138. Merline R, Moreth K, Beckmann J, Nastase MV, Zeng-Brouwers J, Tralhão JG, et al. Signaling by the matrix proteoglycan decorin controls inflammation and cancer through PDCD4 and microRNA-21. Sci Signal. (2011) 4:ra75. doi: 10.1126/scisignal.2001868
139. Hsieh LTH, Frey H, Nastase MV, Tredup C, Hoffmann A, Poluzzi C, et al. Bimodal role of NADPH oxidases in the regulation of biglycan-triggered IL-1β synthesis. Matrix Biol. (2016) 49:61–81. doi: 10.1016/j.matbio.2015.12.005
140. Okada M, Imoto K, Sugiyama A, Yasuda J, Yamawaki H. New insights into the role of basement membrane-derived matricryptins in the heart. Biol Pharm Bull. (2017) 40:2050–60. doi: 10.1248/bpb.b17-00308
141. Foguer K, de Braga MS, Peron JPS, Bortoluci KR, Bellini MH. Endostatin gene therapy inhibits intratumoral macrophage M2 polarization. Biomed Pharmacother. (2016) 79:102–11. doi: 10.1016/j.biopha.2016.01.035
142. Schmidt DR, Kao WJ. The interrelated role of fibronectin and interleukin-1 in biomaterial-modulated macrophage function. Biomaterials. (2007) 28:371–82. doi: 10.1016/j.biomaterials.2006.08.041
143. Gelse K, Pöschl E, Aigner T. Collagens – Structure, function, and biosynthesis. Adv Drug Deliv Rev. (2003) 55:1531–46. doi: 10.1016/j.addr.2003.08.002
144. Madsen DH, Leonard D, Masedunskas A, Moyer A, Jürgensen HJ, Peters DE, et al. M2-like macrophages are responsible for collagen degradation through a mannose receptor-mediated pathway. J Cell Biol. (2013) 202:951–66. doi: 10.1083/jcb.201301081
145. Boraschi-Diaz I, Wang J, Mort JS, Komarova SV. Collagen type i as a ligand for receptor-mediated signaling. Front Phys. (2017) 5:12. doi: 10.3389/fphy.2017.00012
146. Rowley AT, Meli VS, Wu-Woods NJ, Chen EY, Liu WF, Wang S-W. Effects of surface-bound collagen-mimetic peptides on macrophage uptake and immunomodulation. Front Bioeng Biotechnol. (2020) 8:747. doi: 10.3389/fbioe.2020.00747
147. Zaveri TD, Lewis JS, Dolgova NV, Clare-Salzler MJ, Keselowsky BG. Integrin-directed modulation of macrophage responses to biomaterials. Biomaterials. (2014) 35:3504–15. doi: 10.1016/j.biomaterials.2014.01.007
148. Meyaard L. LAIR and collagens in immune regulation. Immunol Lett. (2010) 128:26–8. doi: 10.1016/j.imlet.2009.09.014
149. Rowley AT, Nagalla RR, Wang S, Liu WF. Extracellular matrix-based strategies for immunomodulatory biomaterials engineering. Adv Healthc Mater. (2019) 8:1801578. doi: 10.1002/adhm.201801578
150. Pakshir P, Alizadehgiashi M, Wong B, Coelho NM, Chen X, Gong Z, et al. Dynamic fibroblast contractions attract remote macrophages in fibrillar collagen matrix. Nat Commun. (2019) 10:1–17. doi: 10.1038/s41467-019-09709-6
151. Lenselink EA. Role of fibronectin in normal wound healing. Int Wound J. (2015) 12:313–6. doi: 10.1111/iwj.12109
152. Barker TH, Engler AJ. The provisional matrix: setting the stage for tissue repair outcomes. Matrix Biol. (2017) 60–1:1–4. doi: 10.1016/j.matbio.2017.04.003
153. Grinnell F. Fibronectin and wound healing. J Cell Biochem. (1984) 26:107–16. doi: 10.1002/jcb.240260206
154. Majumdar S, Kraft ML. Exploring the maturation of a monocytic cell line using self-organizing maps of single-cell Raman spectra. Biointerphases. (2020) 15:041010. doi: 10.1116/6.0000363
155. Fei D, Meng X, Yu W, Yang S, Song N, Cao Y, et al. Fibronectin (FN) cooperated with TLR2/TLR4 receptor to promote innate immune responses of macrophages via binding to integrin β1. Virulence. (2018) 9:1588–600. doi: 10.1080/21505594.2018.1528841
156. MacIel J, Oliveira MI, Gonalves RM, Barbosa MA. The effect of adsorbed fibronectin and osteopontin on macrophage adhesion and morphology on hydrophilic and hydrophobic model surfaces. Acta Biomater. (2012) 8:3669–77. doi: 10.1016/j.actbio.2012.06.010
157. Digiacomo G, Tusa I, Bacci M, Cipolleschi MG, Dello Sbarba P, Rovida E. Fibronectin induces macrophage migration through a SFK-FAK/CSF-1R pathway. Cell Adhes Migr. (2017) 11:327–37. doi: 10.1080/19336918.2016.1221566
158. Seiffert D, Smith JW. The cell adhesion domain in plasma vitronectin is cryptic. J Biol Chem. (1997) 272:13705–10. doi: 10.1074/jbc.272.21.13705
159. Ugarova TP, Zamarron C, Plow EF, Veklich Y, Weisel JW, Bowditch RD, et al. Conformational transitions in the cell binding domain of fibronectin. Biochemistry. (1995) 34:4457–66. doi: 10.1021/bi00013a039
160. Ricard-Blum S, Ballut L. Matricryptins derived from collagens and proteoglycans. Front Biosci. (2011) 16:674–97. doi: 10.2741/3712
161. Davis GE. Affinity of integrins for damaged extracellular matrix: αvβ3 binds to denatured collagen type I through RGD sites. Biochem Biophys Res Commun. (1992) 182:1025–31. doi: 10.1016/0006-291X(92)91834-D
162. Kang H, Yang B, Zhang K, Pan Q, Yuan W, Li G, et al. Immunoregulation of macrophages by dynamic ligand presentation via ligand–cation coordination. Nat Commun. (2019) 10:1696. doi: 10.1038/s41467-019-09733-6
163. Kao WJ, Lee D. In vivo modulation of host response and macrophage behavior by polymer networks grafted with fibronectin-derived biomimetic oligopeptides: the role of RGD and PHSRN domains. Biomaterials. (2001) 22:2901–9. doi: 10.1016/S0142-9612(01)00037-0
164. Bisaccia F, Morelli MAC, de Biasi M, Traniello S, Spisani S, Tamburro AM. Migration of monocytes in the presence of elastolytic fragments of elastin and in synthetic derivates structure-activity relationships. Int J Pept Protein Res. (1994) 44:332–41. doi: 10.1111/j.1399-3011.1994.tb01017.x
165. Senior RM, Griffin GL, Mecham RP, Wrenn DS, Prasad KU, Urry DW. Val-Gly-Val-Ala-Pro-Gly, a repeating peptide in elastin, is chemotactic for fibroblasts and monocytes. J Cell Biol. (1984) 99:870–4. doi: 10.1083/jcb.99.3.870
166. Hunninghake GW, Davidson JM, Rennard S, Szapiel S, Gadek JE, Crystal RG. Elastin fragments attract macrophage precursors to diseased sites in pulmonary emphysema. Science. (1981) 212:925–7. doi: 10.1126/SCIENCE.7233186
167. Maquart FX, Bellon G, Pasco S, Monboisse JC. Matrikines in the regulation of extracellular matrix degradation. Biochimie. (2005) 87:353–60. doi: 10.1016/j.biochi.2004.10.006
168. Wells SM, Sellaro T, Sacks MS. Cyclic loading response of bioprosthetic heart valves?: effects of fixation stress state on the collagen fiber architecture. Biomaterials. (2005) 26:2611–9. doi: 10.1016/j.biomaterials.2004.06.046
169. Hinek A, Boyle J, Rabinovitch M. Vascular smooth muscle cell detachment from elastin and migration through elastic laminae is promoted by chondroitin sulfate-induced “shedding” of the 67-kDa cell surface elastin binding protein. Exp Cell Res. (1992) 203:344–53. doi: 10.1016/0014-4827(92)90008-V
170. Sorokin L. The impact of the extracellular matrix on inflammation. Nat Rev Immunol. (2010) 10:712–23. doi: 10.1038/nri2852
171. Weathington NM, Van Houwelingen AH, Noerager BD, Jackson PL, Kraneveld AD, Galin FS, et al. A novel peptide CXCR ligand derived from extracellular matrix degradation during airway inflammation. Nat Med. (2006) 12:317–23. doi: 10.1038/nm1361
172. Pickart L, Vasquez-Soltero JM, Margolina A. GHK peptide as a natural modulator of multiple cellular pathways in skin regeneration. Biomed Res Int. (2015) 2015:648108. doi: 10.1155/2015/648108
173. Flanagan TC, Sachweh JS, Frese J, Schnöring H, Gronloh N, Koch S, et al. In vivo remodeling and structural characterization of fibrin-based tissue-engineered heart valves in the adult sheep model. Tissue Eng Part A. (2009) 15:2965–76. doi: 10.1089/ten.tea.2009.0018
174. Gottlieb D, Kunal T, Emani S, Aikawa E, Brown DW, Powell AJ, et al. In vivo monitoring of function of autologous engineered pulmonary valve. J Thorac Cardiovasc Surg. (2010) 139:723–31. doi: 10.1016/j.jtcvs.2009.11.006
175. Schmidt D, Dijkman PE, Driessen-Mol A, Stenger R, Mariani C, Puolakka A, et al. Minimally-invasive implantation of living tissue engineered heart valves. J Am Coll Cardiol. (2010) 56:510–20. doi: 10.1016/j.jacc.2010.04.024
176. Sanders B, Loerakker S, Fioretta ES, Bax DJp, Driessen-Mol A, Hoerstrup SP, et al. Improved geometry of decellularized tissue engineered heart valves to prevent leaflet retraction. Ann Biomed Eng. (2016) 44:1061–71. doi: 10.1007/s10439-015-1386-4
177. Motta SE, Fioretta ES, Lintas V, Dijkman PE, Hilbe M, Frese L, et al. Geometry influences inflammatory host cell response and remodeling in tissue-engineered heart valves in-vivo. Sci Rep. (2020) 10:1–14. doi: 10.1038/s41598-020-76322-9
178. Moore LB, Kyriakides TR. Molecular characterization of macrophage-biomaterial interactions. Adv Exp Med Biol. (2015) 865:109–22. doi: 10.1007/978-3-319-18603-0_7
179. Choudhury D, Yee M, Sheng ZLJ, Amirul A, Naing MW. Decellularization systems and devices: state-of-the-art. Acta Biomater. (2020) 115:51–9. doi: 10.1016/j.actbio.2020.07.060
180. Behmer Hansen RA, Wang X, Kaw G, Pierre V, Senyo SE. Accounting for material changes in decellularized tissue with underutilized methodologies. Biomed Res Int. (2021) 2021:6696295. doi: 10.1155/2021/6696295
181. Aamodt JM, Grainger DW. Extracellular matrix-based biomaterial scaffolds and the host response. Biomaterials. (2016) 86:68–82. doi: 10.1016/j.biomaterials.2016.02.003
182. Crapo PM, Gilbert TW, Badylak SF. An overview of tissue and whole organ decellularization processes. Biomaterials. (2011) 32:3233–43. doi: 10.1016/j.biomaterials.2011.01.057
183. Dziki JL, Wang DS, Pineda C, Sicari BM, Rausch T, Badylak SF. Solubilized extracellular matrix bioscaffolds derived from diverse source tissues differentially influence macrophage phenotype. J Biomed Mater Res Part A. (2017) 105:138–47. doi: 10.1002/jbm.a.35894
184. Londono R, Dziki JL, Haljasmaa E, Turner NJ, Leifer CA, Badylak SF. The effect of cell debris within biologic scaffolds upon the macrophage response. J Biomed Mater Res Part A. (2017) 105:2109–18. doi: 10.1002/jbm.a.36055
185. Petrosyan A, Da Sacco S, Tripuraneni N, Kreuser U, Lavarreda-Pearce M, Tamburrini R, et al. A step towards clinical application of acellular matrix: a clue from macrophage polarization. Matrix Biol. (2017) 57–58:334–46. doi: 10.1016/j.matbio.2016.08.009
186. Keane TJ, Londono R, Turner NJ, Badylak SF. Consequences of ineffective decellularization of biologic scaffolds on the host response. Biomaterials. (2012) 33:1771–81. doi: 10.1016/j.biomaterials.2011.10.054
187. Ground M, Waqanivavalagi S, Walker R, Milsom P, Cornish J. Models of immunogenicity in preclinical assessment of tissue engineered heart valves. Acta Biomater. (2021) 133:102–13. doi: 10.1016/j.actbio.2021.05.049
188. Bm S, Jl D, Bf S, Cj M, Cl D, Sf B. The promotion of a constructive macrophage phenotype by solubilized extracellular matrix. Biomaterials. (2014) 35:8605–12. doi: 10.1016/J.BIOMATERIALS.2014.06.060
189. Dohmen PM, Da Costa F, Lopes SV, Yoshi S, Da Souza FP, Vilani R, et al. Results of a decellularized porcine heart valve implanted into the juvenile sheep model. Heart Surg Forum. (2005) 8:72–6. doi: 10.1532/HSF98.20041140
190. Iwai S, Torikai K, Coppin CM, Sawa Y. Minimally immunogenic decellularized porcine valve provides in situ recellularization as a stentless bioprosthetic valve. J Artif Organs. (2007) 10:29–35. doi: 10.1007/s10047-006-0360-1
191. Leyh RG, Wilhelmi M, Walles T, Kallenbach K, Rebe P, Oberbeck A, et al. A cellularized porcine heart valve scaffolds for heart valve tissue engineering and the risk of cross-species transmission of porcine endogenous retrovirus. J Thorac Cardiovasc Surg. (2003) 126:1000–4. doi: 10.1016/S0022-5223(03)00353-2
192. Goecke T, Theodoridis K, Tudorache I, Ciubotaru A, Cebotari S, Ramm R, et al. In vivo performance of freeze-dried decellularized pulmonary heart valve allo- and xenografts orthotopically implanted into juvenile sheep. Acta Biomater. (2018) 68:41–52. doi: 10.1016/j.actbio.2017.11.041
193. D’Amore A, Luketich SK, Raffa GM, Olia S, Menallo G, Mazzola A, et al. Heart valve scaffold fabrication: bioinspired control of macro-scale morphology, mechanics and micro-structure. Biomaterials. (2018) 150:25–37. doi: 10.1016/j.biomaterials.2017.10.011
194. Tallawi M, Rosellini E, Barbani N, Cascone MG, Rai R, Saint-Pierre G, et al. Strategies for the chemical and biological functionalization of scaffolds for cardiac tissue engineering: a review. J R Soc Interface. (2015) 12:20150254. doi: 10.1098/rsif.2015.0254
195. Bonfield TL, Colton E, Marchant RE, Anderson JM. Cytokine and growth factor production by monocytes/macrophages on protein preadsorbed polymers. J Biomed Mater Res. (1992) 26:837–50. doi: 10.1002/jbm.820260702
196. Rossi F, Van Griensven M. Polymer functionalization as a powerful tool to improve scaffold performances. Tissue Eng Part A. (2014) 20:2043–51. doi: 10.1089/ten.tea.2013.0367
197. Gwanmesia P, Ziegler H, Eurich R, Barth M, Kamiya H, Karck M, et al. Opposite effects of transforming growth factor-β1 and vascular endothelial growth factor on the degeneration of aortic valvular interstitial cell are modified by the extracellular matrix protein fibronectin: implications for heart valve engineering. Tissue Eng Part A. (2010) 16:3737–46. doi: 10.1089/ten.tea.2010.0304
198. Barthes J, Lagarrigue P, Riabov V, Lutzweiler G, Kirsch J, Muller C, et al. Biofunctionalization of 3D-printed silicone implants with immunomodulatory hydrogels for controlling the innate immune response: an in vivo model of tracheal defect repair. Biomaterials. (2021) 268:120549. doi: 10.1016/j.biomaterials.2020.120549
199. Fioretta ES, Fledderus JO, Burakowska-Meise EA, Baaijens FPT, Verhaar MC, Bouten CVC. Polymer-based Scaffold designs for in situ vascular tissue engineering: controlling recruitment and differentiation behavior of endothelial colony forming cells. Macromol Biosci. (2012) 12:577–90. doi: 10.1002/mabi.201100315
200. Ren X, Feng Y, Guo J, Wang H, Li Q, Yang J, et al. Surface modification and endothelialization of biomaterials as potential scaffolds for vascular tissue engineering applications. Chem Soc Rev. (2015) 44:5680–742. doi: 10.1039/c4cs00483c
Keywords: collagen, fibronectin, inflammation, regenerative medicine, scaffold functionalization, immune response, extracellular matrix (ECM), macrophages
Citation: Poulis N, Martin M, Hoerstrup SP, Emmert MY and Fioretta ES (2022) Macrophage-extracellular matrix interactions: Perspectives for tissue engineered heart valve remodeling. Front. Cardiovasc. Med. 9:952178. doi: 10.3389/fcvm.2022.952178
Received: 24 May 2022; Accepted: 15 August 2022;
Published: 13 September 2022.
Edited by:
Laura Iop, University of Padua, ItalyReviewed by:
Jatin Patel, Queensland University of Technology, AustraliaJohn Mayer, Boston Childrens Hospital and Harvard Medical School, United States
Copyright © 2022 Poulis, Martin, Hoerstrup, Emmert and Fioretta. This is an open-access article distributed under the terms of the Creative Commons Attribution License (CC BY). The use, distribution or reproduction in other forums is permitted, provided the original author(s) and the copyright owner(s) are credited and that the original publication in this journal is cited, in accordance with accepted academic practice. No use, distribution or reproduction is permitted which does not comply with these terms.
*Correspondence: Maximilian Y. Emmert, maximilian.emmert@irem.uzh.ch, emmert@dhzb.de; Emanuela S. Fioretta, emanuela.fioretta@irem.uzh.ch
†These authors have contributed equally to this work and share first authorship
‡These authors have contributed equally to this work and share last authorship