Tungsten Promoted Ni/Al2O3 as a Noble-Metal-Free Catalyst for the Conversion of 5-Hydroxymethylfurfural to 1-Hydroxy-2,5-Hexanedione
- 1College of Food and Drug, Luoyang Normal University, Luoyang, China
- 2Henan Key Laboratory of Function-Oriented Porous Material, College of Chemistry and Chemical Engineering, Luoyang Normal University, Luoyang, China
The conversion of 5-hydroxymethylfurfural (HMF) to 1-hydroxy-2,5-hexanedione (HHD) represented a typical route for high-value utilization of biomass. However, this reaction was often catalyzed by the noble metal catalyst. In this manuscript, W promoted Ni/Al2O3 was prepared as a noble-metal-free catalyst for this transformation. The catalysts were characterized by XRD, XPS, NH3-TPD, TEM, and EDS-mapping to study the influence of the introduction of W. There was an interaction between Ni and W, and strong acid sites were introduced by the addition of W. The W promoted Ni/Al2O3 showed good selectivity to HHD when used as a catalyst for the hydrogenation of HMF in water. The influences of the content of W, temperature, H2 pressure, reaction time, and acetic acid (AcOH) were studied. NiWOx/Al2O3-0.5 (mole ratio of W:Ni = 0.5) was found to be the most suitable catalyst. The high selectivity to HHD was ascribed to the acid sites introduced by W. This was proved by the fact that the selectivity to HHD was increased a lot when AcOH was added just using Ni/Al2O3 as catalysts. 59% yield of HHD was achieved on NiWOx/Al2O3-0.5 at 393 K, 4 MPa H2 reacting for 6 h, which was comparable to the noble metal catalyst, showing the potential application in the production of HHD from HMF.
Introduction
The production of chemicals from a renewable resource is one of the essential tasks for sustainable chemistry (Corma et al., 2007; Besson et al., 2014; Mika et al., 2017; Fang et al., 2020; Xu et al., 2020). As the 5-hydroxymethylfurfural (HMF) could be obtained easily by the dehydration of hexoses (Yu and Tsang, 2017; Fan et al., 2019; Kang et al., 2019; Chang et al., 2021; Das and Mohanty, 2021; Guo et al., 2021; Tempelman et al., 2021), a class of compounds abundant in nature, the transformation of HMF is one of the hot topics for sustainable chemistry (Averochkin et al., 2021; Bielski and Grynkiewicz, 2021; Fang and Riisager, 2021). Many studies had focused on the conversion of HMF to various products with potential or practice applications. It was reported that fuels (Esteves et al., 2020) and their additives (Nagpure et al., 2020), polymer monomers (Duan et al., 2017a; Elsayed et al., 2020; Wang et al., 2020; Fulignati et al., 2021) and other chemicals (Ohyama et al., 2017; Ramos et al., 2017; Wozniak et al., 2019; Zhang et al., 2019) with the high added value could be produced using HMF as feedstock through catalytic hydrogenation (Ohyama et al., 2016; Ren et al., 2016; Yang et al., 2019a; Long et al., 2019; Han et al., 2020; Wiesfeld et al., 2020; Gao et al., 2021), oxidation (Neatu et al., 2016; Martínez-Vargas et al., 2017; Deshan et al., 2020), etherification (Che et al., 2015) and other catalytic procedures (Karve et al., 2020; Zhang et al., 2021).
1-hydroxy-2,5-hexanedione (HHD) was one of the high value-added compounds obtained from HMF through catalytic hydrogenation (Schiavo et al., 1991; Gupta et al., 2015; Zhu et al., 2019; Yang et al., 2020). The HHD could be used for the preparation of polyols, nitrogen and oxygen heterocycles. Recently, it was reported that the HHD could convert to 2-hydroxy-3-methyl-2-cyclopenten-1-one (MCP) through an intramolecular aldol condensation procedure at mild conditions (Duan et al., 2017b; Wozniak et al., 2018). The MCP was a commercialized edible essence produced from petrochemical feedstock by multistep reactions with low yield and severe pollution. The HHD was a potential feedstock candidate for the improvement in the production of MCP.
The transformation of HMF to HHD had been reported by several groups. The reaction was usually conducted in water under H2 pressure. The formic acid could also be used as the hydrogen source when a homogeneous catalyst was used (Xu et al., 2017). Acid additives such as HCl, Amberlyst-15 or H3PO4 was usually necessary for this conversion (Liu et al., 2014). To avoid acid additives, the introduction of acid sites in the catalyst was a good choice. For example, the reaction could conduct without the addition of acid additives when acid support MIL-101 (Yang et al., 2019b), zeolite (Ramos et al., 2019) or Nb2O5 (Duan et al., 2017b) was used for the preparation of Pd catalyst. The supported Pd was found to be an effective heterogeneous catalyst for this transformation while the Ir complexes were a good homogeneous catalyst candidate (Xu et al., 2017). The Ru (Gupta et al., 2015) complex and supported Au (Ohyama et al., 2014) could also catalyse this reaction. The employment of noble-metal was a barrier for the further application of the conversion of HMF to HHD. To reduce the use of noble-metal, high-performance catalyst with low noble-metal load, high dispersion and activity was designed and applied for this transformation.
The application of noble-metal-free catalyst for the conversion of HMF to HHD without acid additives was a better choice for the improvement. However, there was only one report that HMF in water (∼0.3 wt%) could be converted to HHD by Ni2P nanoparticles up to now (Fujita et al., 2020). In this manuscript, the tungsten promoted Ni/Al2O3 was simply prepared and used as a noble-metal-free catalyst for the transformation of HMF to HHD. The catalyst showed high activity and stability in the reaction. The introduction of tungsten in the catalyst improved the activity and selectivity to HHD greatly.
Results and Discussion
Characterization
The X-ray diffraction (XRD) patterns of NiWOx/Al2O3 with different content of W was displayed in Figure 1. Figure 1A were the results of samples after calcined. Except for the diffraction peaks for γ-Al2O3, Ni/Al2O3 had additional two peaks at 37.3o and 43.4o. This should be ascribed to (111) and (200) diffraction peaks of NiO (PDF#47-1049). The two peaks decreased as the content of W increased for NiWOx/Al2O3 and almost disappeared for NiWOx/Al2O3-0.7 and NiWOx/Al2O3-0.9. The WOx/Al2O3 showed (001) (020) (200) (111) (021) (201), and (220) peaks of WO3 without NiO peaks (Supplementary Figure S1). W species mainly existed as WO3 after the calcination. However, no diffraction peaks ascribed to WOx could be found for NiWOx/Al2O3 showed that the W had a reasonable degree of dispersion on the support. After the Ni/Al2O3 was reduced in H2, the diffraction peaks ascribed to NiO disappeared (Figure 1B). Two new peaks corresponding to Ni (111) and (200) were immersed at 44.5o and 51.9o (PDF#04-0850). This showed that most of the NiO could be reduced to Ni by H2 reduction, which was consistent with the results of H2-Temperature programmed reduction (H2-TPR) (Supplementary Figure S2 and Table S1). After the introduction of W, NiWOx/Al2O3 still had the diffraction peaks of Ni. The diffraction peaks moved to a smaller angle with increased W content. This should be caused by the W atoms entering the lattice of Ni.
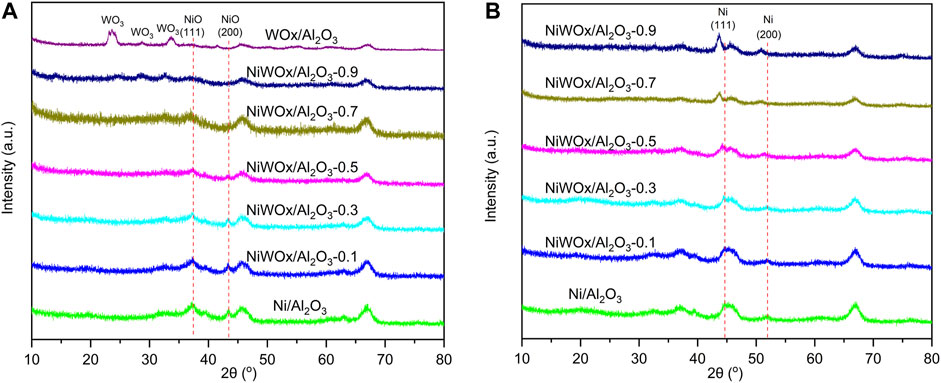
FIGURE 1. The XRD patterns of NiWOx/Al2O3 with different content of W before (A) and after (B) H2 reduction.
The surface chemical state was characterized by X-ray photoelectron spectroscopy (XPS) and the results were shown in Figure 2 and Supplementary Figure S3. After deconvolution operation, the Ni/Al2O3 showed two peaks centered at 856.7 and 855.3 eV, which should be ascribed to NiAl2O4 and NiO, respectively (Figure 2A) (Yang et al., 2016). No peaks (853.0 eV) ascribed to metallic Ni could be found in the XPS spectra. This should be caused by the oxidation of surface Ni when exposed to air. The binding energy shifted to higher energy when W was introduced. The binding energy peaks of NiAl2O4 and NiO were 857.4 and 855.8 eV, respectively for NiWOx/Al2O3-0.5. This decrease indicated that the W had interaction with Ni. This interaction between Ni and W should weaken that between Ni and Al. Consequently, the relative content of NiAl2O4 decreased after the introduction of W revealed by the result of XPS (Figure 2A). The interaction between Ni and W was further proved by the XPS spectra of W (Figure 2B). The WOx/Al2O3 had peaks at 37.4 and 35.4 eV corresponding to the binding energy of WO3 of 4f5/2 and 4f7/2 (Cao et al., 2014). This value decreased to 37.3 and 35.3 for NiWOx/Al2O3-0.5. The interaction between Ni and W was also consistent with the results of H2-TPR that the reduction temperature peak was changed after the introduction of W (Supplementary Figure S2; Table S1).
The acidic properties of Ni/Al2O3, NiWOx/Al2O3-0.5 and WOx/Al2O3 were investigated by the temperature-programmed desorption of ammonia (NH3-TPD), and the results were shown in Figure 3 and Supplementary Figure S4. The Ni/Al2O3 showed a sharp peak at 570 K which was ascribed to the weak to medium acid site of Al2O3. The NH3-TPD of NiWOx/Al2O3-0.5 showed three wide peaks centered at 442, 544, and 700 K corresponding to the weak acid, medium acid, and strong acid sites respectively. The NiWOx/Al2O3-0.5 posed a relatively strong acid site except for the weak to medium acid site compared to Ni/Al2O3. This desorption curve was similar to WOx/Al2O3 (Supplementary Figure S4). Hence, the strong acid site was produced due to the introduction of W in NiWOx/Al2O3-0.5.
The morphology of Ni/Al2O3 and NiWOx/Al2O3-0.5 was characterized by transmission electron microscopy (TEM). The TEM images (Figure 4) showed that the Ni was distributed on the surface of the support in granular or rod form for both Ni/Al2O3, NiWOx/Al2O3-0.5 in nanoscale. To get a clearer distribution of elements, the energy-dispersive spectrometer (EDS) mapping was taken for both catalysts. The results were shown in Figures 4C,D, Supplementary Figures S5–8 and Supplementary Table S2. The Supplementary Table S2 summarized the percentage of each element in Ni/Al2O3 and NiWOx/Al2O3-0.5. The content of Ni, W was close to the theoretical value. It could be seen that the distribution of Ni could be divided into two categories for Ni/Al2O3. Some of the Ni gathered together to form metal particles as shown in TEM images. While the other Ni did not agglomerate and dispersed on the support evenly. This should be ascribed to the unreduced Ni species with high interaction between Al. After the introduction of W, the NiWOx/Al2O3-0.5 had a similar Ni distribution as Ni/Al2O3 (Figure 4D). The EDS mappings of W showed that the Ni and W did not have a separated distribution. The W always distributed followed the distribution trend of Ni. This was advantageous for the synergistic effect between Ni and WOx.
Hydrogenation of HMF
The hydrogenation of HMF was conducted in water under a hydrogen atmosphere. We first checked the effect of W for the reaction, and the results were shown in Figure 5. 46% conversion of HMF was obtained when Ni/Al2O3 without W was used as the catalyst. HHD was detected as one of the products, proving that the reaction could be catalyzed by Ni catalyst. However, the selectivity to HHD was only 29% accompanied by 19% selectivity to 3-(hydroxymethyl)cyclopentan-1-one (HCPO) and 12% selectivity to 2,5-Bis(hydroxymethyl) furan (BHMF). Deep hydrogenation products reported in the literature (Yao et al., 2013; Pomeroy et al., 2021; Zhang et al., 2022) for Ni and Ru based catalysts (Ni–Ce/Al2O3, Ni-Co-Al mixed oxide, and Ru/C) such as 2,5-bis(hydroxymethyl) tetrahydrofuran, 1,2,6-hexanetriol and 1,2,5-hexanetriol were not found, showing that the catalyst had moderate hydrogenation activity. When a small amount of W was introduced to the Ni/Al2O3, the conversion of HMF increased to 68% while the selectivity to HHD decreased a little to 25% for NiWOx/Al2O3-0.1. A small amount of W had no promoting effect for the selectivity to HHD. However, as the amount of W increased, the selectivity to HHD had a sharp increase to 66% for NiWOx/Al2O3-0.5 with a little increase in the conversion of HMF. When continued to increase the content of W, the selectivity to HHD maintained around 60% while the conversion of HMF decreased to 59%. An appropriate amount of W was needed for the high performance for hydrogenation of HMF to HHD. When no Ni was used in the catalyst (WOx/Al2O3), the HMF was almost unchanged showed that the W did not have the function for hydrogenation. The increased activity for the conversion of HMF could be caused by the interaction between Ni and W which was clued by the XRD, XPS, and H2-TPR. Firstly, the electronic state of Ni was changed by the addition of W. Secondly, the interaction between Ni and W weaken that between Ni and Al. So, the content of NiAlO4, a species that had no hydrogenation activity, decreased. Therefore, the hydrogenation activity increased after the introduction of W. The high selectivity to HHD should be ascribed to the strong acid site introduced by the addition of W. It was reported that the acids play a key role in the isomerization of furan rings in the conversion of HMF to HHD. The introduced strong acid sites by W were conducive to the rearrangement of furan rings during the reaction. Thus, the selectivity to HHD was enhanced.
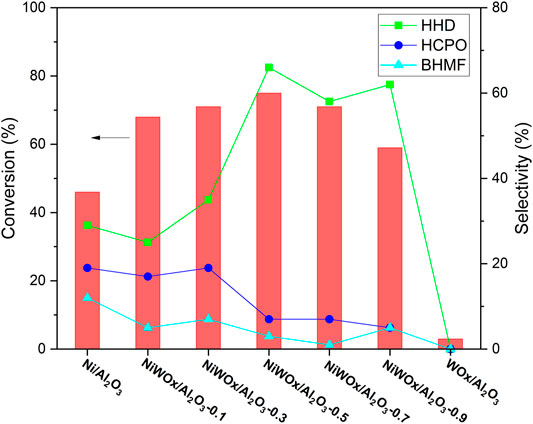
FIGURE 5. The hydrogenation of HMF on different catalyst. Reaction conditions: catalysts (20.0 mg), HMF solution (2.00 g, HMF: 1 mmol), H2 (4 MPa), 413 K, 2 h.
The hydrogenation of HMF to HHD was a multistep reaction including hydrogenation and isomerization. The temperature was very important for this multistep reaction. We studied the effect of temperature by conducting the reaction at a temperature between 333 and 453 K and the results were shown in Figure 6. Generally, the conversion should be increased with the rise in temperature. However, the conversion of HMF experienced a process of falling first and then rising. As shown in Figure 6A, the temperature range was divided into three distinct parts. In each temperature range, the conversion of HMF increased smoothly with the rise in temperature. However, there had a sharp descent in the conversion when the temperature increased from 353 to 363 K and a sharp ascend in the conversion when the temperature increased from 403 to 413 K. We first checked the change of catalysts after reacting at different temperature by XRD (Supplementary Figure S9). It could be seen that, both the NiWOx/Al2O3-0.5 and Al2O3 had no obvious change after reacting at different temperature. This showed the catalysts was stable at the reaction condition. The changes in conversion could be interpreted by the different reaction pathways revealed by the change in selectivity (Figure 6B). At a temperature lower than 363 K, the main product was BHMF which was the hydrogenation of aldehyde in HMF. Both the selectivity to HHD and HCPO, which should be produced by the isomerization of furan rings, was very low. As the reaction temperature raised from 333 to 363 K, the selectivity to BHMF decreased while the selectivity to HHD increased. The maximum increase in the selectivity to HHD was observed when the temperature increased from 353 to 363 K. The hydrogenation active center only required the hydrogenation of aldehyde group for BHMF. However, both the aldehyde group and intermediates needed to be hydrogenated by the hydrogenation active center for the production of HHD. As a result, there had a decline in conversion when the temperature increased from 353 to 363 K. A similar phenomenon also occurred at temperatures increased from 403 to 413 K. The selectivity to HHD decreased sharply when the temperatures increased from 403 to 413 K. At the same time, the selectivity to HCPO began to increase. In a word, the hydrogenation active center should play the role of hydrogenation function in multiple steps that led to the low conversion in the transformation of HMF to HHD. The suitable temperature range for high selectivity to HHD was from 363 to 413 K.
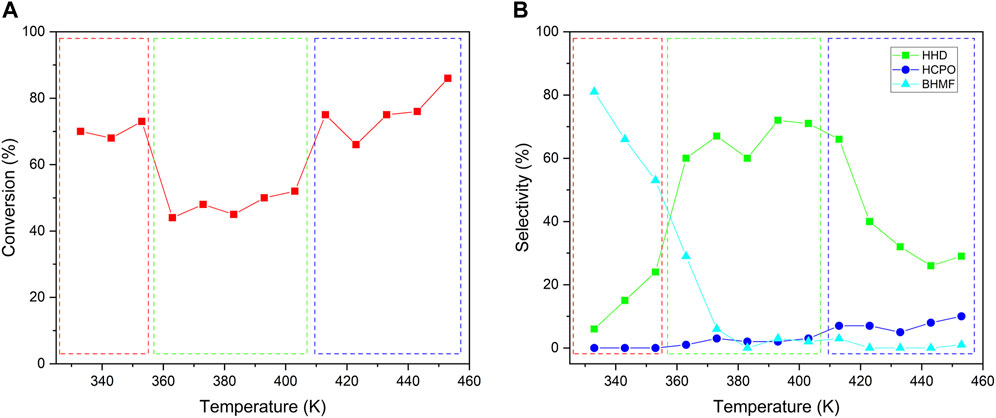
FIGURE 6. The effect of temperature on the conversion (A) and selectivity (B) for HMF conversion. Reaction conditions: NiWOx/Al2O3-0.5 (20.0 mg), HMF solution (2.00 g, HMF: 1 mmol), H2 (4 MPa), 333-453 K, 2 h.
The effect of H2 pressure and reaction time was studied to optimize the reaction conditions. The conversion of HMF increased with the increase in H2 pressure (Figure 7). When the H2 pressure was lower than 4 MPa, the selectivity increased with the increase in pressure. The highest selectivity to HHD was achieved at 4 MPa H2. The conversion increased with the extension of time (Figure 7B). When the reaction was conducted at a shorter time, there had a low selectivity to HHD and BHMF, the intermediate for HHD, was found as the main products. However, as the reaction prolonged to 2 h, the selectivity to HHD increased to 72% while that to BHMF decreased to 3%. The selectivity to HHD kept around 65% when further extension of time. The highest yield of HHD was 59% which was obtained after 6 h reaction.
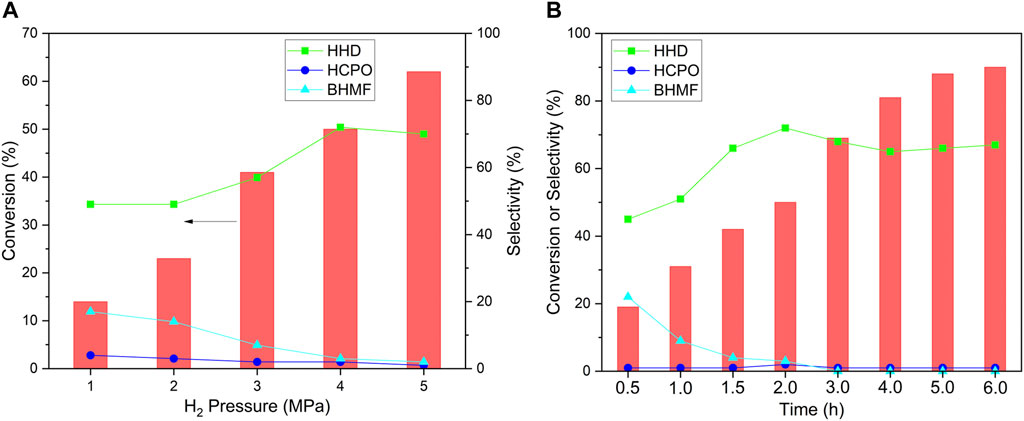
FIGURE 7. The effect of H2 pressure (A) and reaction time (B) for the hydrogenation of HMF. Reaction conditions: NiWOx/Al2O3-0.5 (20.0 mg), HMF solution (2.00 g, HMF: 1 mmol), H2 (1-5 MPa), 393 K, 0.5-6.0 h.
To verify the effect of acid for the conversion of HMF to HHD, the Ni/Al2O3 was used as the catalyst for this transformation with different amounts of acetic acid (AcOH). The results were shown in Table 1. As mentioned above, the selectivity to HHD was 29% without the addition of AcOH. The byproduct or intermediate were HCPO and BHMF. This result was in accordance with the previous report (Perret et al., 2016) that the HHD was one of the products when nickel/alumina was used for the hydrogenation of HMF in water. However, the selectivity to HHD was typically low (less than 15%) when no acid was used. When 10 mg of AcOH was added, there had a little decline in the conversion of HMF. However, the selectivity to HHD increased from 29 to 59% (Table 1, Entry 2). This showed that the acid was conducive to improving the selectivity to HHD rather than the conversion of HMF. Further to increase the amount of acetic acid, there was no significant further improvement in the selectivity to HHD (Table 1, Entries 3-6). The highest selectivity for HHD was 61% which was acquired with 40 mg of AcOH. These experiments proved the role of acid for the high selectivity to HHD.
Based on the characterization and the hydrogenation of HMF, the reaction pathway and the role of catalyst was proposed as shown in Figure 8. The HMF was firstly hydrogenated to BHMF on Ni. Based on the literature and our previous work (Duan et al., 2017b; Martínez-Vargas et al., 2017; Ramos et al., 2017; Fujita et al., 2020), the BHMF was ready to isomerize to 1-hydroxyhex-3-ene-2,5-dione (HHED) catalyzed by acid. This was the crux for the reaction. In this work, the WOx played the role of acid to catalyze this transformation. At last, the HHED was hydrogenated to HHD on Ni. The suitable acidity of WOx and the moderate hydrogenation activity was the key for this multistep tandem reaction. The adjacent distribution of Ni and WOx accelerated the conversion of intermediates thus avoiding possible polymerization side reactions.
Conclusion
In conclusion, the conversion of HMF to HHD was achieved by noble-metal-free W promoted Ni/Al2O3. The Ni and W uniformly dispersed on the surface of the support. The interaction between W and Ni increased the activity of Ni/Al2O3 for the hydrogenation of HMF. The introduction of W generated strong acid sites, which were the key for the high selectivity to HHD. The role of acid was proved by the addition of AcOH to unpromoted Ni/Al2O3. A suitable temperature was needed for the transformation of HMF to HHD smoothly. After the optimization of the conditions, a 59% yield of HHD was acquired at 393 K, 4 MPa H2 reacted for 6 h on NiWOx/Al2O3-0.5. This work provided the idea for high selectivity to HHD from HMF by the introduction of suitable acid and improved the feasibility of putting this reaction into practical application by using a non-noble metal catalyst.
Materials and Method
Materials
HMF (98%, C6H6O3) was bought from Zhengzhou Alpha Chemical Co. Ltd. Aluminum oxide (99.99% metals basis, ≤20 nm, Crystal form: γ-Al2O3), n-decane (99.8%, C10H22) and ammonium metatungstate [99.5% metals basis (NH4)6H2W12O40·xH2O] were purchased from Aladdin Chemistry Co. Ltd. Nickel (II) nitrate hexahydrate [98%, Ni(NO3)2·6H2O] and AcOH (99.5%, C2H4O2) was got from Anhui Zesheng Technology Co., Ltd.
Characterization
A Rigaku D/Max 2500/PC powder diffractometer was used to collect the X-ray diffraction (XRD) patterns. Cu Kα radiation at 40 kV was used as the X-ray source. Thermo Escalab 250Xi spectrometer with Al Kα was used to characterize the X-ray photoelectron spectroscopy (XPS) spectra. The sample powder was overspread on a double-faced adhesive tape on aluminum foil. The sample was pressurized to 8 MPa for 30 s and used for measurement. Before measurement, the chamber pressure was vacuumized to <1 × 10−10 mBar. The binding energy (BE) was adjusted by the binding energy of C1s. The transmission electron microscopy (TEM) images and energy-dispersive spectrometer (EDS) elemental mappings were taken on a JEOL JEM-2100 F field emission transmission electron equipped with An Oxford 80T detector. The temperature-programmed desorption of ammonia (NH3-TPD) and H2-Temperature programmed reduction (H2-TPR) was conducted on the Micromeritics AutoChem II 2920 Instrument. Typically for NH3-TPD, 60.0 mg of sample were loaded into the sample tube. The sample was heated to 773 K under He flow (10 mL/min) and kept for 2 h. The tube was cooled to 373 K and 10NH3-He (30 mL/min) was introduced for 0.5 h. Then the atmosphere was switched to He (10 mL/min) and kept for 1 h to remove the physical adsorbed NH3. After that, the temperature was increased (10 K/min) from 373 to 973 K in an atmosphere of He (10 mL/min). The desorbed NH3 was detected by the thermal conductivity detector (TCD). For H2-TPR, the calcinated catalyst (85.0 mg) was degassed at 573 K under an atmosphere of Ar (10 mL/min) for 2 h. The sample was cooled to 373 K. The temperature was increased (10 K/min) from 373 K to 973 k under the atmosphere of 10H2-Ar (30 mL/min). The H2 consumption was monitored by a TCD detector.
Preparation of Catalysts
In a typical procedure, Ni(NO3)2·6H2O (2.50 g) and (NH4)6H2W12O40·xH2O (1.05 g) were dissolved in water (10.00 g). Then Al2O3 was added to the solution. The mixture was stirred evenly to form a paste and kept standing for 24 h. Then, the paste was dried at 393 k overnight. Followed by calcined at 823 K in the air for 4 h. The obtained solid was ground to pass through 100 mesh sieve and reduced at 773 K in H2 to afford the NiWOx/Al2O3-0.5. The 0.5 referred to the mole ratio of W to Ni.
Catalytic Hydrogenation
The hydrogenation reaction was conducted in a 20 mL stainless steel reactor. Typically, the HMF aqueous solution (2.00 g, HMF: 126.0 mg), catalyst (20.0 mg), and magneton were put into a glass lining. The lining was set in the reactor and sealed and purged with H2 for 4 times to displace the air. Then the reactor was filled with H2 at a specified pressure and put in an oil bath set at a certain temperature. After the reaction, 0.5 mL ethanol solution of the internal standard (n-decane) was added and the mixture was diluted to 10 mL by ethanol. After centrifugation, the liquid was used for analysis. The qualitative analysis was conducted by GC on a Shimadzu GC-2014 equipped with a SH-Rtx-1701 column (30 m × 0.32 mm × 0.25 µm). The oven temperature was started from 353 K for 2 min and raised to 523 K with 20 K/min heating rate. The oven was kept at 523 K for 1.5 min. The GC-MS was performed on Shimadzu GC/MS-TQ8040 equipped with an SH-Rxi-5Sil MS column (30 m × 0.25 mm × 0.25 µm). The oven temperature was started from 323 K for 1 min and raised to 473 K with 40 K/min heating rate and then raised to 553 K and kept at the temperature for 5 min.
Data Availability Statement
The original contributions presented in the study are included in the article/supplementary material, further inquiries can be directed to the corresponding author.
Author Contributions
RW, QL, and XQ conducted the experiments. ZL wrote the draft manuscript. YD designed the ideas and revised the manuscript.
Funding
This work was supported by the National Natural Science Foundation of China (21801110 and 21902071), the Department of Education of Henan Province (2021GGJS133), and Luoyang Normal University (2019XJGGJS-05).
Conflict of Interest
The authors declare that the research was conducted in the absence of any commercial or financial relationships that could be construed as a potential conflict of interest.
Publisher’s Note
All claims expressed in this article are solely those of the authors and do not necessarily represent those of their affiliated organizations, or those of the publisher, the editors and the reviewers. Any product that may be evaluated in this article, or claim that may be made by its manufacturer, is not guaranteed or endorsed by the publisher.
Supplementary Material
The Supplementary Material for this article can be found online at: https://www.frontiersin.org/articles/10.3389/fchem.2022.857199/full#supplementary-material
References
Averochkin, G. M., Gordeev, E. G., Skorobogatko, M. K., Kucherov, F. A., and Ananikov, V. P. (2021). Systematic Study of Aromatic‐Ring‐Targeted Cycloadditions of 5‐Hydroxymethylfurfural Platform Chemicals. ChemSusChem 14, 3110–3123. doi:10.1002/cssc.202100818
Besson, M., Gallezot, P., and Pinel, C. (2014). Conversion of Biomass into Chemicals over Metal Catalysts. Chem. Rev. 114, 1827–1870. doi:10.1021/cr4002269
Bielski, R., and Grynkiewicz, G. (2021). Furan Platform Chemicals beyond Fuels and Plastics. Green. Chem. 23, 7458–7487. doi:10.1039/d1gc02402g
Cao, Y., Wang, J., Kang, M., and Zhu, Y. (2014). Efficient Synthesis of Ethylene Glycol from Cellulose over Ni-WO3/SBA-15 Catalysts. J. Mol. Catal. A: Chem. 381, 46–53. doi:10.1016/j.molcata.2013.10.002
Chang, S., He, X., Li, B., and Pan, X. (2021). Improved Bio-Synthesis of 2,5-Bis(Hydroxymethyl)Furan by Burkholderia Contaminans NJPI-15 with Co-substrate. Front. Chem. 9, 635191. doi:10.3389/fchem.2021.635191
Che, P., Lu, F., Nie, X., Huang, Y., Yang, Y., Wang, F., et al. (2015). Hydrogen Bond Distinction and Activation upon Catalytic Etherification of Hydroxyl Compounds. Chem. Commun. 51, 1077–1080. doi:10.1039/c4cc08467e
Corma, A., Iborra, S., and Velty, A. (2007). Chemical Routes for the Transformation of Biomass into Chemicals. Chem. Rev. 107, 2411–2502. doi:10.1021/cr050989d
Das, B., and Mohanty, K. (2021). Sulfonic Acid-Functionalized Carbon Coated Red Mud as an Efficient Catalyst for the Direct Production of 5-HMF from D-Glucose under Microwave Irradiation. Appl. Catal. A: Gen. 622, 118237. doi:10.1016/j.apcata.2021.118237
Deshan, A. D. K., Atanda, L., Moghaddam, L., Rackemann, D. W., Beltramini, J., and Doherty, W. O. S. (2020). Heterogeneous Catalytic Conversion of Sugars into 2,5-Furandicarboxylic Acid. Front. Chem. 8, 659. doi:10.3389/fchem.2020.00659
Duan, Y., Zhang, J., Li, D., Deng, D., Ma, L.-F., and Yang, Y. (2017a). Direct Conversion of Carbohydrates to Diol by the Combination of Niobic Acid and a Hydrophobic Ruthenium Catalyst. RSC Adv. 7, 26487–26493. doi:10.1039/c7ra03939e
Duan, Y., Zheng, M., Li, D., Deng, D., Ma, L.-F., and Yang, Y. (2017b). Conversion of HMF to Methyl Cyclopentenolone Using Pd/Nb2O5 and Ca-Al Catalysts via a Two-step Procedure. Green. Chem. 19, 5103–5113. doi:10.1039/C7GC02310C
Elsayed, I., Jackson, M. A., and Hassan, E. B. (2020). Hydrogen-Free Catalytic Reduction of Biomass-Derived 5-Hydroxymethylfurfural into 2,5-Bis(Hydroxymethyl)Furan Using Copper-Iron Oxides Bimetallic Nanocatalyst. ACS Sust. Chem. Eng. 8, 1774–1785. doi:10.1021/acssuschemeng.9b05575
Esteves, L. M., Brijaldo, M. H., Oliveira, E. G., Martinez, J. J., Rojas, H., Caytuero, A., et al. (2020). Effect of Support on Selective 5-Hydroxymethylfurfural Hydrogenation towards 2,5-Dimethylfuran over Copper Catalysts. Fuel 270, 117524. doi:10.1016/j.fuel.2020.117524
Fan, W., Verrier, C., Queneau, Y., and Popowycz, F. (2019). 5-Hydroxymethylfurfural (HMF) in Organic Synthesis: A Review of its Recent Applications towards Fine Chemicals. Curr. Org. Synth. 16, 583–614. doi:10.2174/1570179416666190412164738
Fang, R., Dhakshinamoorthy, A., Li, Y., and Garcia, H. (2020). Metal Organic Frameworks for Biomass Conversion. Chem. Soc. Rev. 49, 3638–3687. doi:10.1039/d0cs00070a
Fang, W., and Riisager, A. (2021). Recent Advances in Heterogeneous Catalytic Transfer Hydrogenation/Hydrogenolysis for Valorization of Biomass-Derived Furanic Compounds. Green. Chem. 23, 670–688. doi:10.1039/D0GC03931D
Fujita, S., Nakajima, K., Yamasaki, J., Mizugaki, T., Jitsukawa, K., and Mitsudome, T. (2020). Unique Catalysis of Nickel Phosphide Nanoparticles to Promote the Selective Transformation of Biofuranic Aldehydes into Diketones in Water. ACS Catal. 10, 4261–4267. doi:10.1021/acscatal.9b05120
Fulignati, S., Antonetti, C., Wilbers, E., Licursi, D., Heeres, H. J., and Raspolli Galletti, A. M. (2021). Tunable HMF Hydrogenation to Furan Diols in a Flow Reactor Using Ru/C as Catalyst. J. Ind. Eng. Chem. 100, 390. doi:10.1016/j.jiec.2021.04.057
Gao, G., Jiang, Z., and Hu, C. (2021). Selective Hydrogenation of the Carbonyls in Furfural and 5-Hydroxymethylfurfural Catalyzed by PtNi Alloy Supported on SBA-15 in Aqueous Solution under Mild Conditions. Front. Chem. 9, 759512. doi:10.3389/fchem.2021.759512
Guo, W., Zhang, Z., Hacking, J., Heeres, H. J., and Yue, J. (2021). Selective Fructose Dehydration to 5-Hydroxymethylfurfural from a Fructose-Glucose Mixture over a Sulfuric Acid Catalyst in a Biphasic System: Experimental Study and Kinetic Modelling. Chem. Eng. J. 409, 128182. doi:10.1016/j.cej.2020.128182
Gupta, K., Tyagi, D., Dwivedi, A. D., Mobin, S. M., and Singh, S. K. (2015). Catalytic Transformation of Bio-Derived Furans to Valuable Ketoacids and Diketones by Water-Soluble Ruthenium Catalysts. Green. Chem. 17, 4618–4627. doi:10.1039/c5gc01376c
Han, W., Tang, M., Li, J., Li, X., Wang, J., Zhou, L., et al. (2020). Selective Hydrogenolysis of 5-Hydroxymethylfurfural to 2,5-Dimethylfuran Catalyzed by Ordered Mesoporous Alumina Supported Nickel-Molybdenum Sulfide Catalysts. Appl. Catal. B: Environ. 268, 118748. doi:10.1016/j.apcatb.2020.118748
Kang, P.-L., Shang, C., and Liu, Z.-P. (2019). Glucose to 5-Hydroxymethylfurfural: Origin of Site-Selectivity Resolved by Machine Learning Based Reaction Sampling. J. Am. Chem. Soc. 141, 20525–20536. doi:10.1021/jacs.9b11535
Karve, V. V., Sun, D. T., Trukhina, O., Yang, S., Oveisi, E., Luterbacher, J., et al. (2020). Efficient Reductive Amination of HMF with Well Dispersed Pd Nanoparticles Immobilized in a Porous MOF/Polymer Composite. Green. Chem. 22, 368–378. doi:10.1039/c9gc03140e
Liu, F., Audemar, M., De Oliveira Vigier, K., Clacens, J.-M., De Campo, F., and Jérôme, F. (2014). Combination of Pd/C and Amberlyst-15 in a Single Reactor for the Acid/Hydrogenating Catalytic Conversion of Carbohydrates to 5-Hydroxy-2,5-Hexanedione. Green. Chem. 16, 4110–4114. doi:10.1039/c4gc01158a
Long, J., Xu, Y., Zhao, W., Li, H., and Yang, S. (2019). Heterogeneous Catalytic Upgrading of Biofuranic Aldehydes to Alcohols. Front. Chem. 7, 529. doi:10.3389/fchem.2019.00529
Martínez-Vargas, D. X., Rivera De La Rosa, J., Sandoval-Rangel, L., Guzmán-Mar, J. L., Garza-Navarro, M. A., Lucio-Ortiz, C. J., et al. (2017). 5-Hydroxymethylfurfural Catalytic Oxidation under Mild Conditions by Co (II), Fe (III) and Cu (II) Salen Complexes Supported on SBA-15: Synthesis, Characterization and Activity. Appl. Catal. A: Gen. 547, 132–145. doi:10.1016/j.apcata.2017.08.035
Mika, L. T., Cséfalvay, E., and Németh, Á. (2017). Catalytic Conversion of Carbohydrates to Initial Platform Chemicals: Chemistry and Sustainability. Chem. Rev. 118, 505–613. doi:10.1021/acs.chemrev.7b00395
Nagpure, A. S., Gogoi, P., Lucas, N., and Chilukuri, S. V. (2020). Novel Ru Nanoparticle Catalysts for the Catalytic Transfer Hydrogenation of Biomass-Derived Furanic Compounds. Sust. Energ. Fuels 4, 3654–3667. doi:10.1039/D0SE00361A
Neațu, F., Marin, R. S., Florea, M., Petrea, N., Pavel, O. D., and Pârvulescu, V. I. (2016). Selective Oxidation of 5-Hydroxymethyl Furfural over Non-precious Metal Heterogeneous Catalysts. Appl. Catal. B: Environ. 180, 751–757. doi:10.1016/j.apcatb.2015.07.043
Ohyama, J., Kanao, R., Esaki, A., and Satsuma, A. (2014). Conversion of 5-Hydroxymethylfurfural to a Cyclopentanone Derivative by Ring Rearrangement over Supported Au Nanoparticles. Chem. Commun. 50, 5633–5636. doi:10.1039/c3cc49591d
Ohyama, J., Kanao, R., Ohira, Y., and Satsuma, A. (2016). The Effect of Heterogeneous Acid-Base Catalysis on Conversion of 5-hydroxymethylfurfural into a Cyclopentanone Derivative. Green. Chem. 18, 676–680. doi:10.1039/c5gc01723h
Ohyama, J., Ohira, Y., and Satsuma, A. (2017). Hydrogenative Ring-Rearrangement of Biomass Derived 5-(hydroxymethyl)furfural to 3-(hydroxymethyl)cyclopentanol Using Combination Catalyst Systems of Pt/SiO2 and Lanthanoid Oxides. Catal. Sci. Technol. 7, 2947–2953. doi:10.1039/c7cy00712d
Perret, N., Grigoropoulos, A., Zanella, M., Manning, T. D., Claridge, J. B., and Rosseinsky, M. J. (2016). Catalytic Response and Stability of Nickel/Alumina for the Hydrogenation of 5-Hydroxymethylfurfural in Water. ChemSusChem 9, 521–531. doi:10.1002/cssc.201501225
Pomeroy, B., Grilc, M., and Likozar, B. (2021). Process Condition-Based Tuneable Selective Catalysis of Hydroxymethylfurfural (HMF) Hydrogenation Reactions to Aromatic, Saturated Cyclic and Linear Poly-Functional Alcohols over Ni-Ce/Al2O3. Green. Chem. 23, 7996–8002. doi:10.1039/d1gc02086b
Ramos, R., Grigoropoulos, A., Perret, N., Zanella, M., Katsoulidis, A. P., Manning, T. D., et al. (2017). Selective Conversion of 5-hydroxymethylfurfural to Cyclopentanone Derivatives over Cu-Al2O3 and Co-Al2O3 Catalysts in Water. Green. Chem. 19, 1701–1713. doi:10.1039/C7GC00315C
Ramos, R., Grigoropoulos, A., Griffiths, B. L., Katsoulidis, A. P., Zanella, M., Manning, T. D., et al. (2019). Selective Conversion of 5-Hydroxymethylfurfural to Diketone Derivatives over Beta Zeolite-Supported Pd Catalysts in Water. J. Catal. 375, 224–233. doi:10.1016/j.jcat.2019.04.038
Ren, D., Song, Z., Li, L., Liu, Y., Jin, F., and Huo, Z. (2016). Production of 2,5-Hexanedione and 3-Methyl-2-Cyclopenten-1-One from 5-Hydroxymethylfurfural. Green. Chem. 18, 3075–3081. doi:10.1039/c5gc02493e
Schiavo, V., Descotes, G., and Mentech, J. (1991). Catalytic-Hydrogenation of 5-Hydroxymethylfurfural in Aqueous-Medium. Bull. Soc. Chim. Fr. 5, 704–711.
Tempelman, C. H. L., Jacobs, J. F., Ramkhelawan, S., Mok, A., van der Zalm, W., and Degirmenci, V. (2021). Processing of Agricultural Apple Fruit Waste into Sugar Rich Feedstocks for the Catalytic Production of 5-HMF over a Sn Amberlyst-15 Resin Catalyst. J. Ind. Eng. Chem. 99, 443–448. doi:10.1016/j.jiec.2021.04.056
Wang, T., Wei, J., Liu, H., Feng, Y., Tang, X., Zeng, X., et al. (2020). Synthesis of Renewable Monomer 2, 5-Bishydroxymethylfuran from Highly Concentrated 5-Hydroxymethylfurfural in Deep Eutectic Solvents. J. Ind. Eng. Chem. 81, 93–98. doi:10.1016/j.jiec.2019.08.057
Wiesfeld, J. J., Kim, M., Nakajima, K., and Hensen, E. J. M. (2020). Selective Hydrogenation of 5-Hydroxymethylfurfural and its Acetal with 1,3-Propanediol to 2,5-Bis(Hydroxymethyl)Furan Using Supported Rhenium-Promoted Nickel Catalysts in Water. Green. Chem. 22, 1229–1238. doi:10.1039/c9gc03856f
Wozniak, B., Spannenberg, A., Li, Y., Hinze, S., and de Vries, J. G. (2018). Cyclopentanone Derivatives from 5-Hydroxymethylfurfural via 1-Hydroxyhexane-2,5-Dione as Intermediate. ChemSusChem 11, 356–359. doi:10.1002/cssc.201702100
Wozniak, B., Tin, S., and de Vries, J. G. (2019). Bio-Based Building Blocks from 5-Hydroxymethylfurfural via 1-Hydroxyhexane-2,5-Dione as Intermediate. Chem. Sci. 10, 6024–6034. doi:10.1039/c9sc01309a
Xu, C., Paone, E., Rodríguez-Padrón, D., Luque, R., and Mauriello, F. (2020). Recent Catalytic Routes for the Preparation and the Upgrading of Biomass Derived Furfural and 5-Hydroxymethylfurfural. Chem. Soc. Rev. 49, 4273–4306. doi:10.1039/d0cs00041h
Xu, Y.-J., Shi, J., Wu, W.-P., Zhu, R., Li, X.-L., Deng, J., et al. (2017). Effect of Cp*Iridium(III) Complex and Acid Co-catalyst on Conversion of Furfural Compounds to Cyclopentanones or Straight Chain Ketones. Appl. Catal. A: Gen. 543, 266–273. doi:10.1016/j.apcata.2017.07.004
Yang, Y., Deng, D., Sui, D., Xie, Y., Li, D., and Duan, Y. (2019a). Facile Preparation of Pd/UiO-66-V for the Conversion of Furfuryl Alcohol to Tetrahydrofurfuryl Alcohol under Mild Conditions in Water. Nanomaterials 9, 1698. doi:10.3390/nano9121698
Yang, Y., Ma, J., Jia, X., Du, Z., Duan, Y., and Xu, J. (2016). Aqueous Phase Hydrogenation of Furfural to Tetrahydrofurfuryl Alcohol on Alkaline Earth Metal Modified Ni/Al2O3. RSC Adv. 6, 51221–51228. doi:10.1039/c6ra05680f
Yang, Y., Xie, Y., Deng, D., Li, D., Zheng, M., and Duan, Y. (2019b). Highly Selective Conversion of HMF to 1‐hydroxy‐ 2,5‐hexanedione on Pd/MIL‐101(Cr). ChemistrySelect 4, 11165–11171. doi:10.1002/slct.201903535
Yang, Y., Yang, D., Zhang, C., Zheng, M., and Duan, Y. (2020). Preparation of 1-Hydroxy-2,5-Hexanedione from HMF by the Combination of Commercial Pd/C and Acetic Acid. Molecules 25, 2475. doi:10.3390/molecules25112475
Yao, S., Wang, X., Jiang, Y., Wu, F., Chen, X., and Mu, X. (2013). One-Step Conversion of Biomass-Derived 5-Hydroxymethylfurfural to 1,2,6-Hexanetriol over Ni-Co-Al Mixed Oxide Catalysts under Mild Conditions. ACS Sust. Chem. Eng. 2, 173–180. doi:10.1021/sc4003714
Yu, I. K. M., and Tsang, D. C. W. (2017). Conversion of Biomass to Hydroxymethylfurfural: A Review of Catalytic Systems and Underlying Mechanisms. Bioresour. Tech. 238, 716–732. doi:10.1016/j.biortech.2017.04.026
Zhang, C., Li, Y., Lv, X., Gao, X., Duan, Y., Sui, D., et al. (2022). Catalytic Hydrogenation of 5‐Hydroxymethylfurfural to Hexanetriol. ChemistrySelect 7, 616–630. doi:10.1002/slct.202103797
Zhang, J., Jia, W., Sun, Y., Yang, S., Tang, X., Zeng, X., et al. (2021). An Efficient Approach to Synthesizing 2,5-Bis(N-Methyl-Aminomethyl)Furan from 5-Hydroxymethylfurfural via 2,5-Bis(N-Methyl-Iminomethyl)Furan Using a Two-step Reaction in One Pot. Green. Chem. 23, 5656–5664. doi:10.1039/d1gc01635k
Zhang, S., Ma, H., Sun, Y., Luo, Y., Liu, X., Zhang, M., et al. (2019). Catalytic Selective Hydrogenation and Rearrangement of 5-Hydroxymethylfurfural to 3-Hydroxymethyl-Cyclopentone over a Bimetallic Nickel-Copper Catalyst in Water. Green. Chem. 21, 1702–1709. doi:10.1039/C8GC04009E
Keywords: Ni/Al2O3, HMF, hydrogenation, W promoted, hydroxy-hexanedione
Citation: Duan Y, Wang R, Liu Q, Qin X and Li Z (2022) Tungsten Promoted Ni/Al2O3 as a Noble-Metal-Free Catalyst for the Conversion of 5-Hydroxymethylfurfural to 1-Hydroxy-2,5-Hexanedione. Front. Chem. 10:857199. doi: 10.3389/fchem.2022.857199
Received: 18 January 2022; Accepted: 09 February 2022;
Published: 09 March 2022.
Edited by:
Mithun Kumar Ghosh, Pandit S. N. Shukla University, IndiaReviewed by:
Yoshinao Nakagawa, Tohoku University, JapanSandip Kumar Chandraker, Indira Gandhi National Tribal University, India
Copyright © 2022 Duan, Wang, Liu, Qin and Li. This is an open-access article distributed under the terms of the Creative Commons Attribution License (CC BY). The use, distribution or reproduction in other forums is permitted, provided the original author(s) and the copyright owner(s) are credited and that the original publication in this journal is cited, in accordance with accepted academic practice. No use, distribution or reproduction is permitted which does not comply with these terms.
*Correspondence: Ying Duan, duanying@mail.ustc.eud.cn