Gadolinium-Coated Mesoporous Silica Nanoparticle for Magnetic Resonance Imaging
- 1Department of Molecular Imaging, School of Medical Technology, Qiqihar Medical University, Qiqihar, China
- 2Department of Radiology, The First Affiliated Hospital of Qiqihar Medical University, Qiqihar, China
- 3Department of Molecular Imaging, The First Affiliated Hospital of Qiqihar Medical University, Qiqihar, China
Magnetic resonance molecular imaging can provide anatomic, functional and molecular information. However, because of the intrinsically low sensitivity of magnetic resonance imaging (MRI), high-performance MRI contrast agents are required to generate powerful image information for image diagnosis. Herein, we describe a novel T1 contrast agent with magnetic-imaging properties facilitated by the gadolinium oxide (Gd2O3) doping of mesoporous silica nanoparticles (MSN). The size, morphology, composition, MRI relaxivity (r1), surface area and pore size of these nanoparticles were evaluated following their conjugation with Gd2O3 to produce Gd2O3@MSN. This unique structure led to a significant enhancement in T1 contrast with longitudinal relaxivity (r1) as high as 51.85 ± 1.38 mM−1s−1. Gd2O3@MSN has a larger T1 relaxivity than commercial gadolinium diethylene triamine pentaacetate (Gd-DTPA), likely due to the geometrical confinement effect of silica nanoparticles. These results suggest that we could successfully prepare a novel high-performance T1 contrast agent, which may be a potential candidate for in-vivo MRI.
1 Introduction
Magnetic resonance imaging (MRI) is non-invasive and produces high-resolution morphological and innate three-dimensional image resolution without the risk of radiation damage, making it a critical clinical diagnostic tool. MRI contrast agents are a specific substrate used in these technologies to help improve the contrast between normal and abnormal tissues. Gadolinium diethylene triamine pentaacetate (Gd-DTPA) remains the most common MRI contrast agent as it can enhance the brightness of the region of interest (ROI; positive contrast) (Shin et al., 2015; Ni et al., 2017; Shen et al., 2017). However,Gd-DTPA is a small-molecule contrast agent and suffers from relatively low sensitivity, specificity, and relaxivity, which limits its applications in more complex diagnostic settings (Fraum et al., 2017; Vikas et al., 2017). This implies that alternative contrast agents, specifically those commonly referred to as gadolinium-based T1 contrast materials have begun to attract more attention.
In addition, nano-drug delivery systems (NDDS) are currently amongst the most evaluated drug delivery systems in the world. These NDDS combine a therapeutic payload with nano-sized carriers (such as liposomes, gold nanoparticles, polymeric micelles, and mesoporous silica) to reduce side effects and prolong circulation time (Mikada et al., 2017; Layek et al., 2020). Thus, the introduction of MRI contrast agents into an NDDS may both extend blood circulation time and improve MRI performance (because of the shortened longitudinal proton relaxation time of the surrounding water molecules for T1-type contrast agents) (Lin et al., 2004; Parvesh et al., 2012; Li et al., 2014; Hu et al., 2015; Deng et al., 2016; Wang and Sun, 2020). Of the common NDDS carriers mesoporous silica nanoparticles (MSNs) are widely used in the carriers for drug delivery and biosensing and are likely to be the most appropriate carrier for gadolinium owing to their high surface area, ease of preparation, good biocompatibility, and mesoporous structure (Lin et al., 2004; Taylor et al., 2008; Xia et al., 2019). Additionally, the overall structure is composed of silica and Si-OH groups. Si-O-Si frameworks are quitestable and silica degradation is relatively difficult under physiological conditions, which means that these particles are likely to facilitate good loading of Gd2O3 but inhibit the release of free Gd3+ reducing its toxic effects.
Herein, we report a novel synthesis system for producing Gd3+-incorporated MSN (Gd2O3@MSN), which are characterised by a mesoporous structure, higher surface area and high T1 relaxivity. These nanoparticles (NPs) are easy to prepare and modify, present with low-cost, and possess desirable MRI contrast-enhancement properties, thus making them suitable for the creation of more specific, and possibly even targeted, contrast agents for molecular MRI and could help provide real-time feedback for treatment outcomes (Scheme 1), potentially enhancing the clinical utility of MRI.
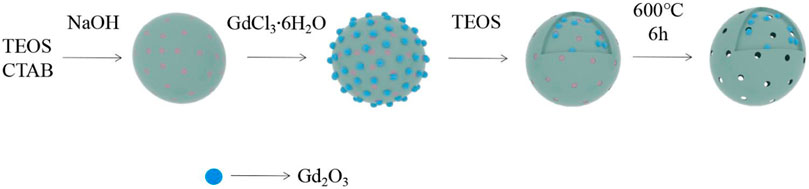
SCHEME 1. Illustration of the preparation of Gd3+-incorporated mesoporous silica nanoparticles (MSN) (Gd2O3@MSN) particles for magnetic resonance imaging.
2 Materials and Methods
2.1 Materials
Hexadecyl trimethyl ammonium bromide (CTAB, 99%) was purchased from Coolaber Science & Technology. Tetraethoxysilane (TEOS, 99%) was purchased from Fuchen Chemical Reagents (Tianjin, China). 3-Aminopropyl-triethoxysilane (99%) was purchased from Macklin Biochemical Co., Ltd. (Shanghai, China). Gadolinium (III) chloride hexahydrate (GdCl3·6H2O, 99.9%) was purchased from Aladdin Biochemical Technology Co., Ltd. (Shanghai China).
2.2 Preparation of Gd2O3@MSN
Briefly, NaOH (140 mg) and CTAB (500 mg) were dissolved in 220 ml of deionised water and stirred (300 rpm) at 80°C for 1 h. Next, we slowly added TEOS (1.8 ml) in a dropwise manner to this suspension while maintaining the stirring (250 rpm) of the recipient solution. This mixture was then stirred for another 2 h and then 20 ml of GdCl3·6H2O aqueous solution (5 mg/ml) was quickly added into the mixture. One hour later, an additional 0.7 ml of TEOS was added to these samples, left for an additional 2 h, centrifuged, washed with ethanol and deionised water, and then dried in an oven at 50°C for 24 h. The resulting Gd2O3@MSN were collected and calcined at 600°C for 6 h to remove CTAB surfactants.
2.3 Characterizations
Gd2O3@MSN (1 mg/ml) particle size and zeta potential was confirmed by dynamic light scattering (Malvern Zetasizer Nano ZS system, Malvern, Worcestershire, United Kingdom). We then performed transmission electron microscopy (TEM) (FEI Talos F200S, United States) to examine the surface morphology of the Gd2O3@MSN particles. Their composition was evaluated by energy dispersive X-ray spectroscopy (EDS). Scanning transmission electron microscopy high-angle annular dark-field (STEM-HAADF) images and energy-dispersive X-ray (EDX) element mapping images were obtained using an FEI Talos F200S microscope at an accelerating voltage of 300 kV. The analysis of the N2-adsorption isotherms was performed using Barrett–Joyner–Halenda (BJH) analysis (Micromeritics ASAP-2460, Norcross, GA, United States). The surface area, total pore volume, and average pore distribution curves for the MSNs were determined using the Brunauer–Emmett–Teller (BET) method. Fourier transform infrared refraction (FT-IR, RF-5301PC, Shimadzu, Japan) analyses of the Gd2O3@MSN particles were performed in the range of 400–4,000 cm−1 or structural characterization.
2.4 T1 Relaxivity and in-vitro Magnetic Resonance Imaging of as-prepared NPs
The Gd3+ concentration of the doped MSNs was proved using Inductively-coupled plasma mass spectrometry (ICP-MS, Agilent 720 ES, United States), and ICP-MS was also used to detect whether free Gd elements were dissociated from Gd2O3@MSNs when immersed in phosphate-buffered saline (PBS) at different pH (7.4, 5.5 and 4.5) for 48 h. Next we evaluated the T1 relaxivity of Gd2O3@MSN and Gd-DTPA with different molar concentrations of gadolinium when kept in 1% agarose solution using a 0.5 T NMI20 Analyst NMR system (Niumag Analytical Instrument Corporation, Sunzhou, China) (Repeat three times for each sample) set to apply the following parameters:SW:100 kHz, TW: 3,000 ms, RFD: 0.08 ms, NS: 8, TE: 1 ms and NTI: 25 (in pure water, 37°C), and the T1 graph was obtained using the inversion recovery (T1-TSE) sequence: TR/TI: 3,000/20 ms, TE: 20 ms, matrix: 256 × 192, layer thickness: 3 mm, FOV: 90 × 120 mm.
2.5 In-Vitro Evaluations
2.5.1 Cell Culture
The human pancreatic cancer cell line AsPC-1, PaCa-2 and 4T1 breast cancer line were purchased from the Shanghai Cell Bank of the Chinese Academy of Sciences and cultured in Roswell Park Memorial Institute 1,640 (RPMI 1640) medium containing 10% (v/v) foetal bovine serum (FBS) and 1% penicillin/streptomycin. The cell lines were cultured using regular cell culture conditions (37°C with 5% CO2).
2.5.2 Cytotoxicity Assay
AsPC-1, PaCa-2 and 4T1 cells were separately plated in 96-well plates (5 × 103cells/well) with 100 μL of medium and incubated for 24 h before the culture medium was replaced with 100 μL of RPMI 1640 supplemented with different concentrations of Gd2O3@MSN (5, 25, 50, 100, 150 and 200 μg/ml) and incubated for an additional 24 h. The drug-containing culture medium was then removed and replaced with 100 μL of fresh medium and 10 μL of CCK-8 and incubated for 2 h before measuring the absorbance at 490 nm using a plate reader (SAFIRE2, TECAN, Switzerland). Cell viability was then calculated using the following equation: Cell viability (%) = (Atreated–Ablank)/(Acontrol–Ablank) × 100%. where Atreated, Acontrol and Ablank represent the absorbance of the treated, control and blank wells, respectively.
2.6 In-Vivo Evaluations
2.6.1 Experimental Animals
Male SPF-grade Sprague–Dawley (SD) rats (180 ± 10 g) were purchased from Liaoning Changsheng biotechnology Co., Ltd. (SXK2020-0001) and housed as prescribed. The animal experiments were approved by the Animal Ethics Committee of Qiqihar Medical University (No. QMU-AECC-2021-168).
2.6.2 In-Vivo Toxicity Studies
Ten healthy SD rats (180 ± 10 g) were randomly divided into two groups (five rats in each). The rats were then injected with 100 mg/kg Gd2O3@MSN or saline. After 7 days, all the rats were sacrificed and approximately 3 ml of blood was collected from each rat for blood chemistry evaluations immediately before being euthanised. Then, the major organs, namely the heart, liver, spleen, lung, and kidneys, were harvested from those rats for H&E staining and histopathological examination (Leica-DM4B digital microscope, Germany).
2.6.3 In-Vivo Magnetic Resonance Imaging Studies
These experiments were performed using a Philips (Achieva 3.0 T) MRI scanner with 8-channel carotid wall imaging special phased array coil. Male SD rats were selected for T1-weighted MRI from each group (n = 3) and injected with Gd2O3@MSN or Gd-DTPA at 0.5 mg of Gd3+ per kg of body weight. The images were then produced using a T1 sequence with the following parameters: TR/TE = 650/10 ms, thickness = 3 mm, 192 × 192 matrices, FOV = 130 × 130 mm and flip angle = 90°. The signal-to-noise ratio (SNR) for each image was then calculated by analysing each ROI (in each image). Contrast enhancement was defined as an increase in SNR after injection using the following equation:
All image data were transferred to a remote computer for analysis.
2.7 Statistical Analysis
Nanoparticle size was analysed using the Nano Measure 1.2 software, and SPSS 20.0 (SPSS Inc., Chicago, United States) was used for data management and statistical analysis (Student’s t-test for unpaired data). The data are expressed as the mean ± standard deviation. A p-value of <0.05 is considered statistically significant and the SNR values were determined using ImageJ.
3 Results and Discussion
3.1 Preparation and Characterization of Gd2O3@MSN
Our preparation used CTAB as the template and TEOS as the main silica source. First, solid silica nanospheres containing the CTAB template were prepared and then Gd3+ was converted to Gd(OH)3 in alkaline solution (pH = 9), before being used to coat the surface of the SiO2 nanospheres. Once the mesoporous silica shell successfully coated the solid core, the final product was calcined at 600°C for 6 h to remove the CTAB template and dehydrate Gd(OH)3 to Gd2O3 (Scheme 2).
Figure 1 described the structural characteristics of the prepared Gd2O3@MSN. TEM (Figures 1A–C) revealed that these NPs were spherical or ellipsoid in shape and of uniform size and distribution, and the mean diameter of these NPs was determined to be 86.85 ± 10.44 nm. Dynamic light scattering (DLS) curves (Figure 1D) revealed that the average diameter of the NPs was 162.50 nm, suggesting that the DLS measured these NPs as slightly larger than the TEM, which might be the result of the rehydration of the NPs in the aqueous solution used for the DLS evaluation. As expected, the zeta potential of the MSN was negative (Figure 1E), whereas the moderate size of these particles should help avoid renal clearance and uptake to the reticuloendothelial system (RES) in the liver, which were both essential for increasing circulation time (Phillps et al., 2010; Koo et al., 2011; Shao et al., 2011; O’Connell et al., 2017; Jiang et al., 2019).
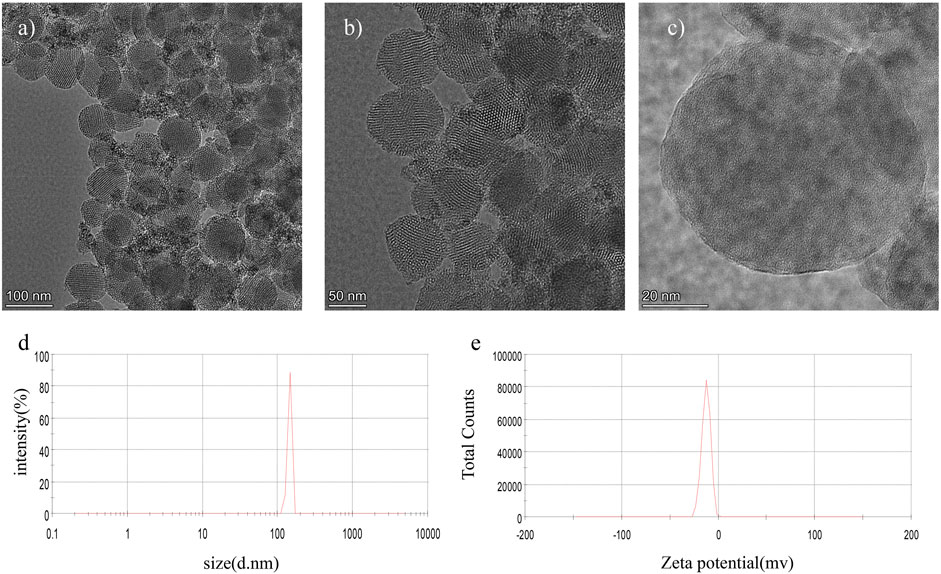
FIGURE 1. Structural evaluation of Gd2O3@mesoporous silica nanoparticles by (A–C) transmission electron microscopy; (D) dynamic light scattering; and (E) zeta potential evaluation.
The EDS (Figure 2B) spectrum confirmed that the red circle appearing in Figure 2A indicates Gd-existence with an atomic fraction of 0.91% (atom.%) Gd (Table 1). ICP-MS (Figure 2C) determined the overall Gd concentration to be 1.01 (atom. %). We then investigated the structure of NPs in detail. STEM-HAADF images (Figure 3A) and EDX elemental mapping images showed the significant and homogeneous signal of Gd in MSNs (Figures 3B–E), indicating the Gd3+ elements were evenly distributed across MSN structure.
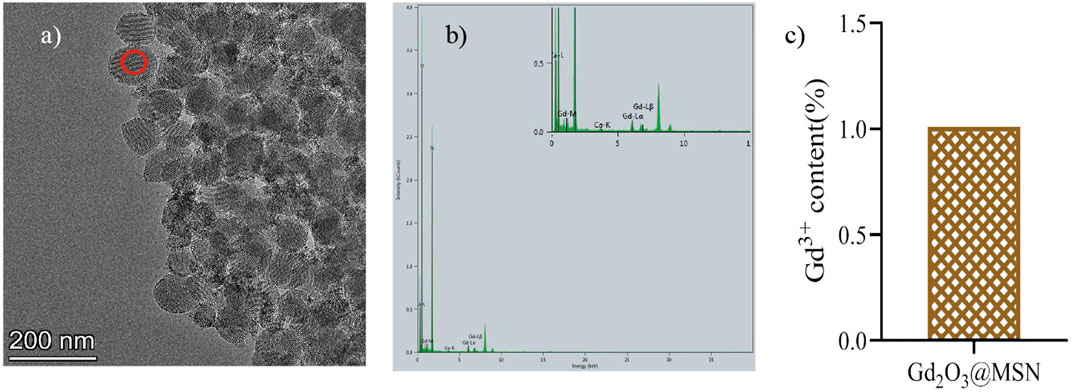
FIGURE 2. (A) Transmission electron microscopy images and (B) Energy dispersive spectrometer spectra of the Gd2O3@mesoporous silica nanoparticles (MSN) particles; (C) the Gd content in Gd2O3@MSN as determined by Inductively-coupled plasma mass spectrometry.

TABLE 1. Results of energy dispersive X-ray spectroscopy analysis of the Gd2O3@mesoporous silica nanoparticles particles.
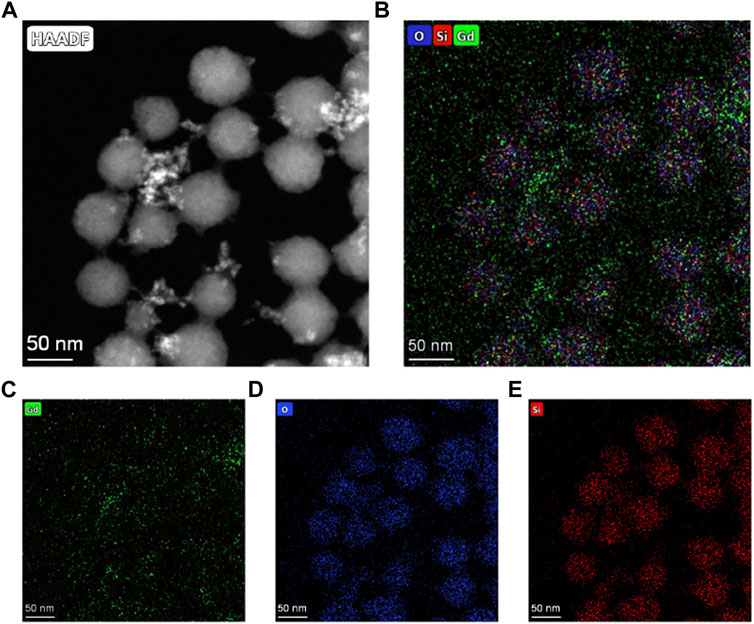
FIGURE 3. (A) Scanning transmission electron microscopy high-angle annular dark-field images of Gd2O3@MSN nanocomposites and (B–E) Elemental mapping images of Gd2O3@MSN nanocomposites, confirming that Gd3+ elements were evenly distributed across MSN structure.
Both surface area and pore distribution are critical for evaluating mesoporous materials; thus, all of the N2 adsorption–desorption isotherms of Gd2O3@MSN using BJH (Figures 4A,B) were evaluated. The samples exhibit typical type IV curves with an evident hysteresis loop, confirming its mesoporous nature (López et al., 2006; Liu et al., 2015; Philippart et al., 2017; Francesca et al., 2018; Naseem et al., 2020; Zhou et al., 2020). The BET test results also suggest that the surface area of the MSN was around 822.96 m2/g, making them much larger than previous versions of similar compounds (Shao et al., 2011). The average pore size of these MSN was 3.49 nm and BJH revealed that they exhibited increased pore volume, 0.72 cm3g−1 compared to seminal MSN materials.
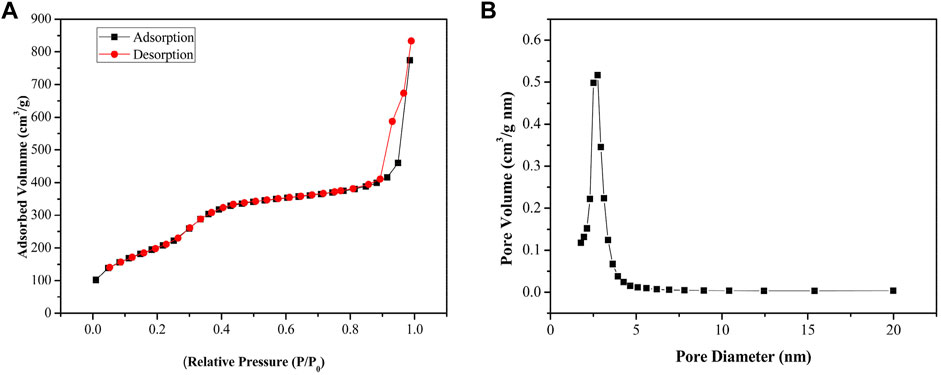
FIGURE 4. (A) Nitrogen adsorption–desorption isotherms. (B) Pore size distribution curves, indicating an average pore size of about 3.49 nm for these Gd2O3@mesoporous silica nanoparticles.
These structures were then validated using an FT-IR spectrometer. The FT-IR spectra for these NPs produced peaks at 801 cm−1 and 455 cm−1 corresponding to Si-OH stretching, a peak at 1,080 cm−1 corresponding to Si-O-Si stretching vibration, and a peak at 3,433 cm−1 corresponding to the absorption bands of the hydroxyl group on MSN surface. These spectra also included a peak at 1,636 cm−1, corresponding to the bending vibration of -OH. Taken together, these patterns were representative of the characteristic absorption peaks of MSN materials (Figure 5) (Ni et al., 2016; He et al., 2019; Jiang et al., 2019; Cai et al., 2020).
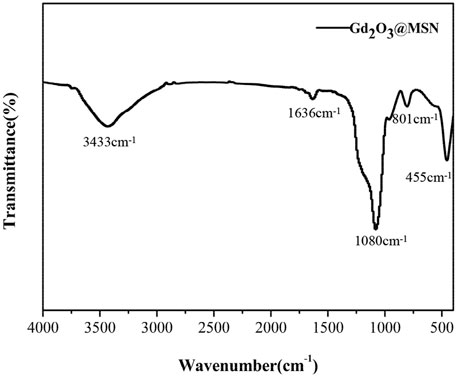
FIGURE 5. Fourier transform infrared refraction spectroscopy of Gd2O3@mesoporous silica nanoparticles.
3.2 T1 Relaxivity and Gd Stability
The ability of pure Gd2O3@MSN was evaluated to enhance T1 contrast using a 0.5 T NMI20 Analyst NMR system. The mean r1 value (0.5 T) for Gd2O3@MSN was 51.85 ± 1.38 mM−1s−1, which was much larger than that of Gd-DTPA (4.62 ± 0.43 mM−1s−1) (Figure 6A). The geometrical confinement of these structures resulted in an approximately 12-fold increase in the Gd2O3@MSN r1 value when compared to that of the commercial Gd-DTPA, which suggested that these MSN could potentially be used as a T1 contrast agent. The stronger contrast effect may be attributed to the tuneable pore structure which facilitates ready access of the water molecules (Parvesh et al., 2012), allowing for multiple water molecules to be coordinated with each Gd centre, decreasing Gd rotation within the framework (Lin et al., 2004; Jiang et al., 2019).
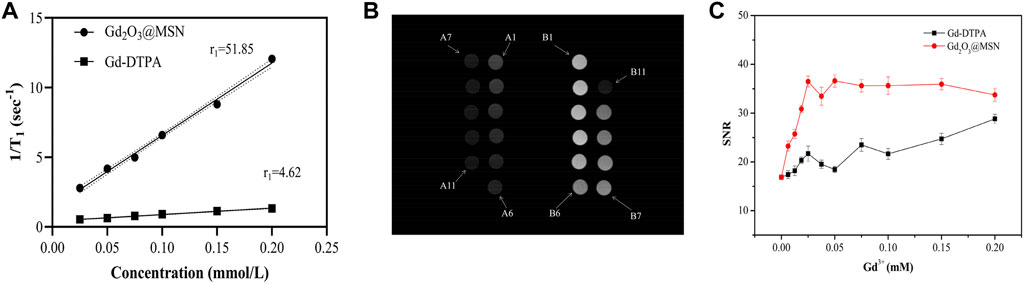
FIGURE 6. Magnetic resonance contrast enhancement analysis of Gd2O3@mesoporous silica nanoparticles (MSN) and Gd-gadolinium diethylene triamine pentaacetate (DTPA). Analysis of the relaxation rate r1 versus gadolinium ion concentration for Gd2O3@MSN and Gd-DTPA in a 0.5T magnetic field (A); T1-weighted phantom images produced from Gd2O3@MSN and Gd-DTPA under a 3T field (B); (C) signal-to-noise ratios of Gd2O3@MSN and Gd-DTPA at varying Gd concentrations.
Figure 6B displayed the in-vitro T1-weighted images obtained using Gd2O3@MSN samples (numbered B1–B11) produced using different Gd concentrations, with these images clearly demonstrating that increasing Gd3+ concentration facilitates increased image brightness. Figure 5B describes similar in-vitro T1-weighted images using Gd-DTPA (numbered A1–A11) at the same concentrations (per Gd atom) as the Gd2O3@MSN images. This data clearly shows a significant increase in brightness in the Gd2O3@MSN images compared to the Gd-DTPA (Supplementary Table S1) images at the same concentration when captured at an MRI intensity of 1972.36 ± 5.57 a. u. (Supplementary Table S2). Next, the signal-to-noise ratio (SNR) was calculated to evaluate the contrast enhancement using finely tuned and broadly representative ROIs within the transverse images. The SNR for each image was then evaluated using the single image method described by the American Association of Physicists in Medicine (AAPM), using the following equation: SNR= (Scentral–Sbackground)/SD (AAPM Quality, 1990). These evaluations revealed that the SNR value of the Gd2O3@MSN images was much higher than that of Gd-DTPA images (Figure 6C).
Given the documented toxicity of free Gd3+ ions, its stability was evaluated within these MSN constructs as the first step for estimating its likely toxicity (Edyta et al., 2019; Lubinda et al., 2018). This was completed by placing Gd2O3@MSN (Gd3+, 100 mg/L) into PBS at different pH values (7.4, 5.5, and 4.5) and incubating these solutions for 48 h at 37°C, with both the pH and temperature designed to emulate various physiological conditions, including normal blood (pH 7.4), endosomes (pH 5.5) and lysosomes (pH 4.5) (Qi et al., 2019; Zhao et al., 2019). ICP-MS was then used to detect any free Gd ions in these solutions (Supplementary Figure S1). These evaluations revealed that there were very few free Gd3+ ions in any of these solutions (<1%) suggesting that Gd2O3@MSN was stable in these simulated in-vitro environments.
3.3 In-Vitro Cytotoxicity Studies
The cytotoxicity of Gd2O3@MSN was estimated using an in-vitro assay of AsPC-1, PaCa-2 and 4T1 cells (Figure 7). CCK-8 assay revealed that there was no significant cytotoxicity following 24 h of exposure to any of the NPs within the described concentration range, suggesting that these NPs exhibit little toxicity toward AsPC-1, PaCa-2 and 4T1 cells (p > 0.05).
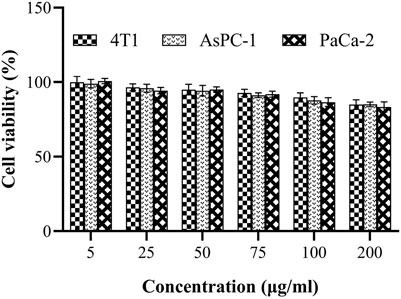
FIGURE 7. Cell viability of AsPC-1, PaCa-2 and 4T1 cells as determined by CCK-8 assay after treatment with Gd2O3@mesoporous silica nanoparticles (5, 25, 50, 100, 150 and 200 μg/ml).
3.4 In-Vivo Safety Evaluation
Healthy SD rats were treated with a high dose (100 mg/kg) of Gd2O3@MSN solution via intravenous (i.v.) injection and then monitored for up to 1 week to assess the in-vivo toxicity of Gd2O3@MSN. Negligible systemic toxicity or side effects were observed when using bodyweight measurement as a comparator (Figure 8) for any of these treatments with all the rats surviving the full-time course. Next, the main organs (heart, liver, spleen, lung, kidney) were harvested from these animals 7 days post-injection and were used to complete the histological assessment of these tissues using haematoxylin and eosin (H&E) staining. H&E evaluations revealed no clear changes in any of these tissues indicating acute (7 days) toxicity in response to Gd2O3@MSN exposure (Figure 9). A mini blood panel was also used to evaluate the potential cytotoxic effects of these NPs with all of the data suggesting the general health of these animals. (Table 2). These biochemical parameters included glutamic aspartate transaminase (AST), alanine aminotransferase (ALT), total bilirubin (T-BIL), blood urea nitrogen (BUN) and creatinine (Cr), all of which had no significant differences when compared with the control group (Gd-DTPA) on day 7 post-injection; thus, confirming that the primary function of both the kidneys and liver was not impaired post Gd2O3@MSN treatment. These results confirm the earlier findings around toxicity and support our hypothesis that this NP is well tolerated in living systems (Bong and Jaeyun, 2019; Yuan et al., 2020).
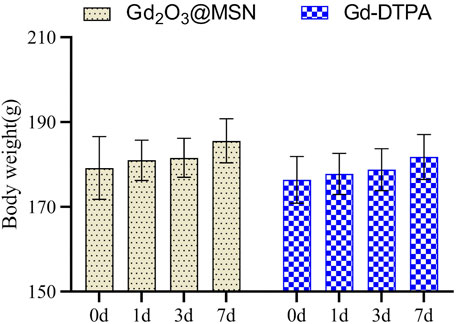
FIGURE 8. Weight changes in rats after 1 week of exposure to Gd2O3@mesoporous silica nanoparticles. Data are expressed as the mean ± standard deviation (n = 5).
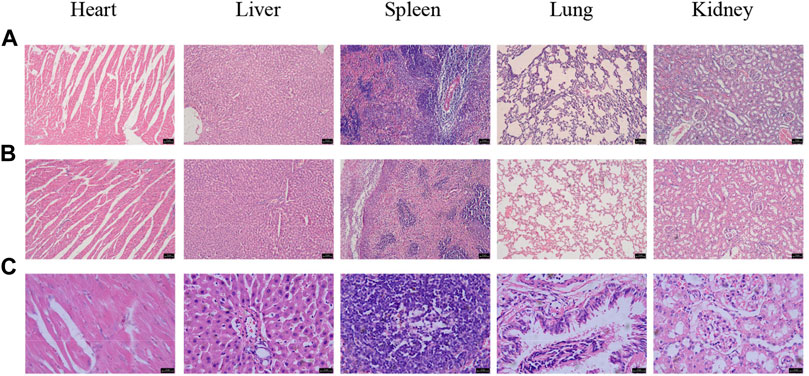
FIGURE 9. Haematoxylin and eosin staining of the heart, liver, spleen, lung and kidneys from (A) Gd- gadolinium diethylene triamine pentaacetate treated Sprague–Dawley rats, scale bar: 75 μm; (B,C) Gd2O3@MSN treated SD rats, scale bar: 75, 25 μm (7 days post-injection).

TABLE 2. Blood chemistry results for Sprague–Dawley rats injected with Gd2O3@mesoporous silica nanoparticles (n = 5).
3.5 In Vivo Magnetic Resonance Imaging Studies
As-prepared contrast materials were injected into healthy SD rats for in-vivo imaging to evaluate Gd2O3@MSN as an in-vivo MRI contrast agent. Previous studies have shown that MSN adsorbs plasma proteins and interacts strongly with the tissue-resident macrophages in the mononuclear phagocyte system (MPS), leading to rapid blood clearance and accumulation in the liver and spleen (Gao et al., 2011; Ekkapongpisit et al., 2012; Li et al., 2016; Wang and Sun, 2020). Given this, we used the likely accumulation of our NPs in the hepatic Kupffer cells to determine our ROIs, focusing on the liver region. T1-weighted images were collected before and 1 h after injection using a 3.0 T clinical MRI scanner. Our evaluations revealed that the liver region exhibited a significantly increased signal in animals treated with Gd-DTPA and Gd2O3@MSN (Figure 10A) when compared to the control. However, a comparison of the Gd-DTPA and Gd2O3@MSN images revealed a distinct increase in the liver signal from the novel contrast agent group, likely as a result of its higher r1 value and increased accumulation in the liver due to its larger size. Next, the signal intensity (SI) was calculated to evaluate the contrast enhancement via the comprehensive evaluation of the ROIs in each of the transverse images (Figure 10B). The ΔSNR value for Gd2O3@MSN was (57.54 ± 6.10)%, which was much higher than that of Gd-DTPA (18.98 ± 1.96)%, confirming the significant improvement in MRI T1 contrast when using Gd2O3@MSN for evaluating the liver.
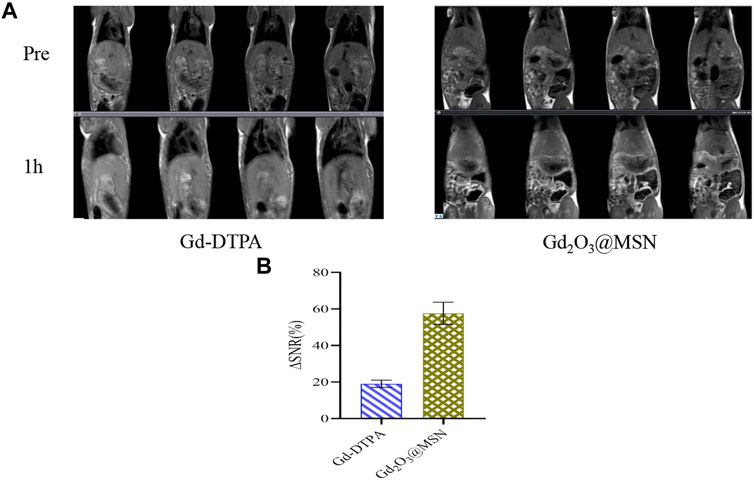
FIGURE 10. (A) T1-weighted in-vivo magnetic resonance imaging of rats (coronal plane) collected before and 1 h after intravenous injection with Gd-gadolinium diethylene triamine pentaacetate (DTPA) or Gd2O3@mesoporous silica nanoparticles (MSN) (at a dose of 0.5 mg Gd per kg of body weight); (B) Signal-to-noise ratios 1 h after intravenous injection of Gd-DTPA or Gd2O3@MSN.
4 Conclusion
To summarize, we successfully designed and fabricated a novel Gd2O3@MSN contrast reagent by one-step synthesis that produced a significant improvement in T1 contrast capacity primarily mediated by the increased geometrical confinement effect of this product. The one-step synthesis method is easy and cost-effective, which would be beneficial for the development of novel MRI contrast agents and for expanding the potential use of high-performance contrast agents for MRI and diagnosis.
Data Availability Statement
The original contributions presented in the study are included in the article/Supplementary Material, further inquiries can be directed to the corresponding authors.
Ethics Statement
The animal study was reviewed and approved by Animal Ethics Committee of Qiqihar Medical University
Author Contributions
Conceptualization and methodology, GL and LH, validation: ZL, JG, and MZ; investigation, ZL; resources:GL and LH; data curation, ZL; writing-original draft preparation, ZL; writing-review and editing, GL and LH; visualization, ZL; project administration and funding acquisition, GL. All authors have read and agreed to the published version of the manuscript.
Fundings
This research was funded by Medical Sciences Project of Qiqihar Academy, grant number QMSI2019L-03, Basic scientific research business expenses of Heilongjiang Provincial Department of Education, grant number 2019-KYYWF-1251.
Conflict of Interest
The authors declare that the research was conducted in the absence of any commercial or financial relationships that could be construed as a potential conflict of interest.
Publisher’s Note
All claims expressed in this article are solely those of the authors and do not necessarily represent those of their affiliated organizations, or those of the publisher, the editors and the reviewers. Any product that may be evaluated in this article, or claim that may be made by its manufacturer, is not guaranteed or endorsed by the publisher.
Acknowledgments
We would like to thank the Qiqihar Medical Center Animal Center for helping with the model rats, the department of MRI at the first Affiliated Hospital of Qiqihar Medical University for MRI equipment support.
Supplementary Material
The Supplementary Material for this article can be found online at: https://www.frontiersin.org/articles/10.3389/fchem.2022.837032/full#supplementary-material
References
AAPM Quality (1990). Assurance Methods and Phantom for Magnetic Resonance Imaging[Z]. AAPM Rep. No 28, 287–295.
Bong, G. C., and Jaeyun, K. (2019). Functional Mesoporous Silica Nanoparticles for Bio-Imaging Applications. Interdiscip. Reviews-Nanomedicine. Nanobiotechnology. 11, e1515. doi:10.1002/wnan.1515
Cai, D. F., Han, C. Y., Liu, C., Ma, X. X., Qian, J. Y., Zhou, J. W., et al. (2020). Chitosan-capped Enzyme-Responsive Hollow Mesoporous Silica Nanoplatforms for colon-specific Drug Delivery. Nanoscale. Res. Lett. 15, 123. doi:10.1186/s11671-020-03351-8
Deng, Z. Y., Qian, Y. F., Yu, Y. Q., Liu, G. H., Hu, J. M., Zhang, J. Y., et al. (2016). Engineering Intracellular Delivery Nanocarriers and Nanoreactors from Oxidation-Responsive Polymersomes via Synchronized Bilayer Cross-Linking and Permeabilizing inside Live Cells. J. Am. Chem. Soc. 138, 10452–10466. doi:10.1021/jacs.6b04115
Edyta, W., Jakub, C., Agnieszka, K., Miroalaw, K., Leon, S., Artur, B., et al. (2019). Toxicity Mechanism of Low Doses of NaGdF4: Yb3+, Er3+ Upconverting Nanoparticles in Activated Macrophage Cell Lines. Biomolecules 9, 14. doi:10.3390/biom9010014
Ekkapongpisit, M., Giovia, A., Follo, C., Caputo, G., and Isidoro, C. (2012). Biocompatibility, Endocytosis, and Intracellular Trafficking of Mesoporous Silica and Polystyrene Nanoparticles in Ovarian Cancer Cells:effects of Size and Surface Charge Groups[J]. Int. J. Nanomedicine. 7, 4147–4158. doi:10.2147/IJN.S33803
Francesca, E. C., Liliana, L., Lukas, G., Goldmann, W. H., and boccaccini, A. R. (2018). Synthesis and Characterization of Silver-Doped Mesoporous Bioactive Glass and its Applications in Conjunction with Electrospinning. Materials (Basel). 11, 692. doi:10.3390/ma11050692
Fraum, T. J., Ludwig, D. R., Bashir, M. R., and Fowler, K. J. (2017). Gadolinium-based Contrast Agents: A Comprehensive Risk Assessment. J. Magn. Reson. Imaging 46, 338–353. doi:10.1002/jmri.25625
Gao, Y., Chen, Y., Ji, X. F., He, X. Y., Yin, Q., Zhang, Z. w., et al. (2011). Controlled Intracellular Release of Doxorubicin in Multidrug-Resistant Cancer Cells by Tuning the Shell-Pore Sizes of Mesoporous Silica Nanoparticles. ACS Nano 5, 9788–9798. doi:10.1021/nn20331010.1021/nn2033105
He, K., Li, J. J., Shen, Y. X., and Yu, Y. Q. (2019). pH-Responsive Polyelectrolyte Coated Gadolinium Oxide-Doped Mesoporous Silica Nanoparticles (Gd2O3@MSNs) for Synergistic Drug Delivery and Magnetic Resonance Imaging Enhancement. J. Mater. Chem. B. 7, 6840–6854. doi:10.1039/c9tb01654f
Hu, X. L., Liu, G., Li, Y., Wang, X. R., and Liu, S. Y. (2015). Cell-penetrating Hyperbranched Polyprodrug Amphiphiles for Synergistic Reductive Milieu-Triggered Drug Release and Enhanced Magnetic Resonance Signals. J. Am. Chem. Soc. 137, 362–368. doi:10.1021/ja5105848
Jiang, w., Fang, H. Y., Liu, F. Q., Zhou, X., Zhao, H. Y., He, X. J., et al. (2019). PEG-coated and Gd-Loaded Fluorescent Silica Nanoparticles for Targeted Prostate Cancer Magnetic Resonance Imaging and Fluorescence Imaging. Int. J. Nanomedicine. 14, 5611–5622. doi:10.2147/IJN.S207098
Koo, H., Huh, M. S., Ju, H. R., Lee, D. E., Sun, I. C., Choi, K., et al. (2011). Nanoprobes for Biomedical Imaging in Living Systems. Nano. Today 6, 204–220. doi:10.1016/j.nantod.2011.02.007
Layek, B., Gidwani, B., Tiwari, S., Joshi, V., Jain, V., and Vyas, A. (2020). Recent Advances in Lipid-Based Nanodrug Delivery Systems in Cancer Therapy. Cpd 26, 3218–3233. doi:10.2174/1381612826666200622133407
Li, X. W., Zhao, W. R., Liu, X. H., Chen, K. Q., Zhu, S. J., Shi, P., et al. (2016). Mesoporous Manganese Silicate Coated Silica Nanoparticles as Multi-Stimuli-Responsive T1-MRI Contrast Agents and Drug Delivery Carriers. Acta Biomater. 30, 378–387. doi:10.1016/j.actbio.2015.11.036
Li, Y. M., Yu, H. S., Qian, Y. F., Hu, J. M., and Liu, S. Y. (2014). Amphiphilic star Copolymer-Based Bimodal Fluorogenic/magnetic Resonance Probes for Concomitant Bacteria Detection and Inhibition. Adv. Mater. 26, 6734–6741. doi:10.1002/adma.201402797
Lin, Y. S., Hung, Y., Su, J. K., Lee, R., Chang, C., Lin, M. L., et al. (2004). Gadolinium(III)-incorporated Nano-Sized Mesoporous Silica as Potential Magnetic Resonance Imaging Contrast Agents. J. Phys. Chem. 108, 15608–15611. doi:10.1021/jp047829a
Liu, S. H., Pavlo, G., Wu, Z. S., Liu, Z. Y., Wei, W., Wagner, M., et al. (2015). Patterning Two-Dimensional Free-Standing Surfaces with Mesoporous Conducting Polymers. Nat. Commun. 6, 8817. doi:10.1038/ncomms9817
López, N. A., Arcos, D., Izquierdo, B. I., Sakamoto, y., Terasaki, O., and Vallet, R. M. (2006). Ordered Mesoporous Bioactive Glasses for Bone Tissue Regeneration. Chem. Mater. 18, 3137–3144. doi:10.1021/cm060488o
Lubinda, M., Steve, T. M., Rosa, B., Nicholas, G. D., Mara, C., and Santin, M. (2018). Gadolinium Tagged Osteoprotegerin-Mimicking Peptide:A Novel Magnetic Resonance Imaging Biospecific Contrast Agent for the Inhibition of Osteoclastogenesis and Osteoclast Activity. Nanomaterials (Basel). 8, 399. doi:10.3390/nano8060399
Mikada, M., Sukhbaatar, A., Miura, Y., Horie, S., Sakamoto, M., and Mori, S. (2017). Evaluation of the Enhanced Permeability and Retention Effect in the Early Stages of Lymph Node Metastasis. Cancer Sci. 108, 846–852. doi:10.1111/cas.13206
Naseem, F., Zhi, Y., Farrukh, M. A., Hussain, F., and Yin, Z. Y. (2020). Mesoporous ZnAl2Si10O24 Nanofertilizers Enable High Yield of Oryza Sativa L. Sci. Rep. 10, 10841. doi:10.1038/s41598-020-67611-4
Ni, D., Bu, W., Ehlerding, E. B., Cai, W., and Shi, J. (2017). Engineering of Inorganic Nanoparticles as Magnetic Resonance Imaging Contrast Agents. Chem. Soc. Rev. 46, 7438–7468. doi:10.1039/c7cs00316a
Ni, K., Zhao, Z. H., Zhang, Z. J., Zhou, Z. J., Yang, L., Wang, L. R., et al. (2016). Geometrically Confined Ultrasmall Gadolinium Oxide Nanoparticles Boost the T1 Contrast Ability. Nanoscale 8, 3768–3674. doi:10.1039/c5nr08402d
O’Connell, C. L., Nooney, R., and McDonagh, C. (2017). Cyanine5-doped Silica Nanoparticles as Ultra-bright Immunospecific Labels for Model Circulating Tumour Cells in Flow Cytometry and Microscopy. Biosens. Bioelectron. 91, 190–198. doi:10.1016/j.bios.2016.12.023
Parvesh, S., Niclas, E. B., Glenn, A. W., Han-Byul, S., Zhou, G. Y., Nobutaka, I., et al. (2012). Gadolinium-Doped Silica Nanoparticles Encapsulating Indocyanine Green for Near Infrared and Magnetic Resonance Imaging. Small 8, 2856–2868. doi:10.1002/smll.201200258
Philippart, A., Gómez, C. N., Arcos, D., Salinas, J., Boccardi, E., Vallet, R. M., et al. (2017). Novel Ion-Doped Mesoporous Glasses for Bone Tissue engineering:Study of Their Structural Characteristics Influenced by the Presence of Phosphorous Oxide. J. Non. Cryst. Sol. 455, 90–97. doi:10.1016/j.jnoncrysol.2016.10.031
Phillps, M. A., Gran, M. L., and Peppas, N. A. (2010). Targeted Nanodelivery of Drugs and Diagnostics. Nano. Today 5, 143–159. doi:10.1016/j.nantod.2010.03.003
Qi, Q. K., Chi, W. J., Li, Y. Y., Qian, Q. L., Chen, J., Miao, L., et al. (2019). A H-Bond Strategy to Develop Acid-Resistant Photoswitchable Rhodamine Spirolactams for Super-resolution Single-Molecule Localization Microscopy. Chem. Sci. 10, 4914–4922. doi:10.1039/c9sc01284b
Shao, Y. Z., Liu, L. Z., Song, S. Q., Cao, R. H., Liu, H., Cui, C. Y., et al. (2011). A Novel One-step Synthesis of Gd3+-Incorporated Mesoporous SiO2 Nanoparticles for Use as an Efficient MRI Contrast Agent. Contrast. Media. Mol. Imaging 6, 110–118. doi:10.1002/cmmi.412
Shen, Z., Wu, A., and Chen, X. (2017). Iron Oxide Nanoparticle Based Contrast Agents for Magnetic Resonance Imaging. Mol. Pharmaceutics 14, 1352–1364. doi:10.1021/acs.molpharmaceut.6b00839
Shin, T.-H., Choi, Y., Kim, S., and Cheon, J. (2015). Recent Advances in Magnetic Nanoparticle-Based Multi-Modal Imaging. Chem. Soc. Rev. 44, 4501–4516. doi:10.1039/c4cs00345d
Taylor, K. M., Kim, J. S., Rieter, W. J., An, H. Y., Lin, W. L., and Lin, W. B. (2008). Mesoporous Silica Nanospheres as Highly Efficient MRI Contrastagents. J. Am. Chem. Soc. 130, 2154–2155. doi:10.1021/ja710193c
Vikas, G., Fernando, C., Frank, G. S., Emanuel, K., and Scott, B. R. (2017). Gadolinium Deposition in the Brain:summary of Evidence and Recommendations. Lancet Neurol. 16, 564–570. doi:10.1016/S1474-4422(17)30158-8
Wang, L. Q., and Sun, X. F. (2020). Mesoporous Silica Hybridized with Gadolinium(III) Nanoplatform for Targeted Magnetic Imaging - Guided Photothermal Breast Cancer Therapy. Dose Response 18, 1559325820902314. doi:10.1177/1559325820902314
Xia, N., Deng, D. H., Mu, X. S., Liu, A., Xie, J. X., Zhou, D. D., et al. (2019). Colorimetric Immunoassays Based on Pyrroloquinoline Quinone-Catalyzed Generation of Fe(II)-ferrozine with Tris(2-Carboxyethyl)phosphine as the Reducing Reagent. Sensors Actuators B: Chem. 306, 127571. doi:10.1016/j.snb.2019.127571
Yuan, D. H., Connor, M. E., and Jason, J. D. (2020). Mesoporous Silica Nanoparticles in Bioimaging. Materials (Basel) 13, 3795. doi:10.3390/ma13173795
Zhao, J. W., Yang, H., Li, J. l., Wang, Y. J., and Wang, X. (2019). Fabrication of pH-Responsive PLGA(UCNPs/DOX) Nanocapsules with Upconversion Luminescence for Drug Delivery. Sci. Rep. 7, 18014. doi:10.1038/s41598-017-16948-4
Keywords: gadolinium oxide, mesoporous silica nanoparticle, magnetic resonance imaging, cytotoxicity, contrast agent
Citation: Li Z, Guo J, Zhang M, Li G and Hao L (2022) Gadolinium-Coated Mesoporous Silica Nanoparticle for Magnetic Resonance Imaging. Front. Chem. 10:837032. doi: 10.3389/fchem.2022.837032
Received: 16 December 2021; Accepted: 24 January 2022;
Published: 15 February 2022.
Edited by:
Wansong Chen, Central South University, ChinaCopyright © 2022 Li, Guo, Zhang, Li and Hao. This is an open-access article distributed under the terms of the Creative Commons Attribution License (CC BY). The use, distribution or reproduction in other forums is permitted, provided the original author(s) and the copyright owner(s) are credited and that the original publication in this journal is cited, in accordance with accepted academic practice. No use, distribution or reproduction is permitted which does not comply with these terms.
*Correspondence: Liguo Hao, haoliguo@qmu.edu.cn; Guohua Li, liguohua@qmu.edu.cn