Intracellular delivery of therapeutic proteins. New advancements and future directions
- Department of Chemistry, Materials and Chemical Engineering “G. Natta”, Politecnico di Milano, Milan, Italy
Achieving the full potential of therapeutic proteins to access and target intracellular receptors will have enormous benefits in advancing human health and fighting disease. Existing strategies for intracellular protein delivery, such as chemical modification and nanocarrier-based protein delivery approaches, have shown promise but with limited efficiency and safety concerns. The development of more effective and versatile delivery tools is crucial for the safe and effective use of protein drugs. Nanosystems that can trigger endocytosis and endosomal disruption, or directly deliver proteins into the cytosol, are essential for successful therapeutic effects. This article aims to provide a brief overview of the current methods for intracellular protein delivery to mammalian cells, highlighting current challenges, new developments, and future research opportunities.
1 Introduction
In the last years, protein-based therapeutics have gained an increasing interest in all areas of medicine (Lv et al., 2019; Zhang S. et al., 2020), attracting the attention of the major pharmaceutical industries (Ren et al., 2022; Pandya and Patravale, 2021), due to their remarkable potentials for treatment, diagnosis, and even prevention (Pakulska et al., 2016; Sá et al., 2021; Tan et al., 2021) of several human pathologies (Liu et al., 2022). Protein therapeutics show notable pharmacological efficacy (Pakulska et al., 2016; Liu X. et al., 2019) combined with high therapeutic potency and selectivity with respect to traditional low molecular weight drugs (Cheng, 2021). Compared to small synthetic molecules (Mitragotri et al., 2014; Slastnikova et al., 2018), proteins offer the advantage to be active and effective at lower concentration with high substrate specificity, favoring minimal adverse effects (Leader et al., 2008) and reduced risks of off targets (Hou et al., 2016; Gao et al., 2018).
Previous studies show that most attractive targets are typically located inside the cell (Postupalenko et al., 2015; Tan et al., 2022), thus, in order to exploit full potential of protein-based therapeutics, intracellular protein delivery is fundamental to target intracellular biomolecules (Gu et al., 2011; Mitragotri et al., 2014; Scaletti et al., 2018; Liu X. et al., 2019; Lv et al., 2019). This represent one of the major challenges to overcome since proteins are large and complex biomolecules (Lee et al., 2019; Goswami et al., 2020; Raman et al., 2021; Davis et al., 2022), with markedly hydrophilic features (Lv et al., 2020), resulting in poor cell membrane permeability (Postupalenko et al., 2015; Wang and Yu, 2020). Hence, the not spontaneous crossing of the anionic-hydrophobic cell membrane (Mulgrew-Nesbitt et al., 2006) will limits the currently marketed protein drugs to extracellular targets (Marschall et al., 2014; Mitragotri et al., 2014; Slastnikova et al., 2018; Gaston et al., 2019; Qin et al., 2019).
The objective of this concise review is to outline the existing techniques for delivering proteins inside mammalian cells, aiming to highlight the current challenges, recent advancements, and potential research prospects in this field.
2 Developments and challenges in intracellular protein delivery
Different exogenous proteins have been recently explored for intracellular delivery, to modulate cell function and fate, by targeting disease-relevant intracellular receptors. Various strategies for intracellular targeting of antibodies, their fragments, or antibody-like molecules have been extensively reported in other works (Stewart et al., 2016; Slastnikova et al., 2018; Xie et al., 2020). Due to their remarkable specificity and affinity for a target molecule, antibodies are widely used for inhibiting specific activity and for diagnostics, as well as for basic experimental tools, given their role in unveiling cell signaling pathways and diseases mechanisms. Moreover, other therapeutic proteins have been investigated for targeting intracellular sites, including systems for genome editing, induction of apoptosis or toxicity, and blocking specific protein expression, as summarized in Table 1.
The clinical applications of these protein drugs face several limitations in terms of delivery efficacy, stability, and final intracellular activity. Additional obstacles, such as fast enzymatic degradation in the bloodstream (Yan et al., 2022) and possible immune system response [common to therapeutic proteins for extracellular delivery (Parodi et al., 2017; Moncalvo et al., 2020)], must be considered.
Although delivery vehicles can help transporting proteins across cell membranes (Luther et al., 2020), the limited number of binding sites on protein surface represents a key issue that hinders the efficient transport of the cargo proteins by the appropriate carrier (Lv et al., 2020). In fact, the surface of proteins is notoriously heterogeneous, being covered by cationic, anionic, and hydrophobic groups. For this reason, carriers often rely on covalent conjugation of functional molecules (Loibl and Gianni, 2017), although critical disadvantages of such systems include the limited availability of residues for conjugation, potential effects on protein folding and function (Weiner, 2015) [given their sensitivity to chemical modifications (Zhang et al., 2018)], and complex workflow steps. Moreover, cellular internalization often brings the nanocarrier to the cytoplasm via endosomes, by means of naturally occurring endocytosis processes, such as clathrin-mediated endocytosis (Kaksonen et al., 2006), caveolae-mediated endocytosis (Nabi and Le, 2003) or micropinocytosis (Kerr and Teasdale, 2009). Endosomes will ultimately be transformed into lysosomes, with a consequent increase of the environment acidity and the secretion of various proteases (Niamsuphap et al., 2020), causing protein degradation. Nonetheless, endosomal discharge is generally an inefficient process, with only ∼1% of the total cargo being released intact into the cytoplasm excluding deterioration or expulsion by exocytosis (Stewart et al., 2016). Non-specific clearance by the reticuloendothelial system (RES) after systemic administration of protein-loaded carriers generally causes a significant decrease of the delivery efficiency into the target tissues. To address this issue, strategies as a transient stealth coating of liver reticuloendothelial cells by two-arm-PEG-oligopeptide may be effective in preventing the clearance of nonviral and viral nanovectors by the liver sinusoidal endothelium (Dirisala et al., 2020).
Therefore, the development of efficient and versatile delivery strategies is crucial for an effective use of protein drugs (Feng et al., 2022). They need to reach cytoplasmic targets safely (Wang and Yu, 2020) by encapsulating the desired cargo into cell-degradable nanocarriers (Tsao et al., 2020; Liu et al., 2022), able to trigger endocytosis and endosomal disruption (Zhang et al., 2018), or capable to directly deliver proteins into the cytosol (Sun et al., 2022).
3 Intracellular protein delivery techniques: An overview
During the past decade numerous prominent techniques have been proposed for intracellular delivery of proteins (Fu et al., 2014; Bruce and McNaughton, 2017; Ray et al., 2017; Tian et al., 2022), involving physical methods to cross cell membrane, protein chemical modification and protein transport through carriers (Scaletti et al., 2018; Lee et al., 2019; Goswami et al., 2020) or a combination of the three types. Some examples of the strategies proposed in the next sections are depicted in Figure 1.
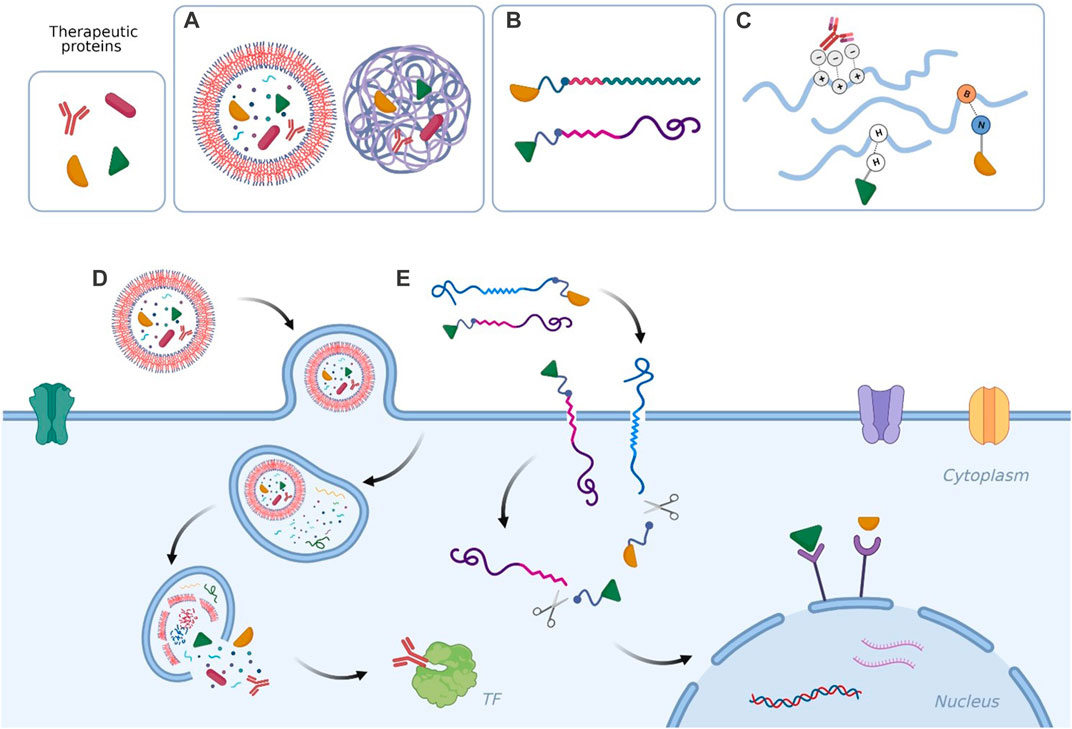
FIGURE 1. Examples of (A) therapeutic proteins encapsulated in polymersomes and in polymeric nanoparticles; (B) therapeutic proteins conjugated to amphiphilic polymers and to cell-permeable peptides; (C) therapeutic proteins forming non-covalent complexes with polymers; (D) nanosystem delivered across the cell membrane via endocytosis to release the therapeutic cargo in proximity of a cytosolic target; (E) protein-polymer conjugate and protein-peptide conjugate entering the cell via direct translocation/transduction and delivering the therapeutic material to nucleus receptors. Created with BioRender.com.
In most cases, model proteins have been tested rather than more expensive therapeutic proteins, which are often difficult to track both in vitro and in vivo. Fluorescent albumin and IgG antibody (Tian et al., 2013; Sarker et al., 2014; Liew et al., 2020; Barrios et al., 2022; Davis et al., 2022), (enhanced) green fluorescent protein (GFP) (Fuchs and Raines, 2007; Kaczmarczyk et al., 2011; Sarker et al., 2014; Zuris et al., 2015; Kube et al., 2017; Liew et al., 2020; Davis et al., 2022), streptavidin (Shi et al., 2017; Davis et al., 2022), horseradish peroxidase (DePorter and McNaughton, 2014), lysozyme (Tian et al., 2013), and ovalbumin (Goswami et al., 2023) are among the typical model proteins used.
3.1 Physical membrane crossing methods
Most of the physical approaches for overcoming cell membrane deal with chemical (Stewart et al., 2018) membrane disruption (Mukherjee et al., 2018) or perforation (Chen N. et al., 2022). Although membrane perforation with electroporation (Mukherjee et al., 2018) and microinjection (Keppeke et al., 2015; Chen N. et al., 2022) or sonoporation (Togtema et al., 2012) is the most straightforward method for cytosolic delivery, these strategies are highly efficient in in vitro studies (Tan et al., 2022), but generally toxic, only suitable for introducing a small number of specific proteins into incubated cells and can hardly be used in vivo.
3.2 Chemical modifications of proteins
Protein modification strategy directly features protein with membrane-permeable ligands, such as cell penetrating peptides (Dixon et al., 2016; Su et al., 2018), chimeric peptides (Yu et al., 2021), cationic peptides or polymers (Su et al., 2018), amphiphilic polymers (Liu X. et al., 2019) and protein transduction domains (Caffrey et al., 2016). Alternatively, the chemical alteration consists in supercharging the protein with cationic groups (Horn and Obermeyer, 2021). The biomodification success depends on the availability of reactive protein handles, located on their surface as free amino acid sides, including amine, hydroxy and thiol groups, or functional moieties present at the protein termini (Stephanopoulos and Francis, 2011). There are many covalent methods available for the modification of protein reactive groups including click chemistry, oxime/hydrazone chemistry (Lutz and Börner, 2008), and strategies such as grafting-to, grafting-from and grafting-through for bioconjugation of proteins with polymers (Stevens et al., 2021).
The amended proteins are capable of entering the cells via cellular membrane transduction and translocation (Horn and Obermeyer, 2021) or through endocytosis, achieving high cytosolic delivery (Posey and Tew, 2018) by increased membrane affinity. Sometime covalent modification of proteins is also applied with anionic species, such as carboxylic acid (Wang and Yu, 2020), boronic acid (Liu X. et al., 2019), anionic peptides and polymers (Zelikin et al., 2016), or nucleic acids (Eltoukhy et al., 2014) to strengthen their negative charge intensity, and thus increase their binding affinity with suitable positively charged carriers that enhance endocytosis (Lv et al., 2020). However, covalent modifications often result in a distribution of products with different degrees of modification, owing to chemically identical active sites distributed on the protein surface (Horn and Obermeyer, 2021). Protein alteration can be designed to be reversible, via moieties which can be cleaved by intracellular stimuli such as reduction (Qian et al., 2018), reactive oxygen species (ROS) (Liu X. et al., 2019), enzyme (Chang et al., 2019) and endo/lysosomal acidity (Maier and Wagner, 2012), however covalent modifications may alter protein structures and related biofunctions (Zhou et al., 2019; Tan et al., 2022). Moreover, the technique requires complex synthesis and purification procedures which may impede the potential clinical translation (Frokjaer and Otzen, 2005; Stevens et al., 2021). A meaningful example of protein alteration for cytosolic delivery involves charge-conversional modification of cationic lysine surface moieties by cyclic anhydrides (Obermeyer et al., 2016; Zhang M. et al., 2020; Tao et al., 2020), which is pH-reversible at late endosomal pH. For instance, IgG was modified with citraconic anhydride to encapsulate it in pH-sensitive polyion micelles, capable of transferring active IgG to the nuclear envelope (Kim et al., 2016). Esterification of carboxylic acid groups of aspartate and glutamate simultaneously decrease negative charge and increase hydrophobicity, promoting direct protein translocation across the cell membrane (Sangsuwan et al., 2019).
Stable and simultaneously reversible conjugation is critical to translocate proteins across a cellular membrane and release them without losing activity (Dutta et al., 2021). Liu B. et al. (2019) developed a click chemistry approach for generating functional polymer–protein conjugate as nanoassemblies of different sizes and isoelectric points, which release in response to three different stimuli: presence of ROS, reducing environment, and pH variations. Arylboronic acid was employed for lysines modification, given the possibility of inserting a stimuli-responsive linker in the polymer-protein conjugate, required for a residue-free release (Stephanopoulos and Francis, 2011). They successfully delivered ribonuclease A (RNaseA) via endosomal escape, employing hydrogen peroxide (H2O2) as the stimulus to reverse the bioconjugation. Similarly, Dutta et al. (2021) designed a self-immolative polymer presenting activated carbonate moieties for covalent self-assembly with the lysines displayed on antibodies surface. The reactive side-chain functionalities were responsive to redox stimuli, and the encapsulated antibodies were released preserving their biological activity. However, slow macromolecular reaction kinetics due to the high acid dissociation constant (pKa) of lysine amines (Koniev and Wagner, 2015), incomplete reactivity of activated carbonate groups with lysines (Dutta et al., 2017), and competitive hydrolytic degradation of polymer, are some of the major obstacles for large biomacromolecules conjugation such as antibodies (Dutta et al., 2021). Considerable attention has been given to enhancing the endosomal escape ability of nanocarriers by incorporating pH-buffering (Lee et al., 2021), membrane-disturbing (Han et al., 2021) or fusogenic (Nishimura et al., 2014) materials. pH-responsive polymeric micelles were designed to promote electrostatic and covalent interactions with anti-nuclear pore complex antibodies (Chen P. et al., 2022). This design reached selective delivery into the cytosol and subsequent nucleus targeting was achieved in cancer cells, rather than non-cancerous cells, both in vitro and in vivo.
3.3 Non-covalent assembly of proteins and carriers
Alternatively, proteins could be transported by carriers through physical encapsulation or complexation. The protein cargoes can be loaded into the inner aqueous/hydrophilic cavities or pores (Tang et al., 2017; Wang and Yu, 2020) of synthetic nanocarriers (Qin et al., 2019), such as liposomes (Wang et al., 2016), polymers (Zhou et al., 2019), polymersomes (Jiang et al., 2018), organic or inorganic nanoparticles (Leader et al., 2008; Zelikin et al., 2016; Lee et al., 2019; Zhang S. et al., 2020), and nanogels (Dutta et al., 2017). These nanomaterials allow intracellular delivery of native proteins without any chemical modification, preventing denaturation (Dutta et al., 2017). This approach is generally suitable for in vivo applications (Lv et al., 2020), although it often requires complex syntheses and purification processes with low protein loading efficiency (Liu et al., 2022). On the other hand, protein-based nanocomplexes can be formed via non-covalent interactions with polymers, functionalized nanoparticles, peptides, and lipids. Amino acid residues may interact via salt bridge, boronate-nitrogen (Liu X. et al., 2019; Liu et al., 2022) or metal-nitrogen (Ren et al., 2022) coordination interactions, electrostatic forces (Rui et al., 2019), inter-macromolecular ionic, hydrophobic (He et al., 2019), and hydrogen-bond interactions (Lv et al., 2020). Such assemblies should provide stability during cell membrane penetration and protein release (Yu et al., 2018), via reversible binding (Stevens et al., 2021). They are obtained via simple mixing under mild aqueous conditions, avoiding complex purification steps, without altering the proteins native functions (Posey and Tew, 2018; Lv et al., 2019; Lv et al., 2020; Pasut, 2014). While chemical modification is often limited by the vast heterogeneity in composition, structure, and stability of proteins, non-covalent strategies can be applied to a wide variety of protein cargoes (Posey and Tew, 2018).
In the last years, different nanocomplexes formed via simple self-assembly have been developed (Liu X. et al., 2019; Lv et al., 2020; Wang and Yu, 2020). Hyperbranched polymer with phenylboronic acid (PBA) was developed to coordinate with protein cargoes (Liu et al., 2022), and degrade by over-produced H2O2 in cancer cells, releasing the proteins (BSA and a monoclonal antibody). Following a similar idea, boronated polymers formed a complex with proteins via nitrogen-boronate coordination and ionic interaction (Yan et al., 2022). Promising cytosolic delivery of cargo proteins and peptides was achieved with maintained bioactivity (Liu X. et al., 2019; Lv et al., 2020). Relying on the same principles, guanidyl groups can strongly bind the residual moieties of protein by a combination of salt bridge and hydrogen bonding interactions. When grafting guanidyl ligands onto nanoparticles or polymers at a high ligand density, the multivalent effect of guanidyl groups allows efficient protein binding (Hatano et al., 2016) and endocytosis (Stanzl et al., 2013; McKinlay et al., 2016). Lv et al. (2020) synthesized guanidyl-grafted polyethylenimine (PEI) to form positively charged nanoparticles with BSA, for an efficient cell membrane penetration. Protein delivery systems poorly performing in serum-containing media were improved by introducing carbamoylated guanidine-containing polymers (Barrios et al., 2022), by chemical modification with fluorous ligands (Zhang et al., 2018) and zwitterionic moieties (Wu et al., 2019), thus decreasing the positive charge density of the nanocomplex (Rui et al., 2019). A rational guanidine modification approach also enhanced the efficiency of proteins delivery in serum-containing media (Li et al., 2015; Keller et al., 2016; Davis et al., 2022). Tan et al. (2022) proposed boronate-decorated poly-L-lysine (PLL) to efficiently deliver cargo proteins into living cells. Positively charged PLL spontaneously form complexes with negatively charged proteins (Abbas et al., 2017). These nanoparticles can release proteins by intracellular ROS after internalization, with maintained activity and minimal toxicity. Amphipathic poly-b-peptides (Pbps), with designable structures, controllable molecular weights, and proteolysis resistant properties, were also investigated for protein delivery (Ren et al., 2022). Pbps amphipathic and positively charged structures promote non-covalent interactions with proteins and membrane disruption (Tezgel et al., 2017), showing successful delivery of EGFP into osteosarcoma cells.
4 Discussion
Significant progress has been made in the field of intracellular delivery in recent years, however the clinical applications of protein drugs are still limited by their real delivery efficacy, stability, and intracellular activity. Therefore, research is moving in various directions with the aim of identifying more appropriate delivery tools. The delivery of proteins into cells is challenging due to two major requirements: efficient uptake and rapid cytosolic delivery without being trapped in the endosomes. Many research efforts have been made regarding protein conjugation with cell-penetrating peptides, and more recently with multifunctional chimeric peptides, which can be designed to accomplish different tasks during cellular uptake and endosomal escape. Other methods for the delivery of purified proteins include protein chemical modification and resurfacing approaches. These methods often need to overcome the limits of toxicity and possible immune activation. Nanocarrier-based protein delivery approaches, such as liposomes, polymer-based nanocarriers, and nanoparticles, are attractive due to the tunable properties of the nanomaterials. It is important to consider additional obstacles such as the rapid enzymatic degradation of therapeutic proteins in the bloodstream and potential immune system responses (Moncalvo et al., 2020). Meanwhile, a significant effort has been dedicated to the design of engineered proteins that can be used to modulate intracellular targets (Miersch and Sidhu, 2016). Co-delivery of protein and nucleic acids has also been examined in the context of targeted genomic editing (Bruce and McNaughton, 2017). Moreover, new intracellular targets within subcellular compartments may be identified for a therapeutic use (Fasciani et al., 2022). Delivery of transcription factors also holds the potential to revolutionize the biomedical field (Ulasov et al., 2018), although the major challenge lies in the delivery process, as it requires proteins transport not only across the cell membrane and the endosome, but also into the nucleus, which represents an additional barrier to overcome.
The field of intracellular protein delivery is still a relatively young area of research and further advancements in this field will require the integration of chemistry, materials science, formulation science, nanomedicine, and biomedical engineering. Enabling therapeutic proteins to access and target intracellular receptors has enormous potential for improving human health and fighting diseases, as well as for gaining knowledge in this significant area of research.
Author contributions
All authors listed have made a substantial, direct, and intellectual contribution to the work and approved it for publication.
Funding
This research was funded by Regione Lombardia (POR FESR 2014–2020) within the framework of the NEWMED project (ID 1175999).
Conflict of interest
The authors declare that the research was conducted in the absence of any commercial or financial relationships that could be construed as a potential conflict of interest.
Publisher’s note
All claims expressed in this article are solely those of the authors and do not necessarily represent those of their affiliated organizations, or those of the publisher, the editors and the reviewers. Any product that may be evaluated in this article, or claim that may be made by its manufacturer, is not guaranteed or endorsed by the publisher.
References
Abbas, M., Zou, Q., Li, S., and Yan, X. (2017). Self-assembled peptide- and protein-based nanomaterials for antitumor photodynamic and photothermal therapy. Adv. Mater. 29, 1605021. doi:10.1002/adma.201605021
Barrios, A., Estrada, M., and Moon, J. H. (2022). Carbamoylated guanidine-containing polymers for non-covalent functional protein delivery in serum-containing media. Angew. Chem. - Int. Ed. 61, e202116722. doi:10.1002/anie.202116722
Bruce, V. J., and McNaughton, B. R. (2017). Inside job: Methods for delivering proteins to the interior of mammalian cells. Cell. Chem. Biol. 24, 924–934. doi:10.1016/j.chembiol.2017.06.014
Caffrey, L. M., Deronde, B. M., Minter, L. M., and Tew, G. N. (2016). Mapping optimal charge density and length of ROMP-based PTDMs for siRNA internalization. Biomacromolecules 17, 3205–3212. doi:10.1021/acs.biomac.6b00900
Chang, J., Cai, W., Liang, C., Tang, Q., Chen, X., Jiang, Y., et al. (2019). Enzyme-instructed activation of pro-protein therapeutics in vivo. J. Am. Chem. Soc. 141, 18136–18141. doi:10.1021/jacs.9b08669
Chen, N., He, Y., Zang, M., Zhang, Y., Lu, H., Zhao, Q., et al. (2022). Approaches and materials for endocytosis-independent intracellular delivery of proteins. Biomaterials 286, 121567. doi:10.1016/j.biomaterials.2022.121567
Chen, P., Yang, W., Hong, T., Miyazaki, T., Dirisala, A., Kataoka, K., et al. (2022). Nanocarriers escaping from hyperacidified endo/lysosomes in cancer cells allow tumor-targeted intracellular delivery of antibodies to therapeutically inhibit c-MYC. Biomaterials 288, 121748. doi:10.1016/j.biomaterials.2022.121748
Cheng, Y. (2021). Design of polymers for intracellular protein and peptide delivery. Chin. J. Chem. 39, 1443–1449. doi:10.1002/cjoc.202000655
Cronican, J. J., Thompson, D. B., Beier, K. T., McNaughton, B. R., Cepko, C. L., and Liu, D. R. (2010). Potent delivery of functional proteins into mammalian cells in vitro and in vivo using a supercharged protein. ACS Chem. Biol. 5, 747–752. doi:10.1021/cb1001153
Davis, H. C., Posey, N. D., and Tew, G. N. (2022). Protein binding and release by polymeric cell-penetrating peptide mimics. Biomacromolecules 23, 57–66. doi:10.1021/acs.biomac.1c00929
DePorter, S. M., and McNaughton, B. R. (2014). Engineered M13 bacteriophage nanocarriers for intracellular delivery of exogenous proteins to human prostate cancer cells. Bioconjug Chem. 25, 1620–1625. doi:10.1021/bc500339k
Dirisala, A., Uchida, S., Toh, K., Li, J., Osawa, S., Tockary, T. A., et al. (2020). Transient stealth coating of liver sinusoidal wall by anchoring two-armed PEG for retargeting nanomedicines. Sci. Adv. 6, eabb8133. doi:10.1126/sciadv.abb8133
Dixon, J. E., Osman, G., Morris, G. E., Markides, H., Rotherham, M., Bayoussef, Z., et al. (2016). Highly efficient delivery of functional cargoes by the synergistic effect of GAG binding motifs and cell-penetrating peptides. Proc. Natl. Acad. Sci. U. S. A. 113, E291–E299. doi:10.1073/pnas.1518634113
Dutta, K., Hu, D., Zhao, B., Ribbe, A. E., Zhuang, J., and Thayumanavan, S. (2017). Templated self-assembly of a covalent polymer network for intracellular protein delivery and traceless release. J. Am. Chem. Soc. 139, 5676–5679. doi:10.1021/jacs.7b01214
Dutta, K., Kanjilal, P., Das, R., and Thayumanavan, S. (2021). Synergistic interplay of covalent and non-covalent interactions in reactive polymer nanoassembly facilitates intracellular delivery of antibodies. Angew. Chem. - Int. Ed. 60, 1821–1830. doi:10.1002/anie.202010412
Eltoukhy, A. A., Chen, D., Veiseh, O., Pelet, J. M., Yin, H., Dong, Y., et al. (2014). Nucleic acid-mediated intracellular protein delivery by lipid-like nanoparticles. Biomaterials 35, 6454–6461. doi:10.1016/j.biomaterials.2014.04.014
Fasciani, I., Carli, M., Petragnano, F., Colaianni, F., Aloisi, G., Maggio, R., et al. (2022). GPCRs in intracellular compartments: New targets for drug discovery. Biomolecules 12, 1343. doi:10.3390/biom12101343
Feng, Y., Guo, Z., Chen, J., Zhang, S., Wu, J., Tian, H., et al. (2022). Cationic polymer synergizing with a disulfide-containing enhancer achieved efficient nucleic acid and protein delivery. Biomater. Sci. 10, 6230–6243. doi:10.1039/d2bm01211a
Frokjaer, S., and Otzen, D. E. (2005). Protein drug stability: a formulation challenge. Nat. Rev. Drug Discov. 4, 298–306. doi:10.1038/nrd1695
Fu, A., Tang, R., Hardie, J., Farkas, M. E., and Rotello, V. M. (2014). Promises and pitfalls of intracellular delivery of proteins. Bioconjug Chem. 25, 1602–1608. doi:10.1021/bc500320j
Fuchs, S. M., and Raines, R. T. (2007). Arginine grafting to endow cell permeability. ACS Chem. Biol. 2, 167–170. doi:10.1021/cb600429k
Gao, X., Tao, Y., Lamas, V., Huang, M., Yeh, W. H., Pan, B., et al. (2018). Treatment of autosomal dominant hearing loss by in vivo delivery of genome editing agents. Nature 553, 217–221. doi:10.1038/nature25164
Gaston, J., Maestrali, N., Lalle, G., Gagnaire, M., Masiero, A., Dumas, B., et al. (2019). Intracellular delivery of therapeutic antibodies into specific cells using antibody-peptide fusions. Sci. Rep. 9, 18688. doi:10.1038/s41598-019-55091-0
Goswami, R., Jeon, T., Nagaraj, H., Zhai, S., and Rotello, V. M. (2020). Accessing intracellular targets through nanocarrier-mediated cytosolic protein delivery. Trends Pharmacol. Sci. 41, 743–754. doi:10.1016/j.tips.2020.08.005
Goswami, R., Lehot, V., Çiçek, Y. A., Nagaraj, H., Jeon, T., Nguyen, T., et al. (2023). Direct cytosolic delivery of citraconylated proteins. Pharmaceutics 15, 218. doi:10.3390/pharmaceutics15010218
Gu, Z., Biswas, A., Zhao, M., and Tang, Y. (2011). Tailoring nanocarriers for intracellular protein delivery. Chem. Soc. Rev. 40, 3638–3655. doi:10.1039/c0cs00227e
Han, X., Zhang, H., Butowska, K., Swingle, K. L., Alameh, M. G., Weissman, D., et al. (2021). An ionizable lipid toolbox for RNA delivery. Nat. Commun. 12, 7233. doi:10.1038/s41467-021-27493-0
Hatano, J., Okuro, K., and Aida, T. (2016). Photoinduced bioorthogonal 1,3-dipolar poly-cycloaddition promoted by oxyanionic substrates for spatiotemporal operation of molecular glues. Angew. Chem. 128, 201–206. doi:10.1002/ange.201507987
He, X., Long, Q., Zeng, Z., Yang, L., Tang, Y., and Feng, X. (2019). Simple and efficient targeted intracellular protein delivery with self-assembled nanovehicles for effective cancer therapy. Adv. Funct. Mater 29, 1906187. doi:10.1002/adfm.201906187
Horn, J. M., and Obermeyer, A. C. (2021). Genetic and covalent protein modification strategies to facilitate intracellular delivery. Biomacromolecules 22, 4883–4904. doi:10.1021/acs.biomac.1c00745
Hou, Y., Yuan, J., Zhou, Y., Yu, J., and Lu, H. (2016). A concise approach to site-specific topological protein-poly(amino acid) conjugates enabled by in situ-generated functionalities. J. Am. Chem. Soc. 138, 10995–11000. doi:10.1021/jacs.6b05413
Jiang, Y., Yang, W., Zhang, J., Meng, F., and Zhong, Z. (2018). Protein toxin chaperoned by LRP-1-targeted virus-mimicking vesicles induces high-efficiency glioblastoma therapy in vivo. Adv. Mater. 30, 1800316. doi:10.1002/adma.201800316
Kaczmarczyk, S. J., Sitaraman, K., Young, H. A., Hughes, S. H., and Chatterjee, D. K. (2011). Protein delivery using engineered virus-like particles. Proc. Natl. Acad. Sci. U. S. A. 108, 16998–17003. doi:10.1073/pnas.1101874108
Kaksonen, M., Toret, C. P., and Drubin, D. G. (2006). Harnessing actin dynamics for clathrin-mediated endocytosis. Nat. Rev. Mol. Cell. Biol. 7, 404–414. doi:10.1038/nrm1940
Keller, M., Kuhn, K. K., Einsiedel, J., Hübner, H., Biselli, S., Mollereau, C., et al. (2016). Mimicking of arginine by functionalized nω-carbamoylated arginine as a new broadly applicable approach to labeled bioactive peptides: High affinity angiotensin, neuropeptide Y, neuropeptide FF, and neurotensin receptor ligands as examples. J. Med. Chem. 59, 1925–1945. doi:10.1021/acs.jmedchem.5b01495
Keppeke, G. D., Andrade, L. E. C., Grieshaber, S. S., and Chan, E. K. L. (2015). Microinjection of specific anti-IMPDH2 antibodies induces disassembly of cytoplasmic rods/rings that are primarily stationary and stable structures. Cell. Biosci. 5, 1. doi:10.1186/2045-3701-5-1
Kerr, M. C., and Teasdale, R. D. (2009). Defining macropinocytosis. Traffic 10, 364–371. doi:10.1111/j.1600-0854.2009.00878.x
Kim, A., Miura, Y., Ishii, T., Mutaf, O. F., Nishiyama, N., Cabral, H., et al. (2016). Intracellular delivery of charge-converted monoclonal antibodies by combinatorial design of block/Homo polyion complex micelles. Biomacromolecules 17, 446–453. doi:10.1021/acs.biomac.5b01335
Koniev, O., and Wagner, A. (2015). Developments and recent advancements in the field of endogenous amino acid selective bond forming reactions for bioconjugation. Chem. Soc. Rev. 44, 5495–5551. doi:10.1039/c5cs00048c
Kube, S., Hersch, N., Naumovska, E., Gensch, T., Hendriks, J., Franzen, A., et al. (2017). Fusogenic liposomes as nanocarriers for the delivery of intracellular proteins. Langmuir 33, 1051–1059. doi:10.1021/acs.langmuir.6b04304
Leader, B., Baca, J. Q., and Golan, E. D. (2008). Protein therapeutics: A summary and pharmacological classification. Nat. Rev. 7, 21–39. doi:10.1038/nrd2399
Lee, J., Sands, I., Zhang, W., Zhou, L., and Chen, Y. (2021). DNA-inspired nanomaterials for enhanced endosomal escape. Available at: https://www.pnas.org/doi/pdf/10.1073/pnas.2104511118 (Accessed April 14, 2021)
Lee, Y. W., Luther, D. C., Kretzmann, J. A., Burden, A., Jeon, T., Zhai, S., et al. (2019). Protein delivery into the cell cytosol using non-viral nanocarriers. Theranostics 9, 3280–3292. doi:10.7150/thno.34412
Li, M., Schlesiger, S., Knauer, S. K., and Schmuck, C. (2015). A tailor-made specific anion-binding motif in the side chain transforms a tetrapeptide into an efficient vector for gene delivery. Angew. Chem. - Int. Ed. 54, 2941–2944. doi:10.1002/anie.201410429
Liew, S. S., Zhang, C., Zhang, J., Sun, H., Li, L., and Yao, S. Q. (2020). Intracellular delivery of therapeutic proteins through N-Terminal site-specific modification. Chem. Commun. 56, 11473–11476. doi:10.1039/d0cc04728g
Liu, B., Ianosi-Irimie, M., and Thayumanavan, S. (2019). Reversible click chemistry for ultrafast and quantitative formation of protein-polymer nanoassembly and intracellular protein delivery. ACS Nano 13, 9408–9420. doi:10.1021/acsnano.9b04198
Liu, X., Wu, F., Ji, Y., and Yin, L. (2019). Recent advances in anti-cancer protein/peptide delivery. Bioconjug Chem. 30, 305–324. doi:10.1021/acs.bioconjchem.8b00750
Liu, X., Zhao, Z., Wu, F., Chen, Y., and Yin, L. (2022). Tailoring hyperbranched poly(β-amino ester) as a robust and universal platform for cytosolic protein delivery. Adv. Mater. 34, 2108116. doi:10.1002/adma.202108116
Loibl, S., and Gianni, L. (2017). HER2-positive breast cancer. Lancet 389, 2415–2429. doi:10.1016/S0140-6736(16)32417-5
Luther, D. C., Huang, R., Jeon, T., Zhang, X., Lee, Y. W., Nagaraj, H., et al. (2020). Delivery of drugs, proteins, and nucleic acids using inorganic nanoparticles. Adv. Drug Deliv. Rev. 156, 188–213. doi:10.1016/j.addr.2020.06.020
Lutz, J. F., and Börner, H. G. (2008). Modern trends in polymer bioconjugates design. Prog. Polym. Sci. Oxf. 33, 1–39. doi:10.1016/j.progpolymsci.2007.07.005
Lv, J., Fan, Q., Wang, H., and Cheng, Y. (2019). Polymers for cytosolic protein delivery. Biomaterials 218, 119358. doi:10.1016/j.biomaterials.2019.119358
Lv, J., Tan, E., Wang, Y., Fan, Q., Yu, J., and Cheng, Y. (2020). Tailoring guanidyl-rich polymers for efficient cytosolic protein delivery. J. Control. Release 320, 412–420. doi:10.1016/j.jconrel.2020.01.056
Miersch, S., and Sidhu, S. S. (2016). Intracellular targeting with engineered proteins. F1000 Fac. Rev. 10 (5), 1947. doi:10.12688/F1000RESEARCH.8915.1
Maier, K., and Wagner, E. (2012). Acid-labile traceless click linker for protein transduction. J. Am. Chem. Soc. 134, 10169–10173. doi:10.1021/ja302705v
Marschall, A. L. J., Zhang, C., Frenzel, A., Schirrmann, T., Hust, M., Perez, F., et al. (2014). Delivery of antibodies to the cytosol: Debunking the myths. MAbs 6, 943–956. doi:10.4161/mabs.29268
McKinlay, C. J., Waymouth, R. M., and Wender, P. A. (2016). Cell-penetrating, guanidinium-rich oligophosphoesters: Effective and versatile molecular transporters for drug and probe delivery. J. Am. Chem. Soc. 138, 3510–3517. doi:10.1021/jacs.5b13452
Mitragotri, S., Burke, P. A., and Langer, R. (2014). Overcoming the challenges in administering biopharmaceuticals: Formulation and delivery strategies. Nat. Rev. Drug Discov. 13, 655–672. doi:10.1038/nrd4363
Moncalvo, F., Martinez Espinoza, M. I., and Cellesi, F. (2020). Nanosized delivery systems for therapeutic proteins: Clinically validated technologies and advanced development strategies. Front. Bioeng. Biotechnol. 8, 89. doi:10.3389/fbioe.2020.00089
Mukherjee, P., Nathamgari, S. S. P., Kessler, J. A., and Espinosa, H. D. (2018). Combined numerical and experimental investigation of localized electroporation-based cell transfection and sampling. ACS Nano 12, 12118–12128. doi:10.1021/acsnano.8b05473
Mulgrew-Nesbitt, A., Diraviyam, K., Wang, J., Singh, S., Murray, P., Li, Z., et al. (2006). The role of electrostatics in protein-membrane interactions. Biochim. Biophys. Acta Mol. Cell. Biol. Lipids 1761, 812–826. doi:10.1016/j.bbalip.2006.07.002
Nabi, I. R., and Le, P. U. (2003). Caveolae/raft-dependent endocytosis. J. Cell. Biol. 161, 673–677. doi:10.1083/jcb.200302028
Niamsuphap, S., Fercher, C., Kumble, S., Huda, P., Mahler, S. M., and Howard, C. B. (2020). Targeting the undruggable: Emerging technologies in antibody delivery against intracellular targets. Expert Opin. Drug Deliv. 17, 1189–1211. doi:10.1080/17425247.2020.1781088
Nishimura, Y., Takeda, K., Ezawa, R., Ishii, J., Ogino, C., and Kondo, A. (2014). A display of pH-sensitive fusogenic GALA peptide facilitates endosomal escape from a Bio-nanocapsule via an endocytic uptake pathway. J. Nanobiotechnology 12, 11. doi:10.1186/1477-3155-12-11
Obermeyer, A. C., Mills, C. E., Dong, X. H., Flores, R. J., and Olsen, B. D. (2016). Complex coacervation of supercharged proteins with polyelectrolytes. Soft Matter 12, 3570–3581. doi:10.1039/c6sm00002a
Pakulska, M. M., Miersch, S., and Shoichet, M. S. (2016). Designer protein delivery: From natural to engineered affinity-controlled release systems. Science 351 (6279), aac4750. doi:10.1126/science.aac4750
Pandya, A. K., and Patravale, V. B. (2021). Computational avenues in oral protein and peptide therapeutics. Drug Discov. Today 26 (6, 1510–1520. doi:10.1016/j.drudis.2021.03.003
Parodi, A., Molinaro, R., Sushnitha, M., Evangelopoulos, M., Martinez, J. O., Arrighetti, N., et al. (2017). Bio-inspired engineering of cell- and virus-like nanoparticles for drug delivery. Biomaterials 147, 155–168. doi:10.1016/j.biomaterials.2017.09.020
Posey, N. D., and Tew, G. N. (2018). Associative and dissociative processes in non-covalent polymer-mediated intracellular protein delivery. Chem. Asian J. 13, 3351–3365. doi:10.1002/asia.201800849
Postupalenko, V., Desplancq, D., Orlov, I., Arntz, Y., Spehner, D., Mely, Y., et al. (2015). Protein delivery system containing a nickel-immobilized polymer for multimerization of affinity-purified his-tagged proteins enhances cytosolic transfer. Angew. Chem. 127, 10729–10732. doi:10.1002/ange.201505437
Prasetyanto, E. A., Bertucci, A., Septiadi, D., Corradini, R., Castro-Hartmann, P., and De Cola, L. (2016). Breakable hybrid organosilica nanocapsules for protein delivery. Angew. Chem. 128, 3384–3388. doi:10.1002/ange.201508288
Qian, L., Fu, J., Yuan, P., Du, S., Huang, W., Li, L., et al. (2018). Intracellular delivery of native proteins facilitated by cell-penetrating poly(disulfide)s. Angew. Chem. 130, 1548–1552. doi:10.1002/ange.201711651
Qiao, D., Liu, L., Chen, Y., Xue, C., Gao, Q., Mao, H. Q., et al. (2018). Potency of a scalable nanoparticulate subunit vaccine. Nano Lett. 18, 3007–3016. doi:10.1021/acs.nanolett.8b00478
Qin, X., Yu, C., Wei, J., Li, L., Zhang, C., Wu, Q., et al. (2019). Rational design of nanocarriers for intracellular protein delivery. Adv. Mater. 31, 1902791. doi:10.1002/adma.201902791
Raman, V., Van Dessel, N., Hall, C. L., Wetherby, V. E., Whitney, S. A., Kolewe, E. L., et al. (2021). Intracellular delivery of protein drugs with an autonomously lysing bacterial system reduces tumor growth and metastases. Nat. Commun. 12, 6116. doi:10.1038/s41467-021-26367-9
Ray, M., Lee, Y. W., Scaletti, F., Yu, R., and Rotello, V. M. (2017). Intracellular delivery of proteins by nanocarriers. Nanomedicine 12, 941–952. doi:10.2217/nnm-2016-0393
Ren, Q., Chen, Q., Ren, L., Cao, C., Liu, R., and Cheng, Y. (2022). Amphipathic poly-β-peptides for intracellular protein delivery. Chem. Commun. 58, 4320–4323. doi:10.1039/d2cc00453d
Rui, Y., Wilson, D. R., Choi, J., Varanasi, M., Sanders, K., Karlsson, J., et al. (2019). Carboxylated branched poly(β-amino ester) nanoparticles enable robust cytosolic protein delivery and CRISPR-Cas9 gene editing. Sci. Adv. 5, eaay3255. doi:10.1126/sciadv.aay3255
Sá, J. D. O., Trino, L. D., Oliveira, A. K., Lopes, A. F. B., Granato, D. C., Normando, A. G. C., et al. (2021). Proteomic approaches to assist in diagnosis and prognosis of oral cancer. Expert Rev. Proteomics 18, 261–284. doi:10.1080/14789450.2021.1924685
Sangsuwan, R., Tachachartvanich, P., and Francis, M. B. (2019). Cytosolic delivery of proteins using amphiphilic polymers with 2-pyridinecarboxaldehyde groups for site-selective attachment. J. Am. Chem. Soc. 141, 2376–2383. doi:10.1021/jacs.8b10947
Sarker, S. R., Hokama, R., and Takeoka, S. (2014). Intracellular delivery of universal proteins using a lysine headgroup containing cationic liposomes: Deciphering the uptake mechanism. Mol. Pharm. 11, 164–174. doi:10.1021/mp400363z
Scaletti, F., Hardie, J., Lee, Y. W., Luther, D. C., Ray, M., and Rotello, V. M. (2018). Protein delivery into cells using inorganic nanoparticle-protein supramolecular assemblies. Chem. Soc. Rev. 47, 3421–3432. doi:10.1039/c8cs00008e
Shi, Y., Inoue, H., Wu, J. C., and Yamanaka, S. (2017). Induced pluripotent stem cell technology: A decade of progress. Nat. Rev. Drug Discov. 16, 115–130. doi:10.1038/nrd.2016.245
Slastnikova, T. A., Ulasov, A. V., Rosenkranz, A. A., and Sobolev, A. S. (2018). Targeted intracellular delivery of antibodies: The state of the art. Front. Pharmacol. 9, 1208. doi:10.3389/fphar.2018.01208
Stadtmauer, E. A., Fraietta, J. A., Davis, M. M., Cohen, A. D., Weber, K. L., Lancaster, E., et al. (2020). CRISPR-engineered T cells in patients with refractory cancer. Science 367 (6481), eaba7365. doi:10.1126/science.aba7365
Stanzl, E. G., Trantow, B. M., Vargas, J. R., and Wender, P. A. (2013). Fifteen years of cell-penetrating, guanidinium-rich molecular transporters: Basic science, research tools, and clinical applications. Acc. Chem. Res. 46, 2944–2954. doi:10.1021/ar4000554
Stephanopoulos, N., and Francis, M. B. (2011). Choosing an effective protein bioconjugation strategy. Nat. Chem. Biol. 7, 876–884. doi:10.1038/nchembio.720
Stevens, C. A., Kaur, K., and Klok, H. A. (2021). Self-assembly of protein-polymer conjugates for drug delivery. Adv. Drug Deliv. Rev. 174, 447–460. doi:10.1016/j.addr.2021.05.002
Stewart, M. P., Langer, R., and Jensen, K. F. (2018). Intracellular delivery by membrane disruption: Mechanisms, strategies, and concepts. Chem. Rev. 118, 7409–7531. doi:10.1021/acs.chemrev.7b00678
Stewart, M. P., Sharei, A., Ding, X., Sahay, G., Langer, R., and Jensen, K. F. (2016). In vitro and ex vivo strategies for intracellular delivery. Nature 538, 183–192. doi:10.1038/nature19764
Su, S., Wang, Y. Y., Du, F. S., Lu, H., and Li, Z. C. (2018). Dynamic covalent bond-assisted programmed and traceless protein release: High loading nanogel for systemic and cytosolic delivery. Adv. Funct. Mater 28, 1805287. doi:10.1002/adfm.201805287
Sun, W., Ji, W., Hall, J. M., Hu, Q., Wang, C., Beisel, C. L., et al. (2015). Self-assembled DNA nanoclews for the efficient delivery of CRISPR-cas9 for genome editing. Angew. Chem. 127, 12197–12201. doi:10.1002/ange.201506030
Sun, W., Ji, W., Hu, Q., Yu, J., Wang, C., Qian, C., et al. (2016). Transformable DNA nanocarriers for plasma membrane targeted delivery of cytokine. Biomaterials 96, 1–10. doi:10.1016/j.biomaterials.2016.04.011
Sun, Y., Lau, S. Y., Lim, Z. W., Chang, S. C., Ghadessy, F., Partridge, A., et al. (2022). Phase-separating peptides for direct cytosolic delivery and redox-activated release of macromolecular therapeutics. Nat. Chem. 14, 274–283. doi:10.1038/s41557-021-00854-4
Tan, E., Wan, T., Yu, C., Fan, Q., Liu, W., Chang, H., et al. (2022). ROS-responsive polypeptides for intracellular protein delivery and CRISPR/Cas9 gene editing. Nano Today 46, 101617. doi:10.1016/j.nantod.2022.101617
Tan, H. X., Juno, J. A., Lee, W. S., Barber-Axthelm, I., Kelly, H. G., Wragg, K. M., et al. (2021). Immunogenicity of prime-boost protein subunit vaccine strategies against SARS-CoV-2 in mice and macaques. Nat. Commun. 12, 1403. doi:10.1038/s41467-021-21665-8
Tang, R., Kim, C. S., Solfiell, D. J., Rana, S., Mout, R., Velázquez-Delgado, E. M., et al. (2013). Direct delivery of functional proteins and enzymes to the cytosol using nanoparticle-stabilized nanocapsules. ACS Nano 7, 6667–6673. doi:10.1021/nn402753y
Tang, R., Wang, M., Ray, M., Jiang, Y., Jiang, Z., Xu, Q., et al. (2017). Active targeting of the nucleus using nonpeptidic boronate tags. J. Am. Chem. Soc. 139, 8547–8551. doi:10.1021/jacs.7b02801
Tao, A., Huang, G., Igarashi, K., Hong, T., Liao, S., Stellacci, F., et al. (2020). Polymeric micelles loading proteins through concurrent ion complexation and pH-cleavable covalent bonding for in vivo delivery. Macromol. Biosci. 20, 1900161. doi:10.1002/mabi.201900161
Tezgel, A. Ö., Jacobs, P., Backlund, C. M., Telfer, J. C., and Tew, G. N. (2017). Synthetic protein mimics for functional protein delivery. Biomacromolecules 18, 819–825. doi:10.1021/acs.biomac.6b01685
Tian, L., Kang, H. C., and Bae, Y. H. (2013). Endosomolytic reducible polymeric electrolytes for cytosolic protein delivery. Biomacromolecules 14, 2570–2581. doi:10.1021/bm400337f
Tian, Y., Tirrell, M. V., and LaBelle, J. L. (2022). Harnessing the therapeutic potential of biomacromolecules through intracellular delivery of nucleic acids, peptides, and proteins. Adv. Healthc. Mater 11, 2102600. doi:10.1002/adhm.202102600
Togtema, M., Pichardo, S., Jackson, R., Lambert, P. F., Curiel, L., and Zehbe, I. (2012). Sonoporation delivery of monoclonal antibodies against human papillomavirus 16 E6 restores p53 expression in transformed cervical keratinocytes. PLoS One 7, e50730. doi:10.1371/journal.pone.0050730
Tsao, C., Yuan, Z., Zhang, P., Liu, E., McMullen, P., Wu, K., et al. (2020). Enhanced pulmonary systemic delivery of protein drugs via zwitterionic polymer conjugation. J. Control. Release 322, 170–176. doi:10.1016/j.jconrel.2020.03.019
Ulasov, A. V., Rosenkranz, A. A., and Sobolev, A. S. (2018). Transcription factors: Time to deliver. J. Control. Release 269, 24–35. doi:10.1016/j.jconrel.2017.11.004
Ventura, J., Eron, S. J., González-Toro, D. C., Raghupathi, K., Wang, F., Hardy, J. A., et al. (2015). Reactive self-assembly of polymers and proteins to reversibly silence a killer protein. Biomacromolecules 16, 3161–3171. doi:10.1021/acs.biomac.5b00779
Wang, M., Alberti, K., Sun, S., Arellano, C. L., and Xu, Q. (2014). Combinatorially designed lipid-like nanoparticles for intracellular delivery of cytotoxic protein for cancer therapy. Angew. Chem. - Int. Ed. 53, 2893–2898. doi:10.1002/anie.201311245
Wang, M., Zuris, J. A., Meng, F., Rees, H., Sun, S., Deng, P., et al. (2016). Efficient delivery of genome-editing proteins using bioreducible lipid nanoparticles. Proc. Natl. Acad. Sci. U. S. A. 113, 2868–2873. doi:10.1073/pnas.1520244113
Wang, Y., and Yu, C. (2020). Emerging concepts of Nanobiotechnology in mRNA delivery. Angew. Chem. - Int. Ed. 59, 23374–23385. doi:10.1002/anie.202003545
Weiner, G. J. (2015). Building better monoclonal antibody-based therapeutics. Nat. Rev. Cancer 15, 361–370. doi:10.1038/nrc3930
Wu, D., Qin, M., Xu, D., Wang, L., Liu, C., Ren, J., et al. (2019). A bioinspired platform for effective delivery of protein therapeutics to the central nervous system. Adv. Mater. 31, 1807557. doi:10.1002/adma.201807557
Xie, J., Gonzalez-Carter, D., Tockary, T. A., Nakamura, N., Xue, Y., Nakakido, M., et al. (2020). Dual-sensitive nanomicelles enhancing systemic delivery of therapeutically active antibodies specifically into the brain. ACS Nano 14, 6729–6742. doi:10.1021/acsnano.9b09991
Yan, Y., Zhou, L., Sun, Z., Song, D., and Cheng, Y. (2022). Targeted and intracellular delivery of protein therapeutics by a boronated polymer for the treatment of bone tumors. Bioact. Mater 7, 333–340. doi:10.1016/j.bioactmat.2021.05.041
Yu, C., Tan, E., Xu, Y., Lv, J., and Cheng, Y. (2018). A guanidinium-rich polymer for efficient cytosolic delivery of native proteins. Bioconjug Chem. 30, 413–417. doi:10.1021/acs.bioconjchem.8b00753
Yu, S., Yang, H., Li, T., Pan, H., Ren, S., Luo, G., et al. (2021). Efficient intracellular delivery of proteins by a multifunctional chimaeric peptide in vitro and in vivo. Nat. Commun. 12, 5131. doi:10.1038/s41467-021-25448-z
Zelikin, A. N., Ehrhardt, C., and Healy, A. M. (2016). Materials and methods for delivery of biological drugs. Nat. Chem. 8, 997–1007. doi:10.1038/nchem.2629
Zhang, M., Chen, X., Li, C., and Shen, X. (2020). Charge-reversal nanocarriers: An emerging paradigm for smart cancer nanomedicine. J. Control. Release 319, 46–62. doi:10.1016/j.jconrel.2019.12.024
Zhang, S., Shen, J., Li, D., and Cheng, Y. (2020). Strategies in the delivery of Cas9 ribonucleoprotein for CRISPR/Cas9 genome editing. Theranostics 11, 614–648. doi:10.7150/thno.47007
Zhang, Z., Shen, W., Ling, J., Yan, Y., Hu, J., and Cheng, Y. (2018). The fluorination effect of fluoroamphiphiles in cytosolic protein delivery. Nat. Commun. 9, 1377. doi:10.1038/s41467-018-03779-8
Zhou, Z., Yan, Y., Wang, L., Zhang, Q., and Cheng, Y. (2019). Melanin-like nanoparticles decorated with an autophagy-inducing peptide for efficient targeted photothermal therapy. Biomaterials 203, 63–72. doi:10.1016/j.biomaterials.2019.02.023
Keywords: intracellular delivery, therapeutic proteins, protein delivery, polymeric nanocarriers, cellpenetrating peptides, protein resurfacing
Citation: Porello I and Cellesi F (2023) Intracellular delivery of therapeutic proteins. New advancements and future directions. Front. Bioeng. Biotechnol. 11:1211798. doi: 10.3389/fbioe.2023.1211798
Received: 25 April 2023; Accepted: 16 May 2023;
Published: 25 May 2023.
Edited by:
Marco P. Monopoli, Royal College of Surgeons in Ireland, IrelandReviewed by:
Anjaneyulu Dirisala, Innovation Centre of NanoMedicine (iCONM), JapanNazende Günday-Türeli, MyBiotech GmbH, Germany
Copyright © 2023 Porello and Cellesi. This is an open-access article distributed under the terms of the Creative Commons Attribution License (CC BY). The use, distribution or reproduction in other forums is permitted, provided the original author(s) and the copyright owner(s) are credited and that the original publication in this journal is cited, in accordance with accepted academic practice. No use, distribution or reproduction is permitted which does not comply with these terms.
*Correspondence: Francesco Cellesi, francesco.cellesi@polimi.it