Altered blood gas tensions of oxygen and carbon dioxide confound coronary reactivity to apnea
- 1Philippa & Marvin Carsley CMR Centre at the Montreal Heart Institute, Université de Montréal, Montreal, QC, Canada
- 2Department of Anaesthesiology and Pain Medicine, Inselspital, Bern University Hospital, University of Bern, Bern, Switzerland
- 3Department of Cardiac Sciences and Radiology, University of Calgary, Calgary, AB, Canada
- 4Department of Medicine and Diagnostic Radiology, McGill University Health Centre, Montreal, QC, Canada
- 5Department of Radiology, Université de Montréal, Montreal, QC, Canada
- 6Department of Cardiology, Heidelberg University Hospital, Heidelberg, Germany
Purpose: Arterial blood gases change frequently during anesthesia and intensive care. Apnea can occur during diagnostic exams and airway and surgical interventions. While the impact of blood gas levels on coronary blood flow is established, their confounding effect on coronary vasoreactivity in response to an apneic stimulus, especially in coronary artery disease, is not known.
Methods: Six anesthetized control swine and eleven swine with coronary artery stenosis were examined. Nine different blood gas levels from a combination of arterial partial pressure of oxygen (70, 100, and 300 mmHg) and carbon dioxide (30, 40, and 50 mmHg) were targeted. Apnea was induced by halting controlled positive pressure ventilation for 3–30s, while the left descending coronary artery flow was measured and reported relative to apnea duration, and at the adjusted mean (12s).
Results: At normoxemic-normocapnic blood gas levels, apnea increased coronary blood flow in proportion to the duration of apnea in the control (r = 0.533, p < 0.001) and stenosed groups (r = 0.566, p < 0.001). This culminated in a 42% (95% CI: 27–58) increase in controls (p < 0.001) and, to a lesser extent, 27% (15–40) in the presence of coronary artery stenosis (p < 0.001). Vasoreactivity was augmented by mild-hypoxemic levels [81% (65–97), and 66% (53–79) increase in flow respectively, p < 0.001 vs. normoxemia], but markedly reduced during hyperoxia (7.5% (−8.2–23) and 0.3% (−12–13), respectively, p < 0.001 vs. normoxemia).
Conclusion: Alterations of blood oxygen and carbon dioxide affect coronary vascular reactivity induced by apnea in swine, which was attenuated further in the presence of coronary stenosis. Especially hyperoxia significantly reduces coronary blood flow and blunts coronary vascular reactivity.
Background
Adequate coronary blood supply is the key component of oxygen homeostasis and failure to adequately perfuse the myocardium can lead to myocardial ischemia. The heart has a very high oxygen (O2) consumption. Coronary blood flow is carefully controlled in response to factors such as oxygen demand and perfusion pressures [1, 2]. Several metabolic substances act as physiologic vasodilators. Increased adenosine production, high partial pressures of carbon dioxide (PaCO2), and low partial pressure of oxygen (PaO2) are all positively correlated with coronary blood flow, with a particularly strong effect on microvessels [1, 3–5]. Hypocapnia and hyperoxia on the other hand trigger vasoconstriction with a subsequent reduction in blood flow [6, 7]. Persistent changes in coronary blood flow, as well as temporary adaptations, are subject to local metabolic blood flow regulation.
Therapeutic strategies in fields such as anesthesia and intensive care focus on maintaining adequate oxygenation and perfusion pressure to secure the oxygenation of vital organs. Physiological responses in the patient along with external influences such as medical interventions can modulate PaO2 and PaCO2 in the blood, resulting in a combination of hypoxemic to hyperoxic and hypocapnic to hypercapnic environments [8–10]. Due to the aforementioned impact of metabolites on coronary vasculature, prolonged exposure to these blood gas states has the potential to shift coronary blood flow to a new equilibrium or steady state [5, 6, 11]. Published evidence for the vasoconstricting effects of hyperoxia has been under-appreciated, and only during recent years, a stronger awareness of the potentially detrimental effects of hyperoxia has emerged, especially for emergency medicine, intensive, and perioperative care. These negative effects include the development of cardiovascular dysfunction, myocardial injury, higher rates of cardiovascular adverse events, and mortality [12–17]. In addition to sustained changes in coronary blood flow, transient and fast-acting vasodilatory stimuli are vital feedback responses that increase blood flow and oxygen supply to the tissues in a short timeframe to avoid ischemia. Apnea is an example of a short-acting stimulus, it occurs frequently during anesthesia and induces vasodilation as a feedback mechanism in the coronary and cerebrovascular systems [18–20]. Apnea as short as even 10s is known to lead to a detectable hyperemic response in cerebral and myocardial tissue [21–24]. While the gradual and transient responses to vasoactive stimuli present in anesthesia and intensive care environments have been individually investigated, data are scarce on potential interactions from a combination of these mechanisms. This can be of concern if certain combinations attenuate or even block hyperemic responses, thereby predisposing myocardial tissue to inducible ischemia. In particular, data are not yet available on how clinically relevant deflections in PaO2 and PaCO2 may alter the coronary vascular response to apneic stimuli.
In an experimental model, we assessed the impact of baseline blood gas levels on the coronary reactivity to a vasodilatory stimulus induced by a short apnea in swine with and without significant coronary stenosis.
Materials and methods
This study was conducted in accordance with the Guide to the Care and Use of Experimental Animals by the Canadian Council on Animal Care and approved by the local Animal Care and Use Board.
Surgical preparation
Seventeen, juvenile, healthy swine (32 ± 2 kg, 4–6 months, Yorkshire-Landrace) were included in the study [11, 19]. Swine were premedicated with 4 ml Telazol IM (200 mg tiletamine, 200 mg zolazepam), and intubated after 2–4 mg/kg propofol IV. Anesthesia was maintained with propofol (4–36 mg/kg/h IV) and remifentanil (0–3.5 μg/kg/min IV) as required. Percutaneous cannulation of the femoral artery and vein was performed for drug and fluid administration and the collection of blood gas and invasive blood pressure measurements. For analysis of coronary blood flow, an ultrasound flow probe was surgically implanted on the proximal left anterior descending (LAD) coronary artery of all swine (Transonic Systems, Ithaca, NY, USA), requiring a left-sided thoracotomy (Figure 1) [25]. Six swine served as controls, while in eleven swine, a hemodynamically relevant coronary artery stenosis was induced using a perivascular hydraulic occluder (In Vivo Metric, CA, USA). The severity of the stenosis was guided by fractional flow reserve (FFR, St. Jude Medical, MN, USA) to target a trans-stenotic FFR of < 0.75 as described before [19].
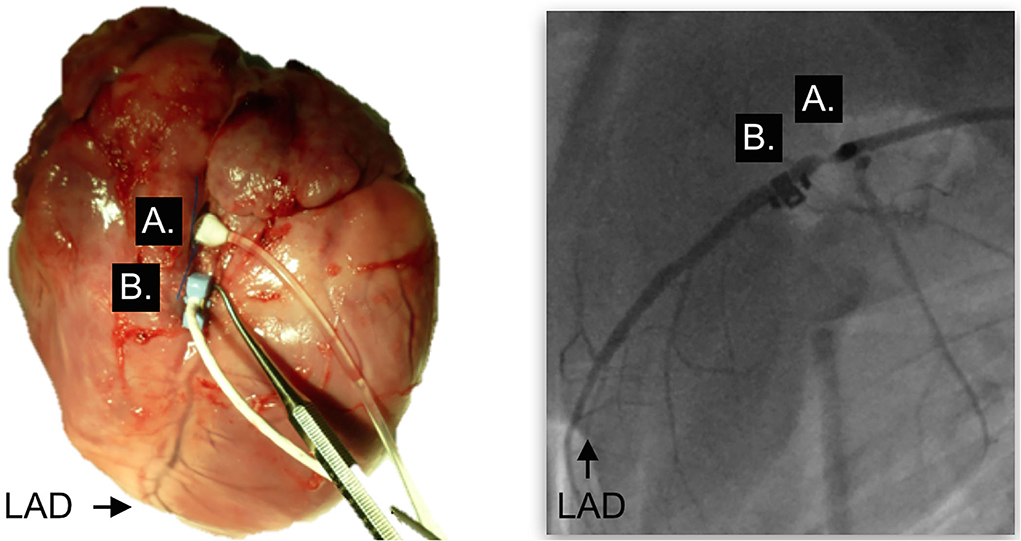
Figure 1. Surgical placement of the occluder and flow probe. Situs after surgical placement of the perivascular occluder (A) and the blood flow probe (B) on the left anterior descending coronary artery (LAD). Left panel, Explanted heart; right panel, fluoroscopy in the same swine in-vivo; LAD, left anterior descending.
Experimental protocol
Approximately 60 min after completion of the surgical procedure, arterial blood gas levels were targeted to nine levels of different combinations in arterial PaO2 and PaCO2 that can realistically be encountered in clinical scenarios: PaO2 of 70, 100, or >300 mmHg (mild hypoxemia, normoxemia, and hyperoxia) and a PaCO2 of 30, 40, or 50 mmHg (hypocapnia, normocapnia, and hypercapnia). The normal gas level of normoxemic normocapnia (PaO2 = 100, PaCO2 = 40 mmHg) was applied twice, first at the beginning and then in a random order together with the other levels to assess if baseline vascular tone changed during the protocol and as a quality control to exclude fluctuations or drift of blood flow as a function of time or other unknown confounders. These nine levels were classified as steady-state levels, indicating hemodynamics and blood gases remained stable and were not fluctuating at the time of data acquisition. After arterial blood gas levels were stabilized for at least 5 min, up to six episodes of apnea were induced after a steady state of each blood gas level was reached using an end-expiratory pause in ventilation. Apnea duration ranged from 3 to 30s. Flow results were expressed as absolute blood flow values (ml/min) and as the percent apnea-induced change (%) from the steady-state conditions to measure coronary vasoreactivity. The blood flow at each steady state was compared between the gas levels and the normoxemic-normocapnic 100/40 mmHg (PaO2/PaCO2) level within each group. The temporary blood flow response was assessed for its correlation with the duration of apnea, for differences between gas levels, and the impact of coronary artery stenosis. Finally, 200 mg propofol and 40 mmol KCl (i.v.) were administered for euthanasia.
Statistical analysis
A univariate analysis was performed to investigate the change in coronary flow, in response to the duration of apnea. A within-subject correlation analysis was performed to compare the absolute and percent-change response (%) of the blood flow to apnea, accounting for both repeated measurements within each individual, and different individual intercepts. To examine the differences between groups and blood gas levels, a two-way mixed effect model was performed with an unstructured covariance and restricted maximum likelihood estimation. The model used the duration of apnea as the covariate and allowed for random intercepts of each individual. The absolute blood flow and magnitude of the flow responses were assessed at each blood gas level between the control and stenosed groups. Within each group, this flow response at each blood gas level was compared to baseline (100/40 mmHg), using the Bonferroni method accounting for multiple comparisons. The response in coronary blood flow was assessed for both the flow response in relation to the duration of apnea and calculated for an adjusted mean apnea time. Angiography and hemodynamic measures are presented as mean ± SD, while the remainder of data produced from mixed effect models are reported as estimated marginal means (upper and lower bounds of 95% confidence intervals). The tests were performed with GraphPad Prism version 9.0 for Mac OS (GraphPad Software, California USA), and R Software (version 3.4.4, “rmcorr” package) [26]. The results were considered statistically significant with a two-tailed P-value of < 0.05.
Results
In the six control swine, 279 breath-holds were recorded. In one swine with coronary artery stenosis, fatal ventricular fibrillation occurred during the coronary intervention, resulting in ten swine with coronary artery stenosis and 432 breath-holds. The mean FFR was 0.63 ± 0.05, (range 0.54–0.74), with a mean diameter stenosis of 63 ± 11%, (range:52–86%). Blood pressure and arterial oxygen saturation (SaO2) for each level are provided in Supplementary Table 1.
Steady-state coronary blood flow
There was no difference in the coronary flow recordings between the two sets of measurements obtained at different times during the procedure at the normal gas level (p = 0.614). Targeting the different blood gas level combinations impacted the coronary blood flow creating a new steady state different from the 100/40 mmHg baseline (Supplementary Table 2). In both the control and stenosis groups, the combination of mild hypoxemia and hypercapnia significantly increased flow from the baseline of 100/40 mmHg, whereas some hyperoxic levels decreased flow.
Mean coronary vascular response to an apneic stimulus
As seen in Figure 2, at the normoxemic-normocapnic baseline (100/40 mmHg), short breath-holds were able to significantly and transiently recruit coronary blood flow. In controls, a 42% increase (p < 0.001) was observed by 12s of apnea reaching an adjusted mean blood flow of 36 (25–50) ml/min from a steady-state blood flow of 26 (19–34) ml/min (Table 1, Supplementary Table 2). A significant 27% (p < 0.001) increase was also observed in the swine with stenosis from a baseline flow of 21 (17–26) ml/min, yet the flow response was significantly lower in the stenosis group (p = 0.01, Figure 3).
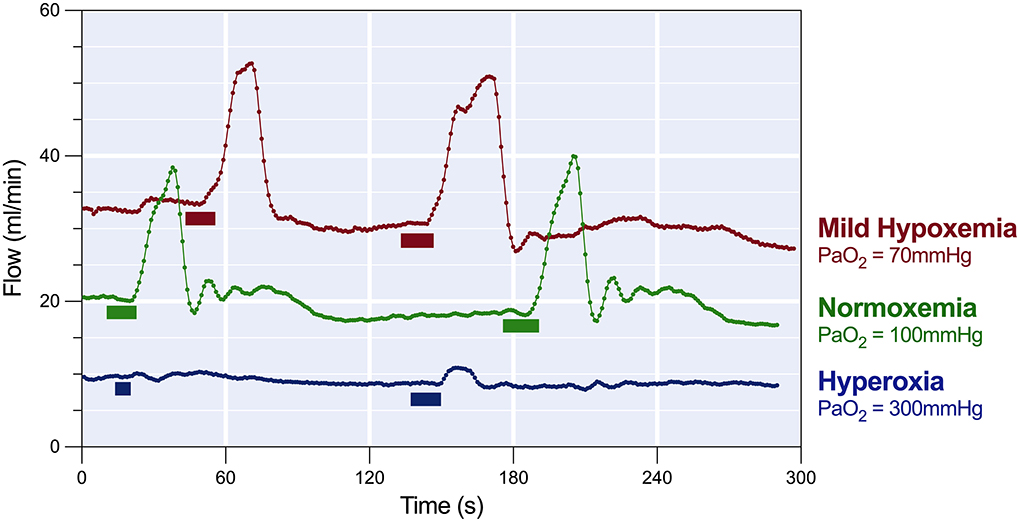
Figure 2. Response of coronary blood flow to breath-holds. The coronary flow read-out of the LAD shows that in a healthy test swine at normocapnic normoxemia (green, PaO2 = 100 mmHg), end-expiratory breath-holds of 8 to 12s (block markers) nearly doubled the coronary blood flow before returning to baseline. In the same subject, during mild normocapnic hypoxemia (red, PaO2 = 70 mmHg) resting blood flow was higher, and apnea of the same duration induced a greater temporary blood flow, while normocapnic hyperoxia (blue, PaO2 > 300 mmHg) reduced steady state blood flow and the response to apnea was minimal.
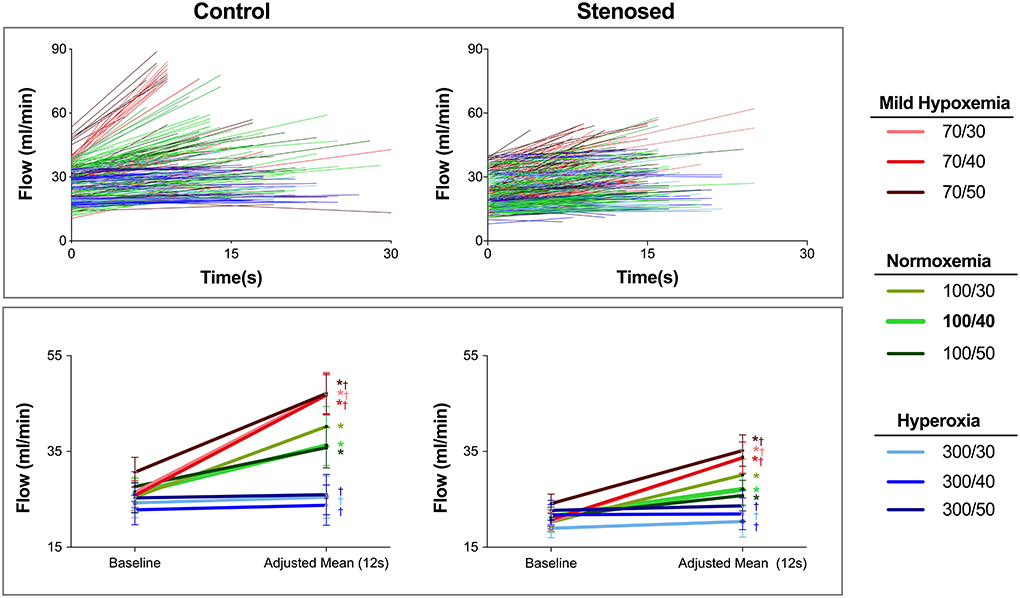
Figure 3. Absolute blood flow during steady state and apnea. Top: The absolute blood flow for each individual apneic stimulus is shown for control (left) and stenosed swine (right) with lines connecting the start of the measurements (t = 0s), and the blood flow at the end of each apnea based on the individual duration of each apnea. In control swine, hypoxemic levels start with a higher baseline blood flow and result in the highest blood flow at the end of apnea. On the other hand, with the hyperoxic levels in the control subjects, the lines are horizontal indicating blood flow did not show a relevant change. This finding is similar to the majority of responses at normoxemia and hyperoxia in the stenosed group where many data points have a lower slope. Bottom: The estimated mean and 95% confidence intervals for absolute blood flow at steady state and at the statistically defined adjusted mean (12s) are shown. *: p < 0.05 for a significant apnea-induced flow recruitment (baseline vs. the adjusted mean). †: p < 0.05, indicating that the magnitude of vasoreactivity was different than observed at 100/40 for each group.
Mean coronary vascular response to apnea with deviated steady-state blood gases
Mild-hypoxemia (PaO2 = 70 mmHg) and hyperoxic (PaO2 = 300 mmHg) steady states significantly impacted the blood flow response to apnea in comparison to the response observed at physiological blood gases (100/40 mmHg, Supplementary Figure 1). At a PaO2 = 70 mmHg, the blood flow response was significantly augmented for all PaCO2 levels (p < 0.001). An increase of at least 50% (34–66) was induced by apnea in controls and by 45% (33–58) in stenosed subjects (Table 1). However, in all hyperoxic levels for both groups, there were no significant flow changes from baseline values (Figure 3, Supplementary Table 2). Once breathing restarted, blood flow returned to the steady state of each arterial blood gas level's baseline.
Coronary vascular response in relation to apnea duration
Analysis was then performed to assess if there was a linear relationship between the flow response and the duration of apnea (Figure 4) and if this differed between groups. In both the control (r = 0.533, p = 0.01) and stenotic groups (r=0.566, p < 0.001), the flow response was positively correlated to a longer apnea duration. Similar to the normal blood gas level, all other normoxemic levels with different PaCO2 responded linearly to the stimuli as well (Figure 4). Apart from hyperoxic-hypocapnia (300/30 mmHg) in controls, no linear relationship was observed for any other hyperoxic level in any group with the duration of apnea, as flow did not increase despite longer apneic stimuli. Moreover, only at normoxemic normocapnia, was this apnea duration-dependent coronary flow response significantly lower in the stenotic group in comparison to the control group. At all other arterial blood gas levels, the presence of coronary artery stenosis could not be distinguished based on the coronary blood flow response.
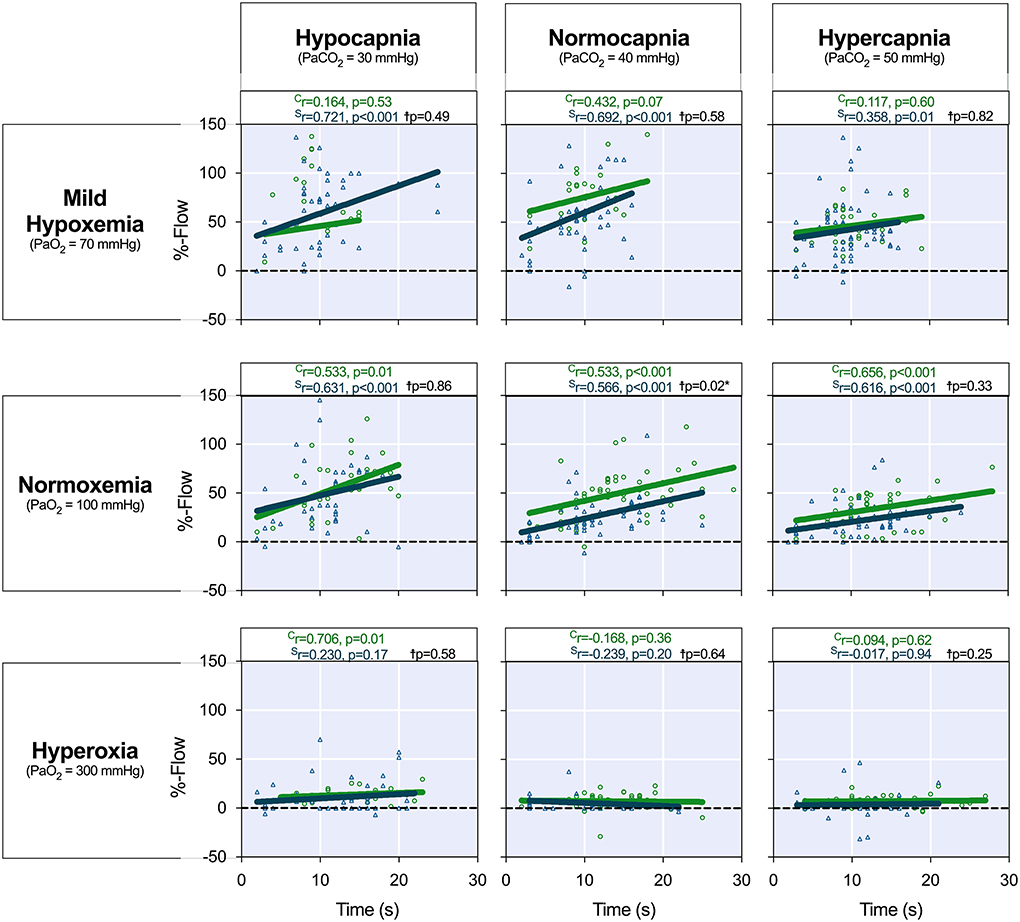
Figure 4. Coronary blood flow response to apnea duration. The coronary artery blood flow response (%-Flow) was calculated as a percent change in blood flow between the start and end of apnea. The %-flow (y-axis) is shown in relation to each individual apnea duration (x-axis) at each arterial blood gas level. The correlation coefficient investigating the flow response (%) in relation to the apnea duration for each level is provided for both the control (green, Cr) and stenosed (blue, Sr) swine. The results reported on the graph (p-value, black) from a mixed model showed that only at PaO2 = 100 mmHg/PaCO2 = 40 mmHg was a significant difference in the flow response (p = 0.020) observed between the groups when accounting for the duration. Hyperoxia resulted in a blunted or absent vascular response to apnea independent of the PaCO2.†Indicates the comparison of the flow response between the health and stenosed groups.
Discussion
Our experimental results demonstrate that in an anesthetized model, shifts in arterial blood gases impact the steady-state coronary blood flow, while short apnea stimuli under 30s result in a significant transient increase in coronary blood flow proportionate to the duration of apnea. This regulatory response to apnea however is confounded by varying PaO2. Mild hypoxemia augmented both steady-state coronary flow and the flow response to apnea, even in the presence of significant coronary stenosis, while arterial hyperoxia resulted in a reduction of steady-state coronary flow and blunts the coronary vascular response to apnea, independent of the presence or absence of severe coronary artery stenosis.
Clinical situations of altered arterial blood gases
The blood gas levels targeted in this study are considered mildly abnormal but are frequently encountered in clinical scenarios, including in spontaneously breathing patients, during anesthesia procedures, and during mechanical ventilation. For example, while PaCO2 values of 38 to 40 mmHg would be considered normal in awake humans [27], a recent study found that 34% of patients with acute heart failure were hypercapnic (PaCO2 > 45 mmHg) at the time of admission, while 33% (PaCO2 < 35 mmHg) were hypocapnic [9]. Additionally, a temporary change in breathing patterns alters PaCO2 and thus coronary vascular reactivity as well, e.g., the change caused by hyperventilation in patients with anxiety. This also applies to hypoventilation in patients with sleep apnea. It has been reported that sleep apnea results in a higher cerebrovascular reactivity, counter-intuitive to the assumption that an adaptation to repetitive hypercapnic and hypoxemic events during the night attenuates this response [28–30]. In general, anesthesia fluctuations of PaCO2 occur frequently. Certain surgical procedures such as capnoperitoneum during laparoscopic surgery shift steady-state blood flow, while other procedures may require a forced apnea such as imaging exams, or require low tidal volumes or even jet-ventilation that makes the elimination of end-tidal CO2 more difficult. Newer practices even advocate for extended apnea periods for some interventions using high-flow nasal oxygen therapy to secure oxygenation with and without apnea [31, 32]. Profound increases in PaCO2 up to 89 mmHg triggered by apnea have been reported in these cases and the effects on coronary function have yet to be assessed [33]. The administration of opioids reduces respiratory drive and may potentially lead to an increase in PaCO2. Whereas, preoxygenation can lead to a reduction in PaCO2 when the patient is asked to take deep breaths. This can be aggravated by excessive manual bag ventilation. During endotracheal intubation or insertion of a supraglottic airway device when ventilation is arrested, PaCO2 will likely rise again, which can be more pronounced when a rapid sequence induction is performed. In this study, the normo- or hypercapnic and hyperoxic baseline followed by 30 s of apnea likely best reflect this clinical situation.
The fraction of inspired oxygen (FiO2) during the maintenance of anesthesia varies between 30 and 80% in literature as there is no universal consensus about target PaO2 levels in adults [34–36]. In our model, we targeted normoxemic (PaO2 of 100 mmHg) and hyperoxic (PaO2 of 300 mmH) states, which are frequently encountered during general anesthesia. Hyperoxia can be achieved quite easily and just 7 min of breathing oxygen through a facemask with a reservoir bag could increase PaO2 > 400 mmHg in healthy participants and patients with coronary artery disease (CAD) [10]. This can be further aggravated with positive pressure ventilation and/or the addition of positive end-expiratory pressure in spontaneously breathing patients. Moreover, oxygen is often the first intervention in emergency medicine. Especially in out-of-hospital settings, including ambulance and other first responder services, there may be a desire to err on the side of caution and avoid hypoxemia by over-oxygenating. A retrospective analysis demonstrated that in trauma patients receiving pre-hospital emergency anesthesia, hyperoxic blood gases levels were common; 61% were severely hyperoxic (PaO2>26.6kPa, 200 mmHg) upon arrival at the hospital, and only < 1% were hypoxemic (PaO2 < 8kPa, 60 mmHg) [8]. Consequently, many recommendations now suggest guiding oxygen supply based on arterial or peripheral oxygen saturation levels (SaO2/SpO2) [37, 38]. Arterial blood gas analysis may not always be available, and saturation-based measures are limited in that they are unable to quantify the degree of hyperoxia. New non-invasive devices such as the oxygen reserve index can indicate PaO2 measurements in the hyperoxic range and could potentially improve FiO2 titration as well [39].
We also targeted mild-hypoxemic levels of 70 mmHg. Under physiologic conditions in elderly patients, similar PaO2 levels can be found due to a reduced alveolar-arteriolar diffusion coefficient [40]. A PaO2 of 70 mmHg may not even be considered hypoxemic by some as this oxygen tension often results in an arterial saturation > 90%. In fact, for relatively healthy persons over the age of 70 without any pulmonary or respiratory diseases, the mean PaO2 for men was reported to be 77 mmHg and 73 mmHg for women, and PaO2 of 60 mmHg could be considered normal [27]. For younger healthy individuals, the PaO2 is expected to be > 80 mmHg. Thus, we labeled this level mildly hypoxemic.
Impact of blood gas changes on steady-state blood flow
Across the nine levels targeted in this study, blood flow was augmented when targeting the level 70/50, composed of two vasodilating stimuli, mild hypoxemia (PaO2 = 70 mmHg) and mild hypercapnia (PaCO2 = 50 mmHg) for both controls and stenosed groups. Each on its own is known to augment blood flow, and their combination has synergistic effects [1]. Additionally, an animal study showed that in swine who were mildly hypoxemic at rest, myocardial oxygenation was still maintained during a 60-s period of apnea, despite a significantly greater blood desaturation than observed during apnea conducted at normoxemia [41]. Other studies have looked at the protective effect of hypercapnia or mild hypoxemia on the skeletal microvascular function [42] and the brain. For example, in a rat model assessing cerebral ischemia, hypercapnia was found to be therapeutic under mild-to-moderate hypoxemia, although detrimental when combined with severe hypoxemia (PaO2 < 50 mmHg) [43]. Consequently, under certain circumstances, vasodilating blood gas combinations may be cardioprotective in a balanced perfusion system that globally distributes blood flow. However, this may not be observed in compromised coronary systems, as vessels downstream to a fixed significant coronary stenosis, or hearts with significant microvascular disease may already be in compensatory dilation and unable to dilate further in response to increased oxygen demand [44]. Furthermore, coronary arteries with obstructive stenosis or a downstream microvascular disease can also be prone to the coronary steal effect, where induced vasodilation directs blood flow away from post-stenotic territories [45, 46].
The vasoconstriction associated with hyperoxia is especially concerning. Oxygen is one of the most used substances in clinical care, especially perioperatively. Our results showed that in both groups, some hyperoxic levels led to a significant decrease in steady-state coronary blood flow. This persistent reduction in blood supply could pose a problem to patients already at risk of ischemia. More recent publications hint at adverse cardiocirculatory effects. A recent publication has shown an association of hyperoxia during general anesthesia with myocardial injury after non-cardiac surgery in patients with known CAD or suspicion thereof [47]. Oxygen administration extends beyond anesthesia and is still a standard in emergency care despite its known vasoconstrictive properties. While there are mixed reports about clinical outcomes as a result of hyperoxia, overall there is a shift toward recognition of the potential detrimental properties in both cardiac and non-cardiac emergencies [17, 48]. Although the long-term impact of hyperoxia remains controversial, our data show there is an immediate consequence of supplemental oxygen on the vasculature. Interestingly, when normoxemic blood gas levels were reinstated, coronary steady-state blood flow and reactivity both returned, suggesting these detrimental effects can be reversed upon correction of arterial blood gases. We have also demonstrated that in swine and patients with chronic coronary syndromes, hyperoxia led to an immediate myocardial oxygenation deficit in the presence of a stenosis, with a matching functional deficit [11, 49]. However, the effect of hyperoxia on oxygen delivery can also differ between patient groups, with heart failure patients more likely to be susceptible to reduced cardiac output and systemic vascular resistance than other patient groups such as septic patients [50], and hyperoxia in a clinical setting remains an interesting and open topic for investigation.
Impact of apnea on the coronary vasomotor response
During anesthesia and other medical procedures, the heart is exposed to many vasoactive stimuli, and the apnea-induced transient augmentation of coronary blood flow is a compensatory mechanism to improve coronary perfusion. In the presence of coronary artery stenosis, the recruitment of blood flow was not as effective as in healthy vessels. This is likely due to the compensatory dilation of the microvessels distal to severe stenosis, with subsequent attenuation of the coronary reserve in these territories. We previously demonstrated that apnea following hyperventilation leads to a transient excess of myocardial oxygenation in healthy humans, but to an oxygenation deficit in patients with stable CAD [45]. By applying apnea of different durations in this study, we were able to identify that the magnitude of the vascular response is also dependent on its duration. Therefore, during extended apnea, e.g., difficult intubation or intentional procedure-related apnea, the added hypoxemia may improve myocardial blood flow to compensate for the decreasing hemoglobin oxygenation but have detrimental effects on tissue with associated coronary artery stenosis. The vasodilatory effects of apnea are not fully understood but are believed to be mediated by multiple mechanisms, which focus on a combined function of the respiratory, cardiac parasympathetic and vasomotor centers [51–53]. While longer breath-holds have the potential to alter the PaO2 and PaCO2 in the blood, the shorter apneas applied in our study are unlikely to significantly alter blood gas composition [54]. Accordingly, we still observed that apnea even shorter than 10s can increase coronary blood flow by more than 30%. More research is needed to elicit the underlying physiologic mechanisms of the observed rapid effects.
We observed that hyperoxia inhibits the vascular response to apnea. Additionally, because hyperoxia itself already reduces coronary blood flow [7, 11, 55], blocking the vasodilatory ability can further compromise tissue oxygenation in the presence of higher oxygen demand. Importantly, if hyperoxia attenuates the protective effect of compensatory recruitment of blood flow by apnea, this may leave the heart susceptible to perioperative ischemia.
Implications for diagnostic settings
On the diagnostic side, cardiovascular imaging and function exams exploit the coronary flow reserve for diagnostic purposes for testing the maximal functional capacity of the coronary vessels rather than measuring absolute blood flow. However, many of these tests assume that their stimuli produce a maximal hyperemic response, with no attenuating factors other than vascular dysfunction. As seen in Figure 3, all mildly hypoxemic and hyperoxic levels caused a different blood flow response than those seen at normal blood gases in our experimental model, and only at normoxemia could a difference between healthy and stenosed vessels be observed. The attenuation of the vascular response to stimuli during hyperoxia could also have implications for coronary stress testing if supplemental oxygen is administered. Diagnostic tests typically use pharmacological vasodilators such as adenosine as a hyperemic stimulus and this may affect the coronary flow response differently than the apnea stimulus used in this and other studies, but similar findings have been reported with other stimuli. A Doppler assessment of coronary flow in five patients with CAD showed that hyperoxia (PaO2 > 250 mmHg) did not affect the coronary response to adenosine, yet the dilator response of acetylcholine was blunted [7]. Opposite to the effects of hyperoxia, we observed that a strong response to coronary stimuli occurs when arterial blood gases are at a mild stage of hypoxemia (PaO2 = 70 mmHg). In a high-altitude-simulation nuclear imaging study, hypoxemia increased the myocardial blood response to exercise-induced stimulation by 38%. In patients with CAD, however, hypoxemia increased baseline coronary flow yet was still associated with a reduced blood supply during exercise, whereas no impact was seen with adenosine [56]. Others have reported that hypoxemia augmented the adenosine response when compared to normoxemia [57]. Under these conditions, a stenotic vessel could give a flow response that would be quantitatively considered healthy under normal situations, possibly masking coronary vascular dysfunction. It should also be noted that the current study focused on mild arterial hypoxemia (SaO2 > 93%), and not severe hypoxemia or overt tissue hypoxia, which may have a different impact on the coronary flow response. It would appear advisable to conduct coronary function testing at normal PaO2 for the individual, represented by a physiological SaO2.
Limitations and future directions
This is a study with a limited sample size and thus sensitive to outliers or any other non-systematic source of bias. Our experimental model is limited in that we assess an essentially healthy coronary artery with a single, acute stenosis, not accounting for the many pathophysiologic and compensatory mechanisms occurring with the development of CAD, including complex multi-vessel or microvascular disease and dysfunctions of the endothelial and smooth muscle cells. Additionally, the blood flow measurement is only obtained from the LAD, and thus our assessment cannot account for the complexity of coronary steal or different baseline flow rates of different vascular territories. The probe only reported mean blood flow, and the impact of the stimuli on pulsatile features could not be assessed. As we only assessed stimuli induced by apnea, results may differ from other stimuli. This could provide a future study goal. In addition, our model does not account for the complicating effects of microvascular dysfunction in CAD.
Conclusion
Using an experimental swine model with invasive coronary blood flow measurements, we provide one of the first reports investigating the confounding effects of mild deflections in PaO2 and PaCO2 on apnea-induced hyperemia. Even though deflections in blood gases are common in perioperative and medical-care environments, the interactions of these deflections on fast-acting vasodilating stimuli are not well understood. Short periods of apnea at physiologic blood gas conditions induced an observable coronary flow recruitment during normocapnic normoxemia, which was attenuated in the presence of a coronary artery stenosis. However, this response was confounded by mild deflections of blood gas levels. While baseline mild hypoxemia augmented the apnea-induced increase in blood flow, hyperoxia almost completely blunted this response. Thus, these findings could have important implications on myocardial oxygenation in situations where blood gas fluctuations are prevalent and patients are at higher risk for myocardial ischemia.
Data availability statement
The raw data supporting the conclusions of this article will be made available by the authors, without undue reservation.
Ethics statement
The animal study was reviewed and approved by Animal Care and Use Board of the Montreal Heart Institute, Montreal, Canada.
Author contributions
Study conception and design contributed by KF, DG, and MF. Material preparation, data collection, and analysis were performed by KF, DG, NS, GN, and JL. The first draft of the manuscript was written by KF, DG, and MF and all authors revised the manuscript. All authors read and approved the final manuscript.
Funding
Funding was provided by the Montreal Heart Institute Foundation, the Canadian Foundation for Innovation, and the Fonds de Recherche du Québec. Open Access funding provided by University of Bern.
Acknowledgments
We are greatly appreciative to Janelle Yu, Stefan Huettenmoser, Camilo Molina, and the members of the Philippa and Marvin Carsley CMR Research Center, and the laboratory of Jean-Francois Tanguay for assisting with the experiments.
Conflict of interest
Author MF declares shareholder interest in Area19 Inc., and Circle Cardiovascular Imaging Inc.
The remaining authors declare that the research was conducted in the absence of any commercial or financial relationships that could be construed as a potential conflict of interest.
Publisher's note
All claims expressed in this article are solely those of the authors and do not necessarily represent those of their affiliated organizations, or those of the publisher, the editors and the reviewers. Any product that may be evaluated in this article, or claim that may be made by its manufacturer, is not guaranteed or endorsed by the publisher.
Supplementary material
The Supplementary Material for this article can be found online at: https://www.frontiersin.org/articles/10.3389/fanes.2022.997836/full#supplementary-material
References
1. Broten TP, Romson JL, Fullerton DA, Van Winkle DM, Feigl EO. Synergistic action of myocardial oxygen and carbon dioxide in controlling coronary blood flow. Circ Res. (1991) 68:531–42. doi: 10.1161/01.RES.68.2.531
2. Guensch DP, Fischer K, Jung C, Hurni S, Winkler BM, Jung B, et al. Relationship between myocardial oxygenation and blood pressure: Experimental validation using oxygenation-sensitive cardiovascular magnetic resonance. PLoS ONE. (2019) 14:e0210098. doi: 10.1371/journal.pone.0210098
3. Clarke JRD, Kennedy R, Duarte Lau F, Lancaster GI, Zarich SW. Invasive evaluation of the microvasculature in acute myocardial infarction: coronary flow reserve versus the index of microcirculatory resistance. J Clin Med. (2019) 9:E86. doi: 10.3390/jcm9010086
4. Hellems HK, Ord JW, Talmers FN, Christensen RC. Effects of hypoxia on coronary blood flow and myocardial metabolism in normal human subjects. Circulation. (1957) 16:893–893.
5. Powers ER, Bannerman KS, Fitz-James I, Cannon PJ. Effect of elevations of coronary artery partial pressure of carbon dioxide (Pco2) on coronary blood flow. J Am Coll Cardiol. (1986) 8:1175–81. doi: 10.1016/S0735-1097(86)80398-9
6. Case RB, Greenberg H. The response of canine coronary vascular resistance to local alterations in coronary arterial P CO2. Circ Res. (1976) 39:558–66. doi: 10.1161/01.RES.39.4.558
7. McNulty PH, King N, Scott S, Hartman G, McCann J, Kozak M, et al. Effects of supplemental oxygen administration on coronary blood flow in patients undergoing cardiac catheterization. Am J Physiol Heart Circ Physiol. (2005) 288:H1057–62. doi: 10.1152/ajpheart.00625.2004
8. Leitch P, Hudson AL, Griggs JE, Stolmeijer R, Lyon RM. ter Avest E. Air ambulance kent surrey sussex. Incidence of hyperoxia in trauma patients receiving pre-hospital emergency anaesthesia: results of a 5-year retrospective analysis. Scand J Trauma, Resusc Emerg Med. (2021) 29:134. doi: 10.1186/s13049-021-00951-w
9. Konishi M, Akiyama E, Iwahashi N, Ebina T, Kimura K. Hypercapnia in acute heart failure patients with or without pulmonary edema. J Card Fail. (2014) 20:S168. doi: 10.1016/j.cardfail.2014.07.209
10. Ganz W, Donoso R, Marcus H, Swan HJC. Coronary hemodynamics and myocardial oxygen metabolism during oxygen breathing in patients with and without coronary artery disease. Circulation. (1972) 45:763–8. doi: 10.1161/01.CIR.45.4.763
11. Guensch DP, Fischer K, Shie N, Lebel J, Friedrich MG. Hyperoxia exacerbates myocardial ischemia in the presence of acute coronary artery stenosis in swine. Circ Cardiovasc Interv. (2015) 8:e002928. doi: 10.1161/CIRCINTERVENTIONS.115.002928
12. Dikmen Y, Onur A. Perioperative hyperoxia: perhaps a malady in disguise. Rom J Anaesth Intensive Care. (2017) 24:53–6. doi: 10.21454/rjaic.7518.241.yal
13. Stub D, Smith K, Bernard S, Nehme Z, Stephenson M, Bray JE, et al. Air versus oxygen in ST-segment elevation myocardial infarction. Circulation. (2015) 22:e114.014494. doi: 10.1161/CIRCULATIONAHA.115.019038
14. Moradkhan R, Sinoway LI. Revisiting the role of oxygen therapy in cardiac patients. J Am Coll Cardiol. (2010) 56:1013–6. doi: 10.1016/j.jacc.2010.04.052
15. Spoelstra-de Man AME, Smit B, Oudemans-van Straaten HM, Smulders YM. Cardiovascular effects of hyperoxia during and after cardiac surgery. Anaesthesia. (2015) 70:1307–19. doi: 10.1111/anae.13218
16. Wang CH, Chang WT, Huang CH, Tsai MS Yu PH, Wang AY, Chen NC, et al. The effect of hyperoxia on survival following adult cardiac arrest: a systematic review and meta-analysis of observational studies. Resuscitation. (2014) 85:1142–8. doi: 10.1016/j.resuscitation.2014.05.021
17. Cornet AD, Kooter AJ, Peters MJ, Smulders YM. The potential harm of oxygen therapy in medical emergencies. Crit Care. (2013) 17:313. doi: 10.1186/cc12554
18. Beaudin AE, Brugniaux JV, Vöhringer M, Flewitt J, Green JD, Friedrich MG, et al. Cerebral and myocardial blood flow responses to hypercapnia and hypoxia in humans. Am J Physiol Heart Circ Physiol. (2011) 301:H1678–86. doi: 10.1152/ajpheart.00281.2011
19. Fischer K, Guensch DP, Shie N, Lebel J, Friedrich MG. Breathing maneuvers as a vasoactive stimulus for detecting inducible myocardial ischemia–an experimental cardiovascular magnetic resonance study. PLoS ONE. (2016) 11:e0164524. doi: 10.1371/journal.pone.0164524
20. Pelletier-Galarneau M, deKemp RA, Hunter CRRN, Klein R, Klein M, Ironstone J, et al. Effects of hypercapnia on myocardial blood flow in healthy human subjects. J Nucl Med. (2018) 59:100–6. doi: 10.2967/jnumed.117.194308
21. Fischer K, Guensch DP, Friedrich MG. Response of myocardial oxygenation to breathing manoeuvres and adenosine infusion. Eur Heart J Cardiovasc Imaging. (2015) 16:395–401. doi: 10.1093/ehjci/jeu202
22. Kastrup A, Li TQ, Takahashi A, Glover GH, Moseley ME. Functional magnetic resonance imaging of regional cerebral blood oxygenation changes during breath holding. Stroke. (1998) 29:2641–5. doi: 10.1161/01.STR.29.12.2641
23. Liu H o L, Huang J u C, Wu CT e, Hsu YY. Detectability of blood oxygenation level-dependent signal changes during short breath hold duration. Magn Reson Imaging. (2002) 20:643–8. doi: 10.1016/S0730-725X(02)00595-7
24. Teixeira T, Hafyane T, Stikov N, Akdeniz C, Greiser A, Friedrich MG. Comparison of different cardiovascular magnetic resonance sequences for native myocardial T1 mapping at 3T. J Cardiovasc Magn Reson. (2016) 18:65. doi: 10.1186/s12968-016-0286-6
25. Fischer K, Neuenschwander MD, Jung C, Hurni S, Winkler BM, Huettenmoser SP, et al. Assessment of myocardial function during blood pressure manipulations using feature tracking cardiovascular magnetic resonance. Front Cardiovasc Med. (2021) 8:1353. doi: 10.3389/fcvm.2021.743849
26. Bakdash JZ, Marusich LR. Repeated measures correlation. Front Psychol. (2017) 8:456. doi: 10.3389/fpsyg.2017.00456
27. Hardie JA, Vollmer WM, Buist AS, Ellingsen I, Mørkve O. Reference values for arterial blood gases in the elderly. Chest. (2004) 125:2053–60. doi: 10.1378/chest.125.6.2053
28. Arteaga DF, Strother MK, Faraco CC, Jordan LC, Ladner TR, Dethrage LM, et al. The vascular steal phenomenon is an incomplete contributor to negative cerebrovascular reactivity in patients with symptomatic intracranial stenosis. J Cereb Blood Flow Metab. (2014) 34:1453–62. doi: 10.1038/jcbfm.2014.106
29. Banik S, Fisher JA, McKetton L, Venkatraghavan L. Mapping intracerebral steal during a hypercapnic challenge. Can J Anaesth. (2017) 64:1265–6. doi: 10.1007/s12630-017-0940-y
30. Fisher JA, Venkatraghavan L, Mikulis DJ. Magnetic resonance imaging-based cerebrovascular reactivity and hemodynamic reserve. Stroke. (2018) 49:2011–8. doi: 10.1161/STROKEAHA.118.021012
31. Riddell Z, Pressler N, Siau K, Mulder CJJ, Shalmani HM, Downs A, et al. Feasibility of high-flow nasal oxygen therapy and two-stage sedation during endoscopic hypopharyngeal therapy. JGH Open. (2020) 4:743–8. doi: 10.1002/jgh3.12348
32. Riva T, Theiler L, Jaquet Y, Giger R, Nisa L. Early experience with high-flow nasal oxygen therapy (HFNOT) in pediatric endoscopic airway surgery. Int J Pediatr Otorhinolaryngol. (2018) 108:151–4. doi: 10.1016/j.ijporl.2018.02.035
33. Booth A, Vidhani K, Lee P, Coman S, Pelecanos A, Dimeski G, Sturgess D. The effect of high-flow nasal oxygen on carbon dioxide accumulation in apneic or spontaneously breathing adults during airway surgery: a randomized-controlled trial. Anesth Analg. (2021) 133:133–41. doi: 10.1213/ANE.0000000000005002
34. Fischer K, Ranjan R, Friess JO, Erdoes G, Mikasi J, Baumann R, et al. Study design for a randomized crossover study investigating myocardial strain analysis in patients with coronary artery disease at hyperoxia and normoxemia prior to coronary artery bypass graft surgery (StrECHO-O2). Contemp Clin Trials. (2021) 110:106567. doi: 10.1016/j.cct.2021.106567
35. Grocott BB, Kashani HH, Maakamedi H, Dutta V, Hiebert B, Rakar M, et al. Oxygen management during cardiopulmonary bypass: a single-center, 8-year retrospective cohort study. J Cardiothorac Vasc Anesth. (2021) 35:100–5. doi: 10.1053/j.jvca.2020.08.029
36. Morkane CM, McKenna H, Cumpstey AF, Oldman AH, Grocott MPW, Martin DS, et al., Pan London Perioperative Audit and Research Network (PLAN), South Coast Perioperative Audit and Research Collaboration (SPARC). Intraoperative oxygenation in adult patients undergoing surgery (iOPS): a retrospective observational study across 29 UK hospitals. Perioper Med. (2018) 7:17. doi: 10.1186/s13741-018-0106-7
37. Ibanez B, James S, Agewall S, Antunes MJ, Bucciarelli-Ducci C, Bueno H, et al. 2017 ESC Guidelines for the management of acute myocardial infarction in patients presenting with ST-segment elevationThe Task Force for the management of acute myocardial infarction in patients presenting with ST-segment elevation of the European Society of Cardiology (ESC). Eur Heart J. (2018) 39:119–77. doi: 10.1093/eurheartj/ehx393
38. O'Driscoll BR, Howard LS, Earis J, Mak V. British thoracic society guideline for oxygen use in adults in healthcare and emergency settings. BMJ Open Respir. (2017) 4:e000170. doi: 10.1136/bmjresp-2016-000170
39. Applegate RL, Dorotta IL, Wells B, Juma D, Applegate PM. The relationship between oxygen reserve index and arterial partial pressure of oxygen during surgery. Anesth Analg. (2016) 123:626–33. doi: 10.1213/ANE.0000000000001262
40. Coleman MD. Chapter 2–respiratory and pulmonary physiology A2–duke, James. In: Anesthesia Secrets. 4th, ed. Philadelphia: Mosby (2011). p. 17–23.
41. Guensch DP, Fischer K, Flewitt JA, Friedrich MG. Myocardial oxygenation is maintained during hypoxia when combined with apnea–a cardiovascular MR study. Physiol Rep. (2013) 1:e00098. doi: 10.1002/phy2.98
42. Damiani E, Casarotta E, Orlando F, Carsetti A, Scorcella C, Domizi R, et al. Effects of normoxia, hyperoxia, and mild hypoxia on macro-hemodynamics and the skeletal muscle microcirculation in anesthetised rats. Front Med. (2021) 8:672257. doi: 10.3389/fmed.2021.672257
43. Yang W, Zhang X, Wang N, Tan J, Fang X, Wang Q, et al. Effects of acute systemic hypoxia and hypercapnia on brain damage in a rat model of hypoxia-ischemia. PLoS ONE. (2016) 11:e0167359. doi: 10.1371/journal.pone.0167359
44. Bill KM. Anaesthesia for patients with cardiac disease undergoing non-cardiac surgery. Anaesth Intensive Care Med. (2009) 10:446–50. doi: 10.1016/j.mpaic.2009.06.010
45. Fischer K, Yamaji K, Luescher S, Ueki Y, Jung B, von Tengg-Kobligk H, et al. Feasibility of cardiovascular magnetic resonance to detect oxygenation deficits in patients with multi-vessel coronary artery disease triggered by breathing maneuvers. J Cardiovasc Magn Reson. (2018) 20:31. doi: 10.1186/s12968-018-0446-y
46. Werner GS, Fritzenwanger M, Prochnau D, Schwarz G, Ferrari M, et al. Determinants of coronary steal in chronic total coronary occlusions. J Am Coll Cardiol. (2006) 48:51–8. doi: 10.1016/j.jacc.2005.11.093
47. Pedersen SS, Holse C, Mathar CE, Chan MTV, Sessler DI, Liu Y, et al. Intraoperative inspiratory oxygen fraction and myocardial injury after noncardiac surgery: results from an international observational study in relation to recent controlled trials. Anesth Analg. (2022) 135. 1021-1030. doi: 10.1213/ANE.0000000000006042
48. Page D, Ablordeppey E, Wessman BT, Mohr NM, Trzeciak S, Kollef MH, et al. Emergency department hyperoxia is associated with increased mortality in mechanically ventilated patients: a cohort study. Crit Care. (2018) 22:9. doi: 10.1186/s13054-017-1926-4
49. Guensch DP, Fischer K, Yamaji K, Luescher S, Ueki Y, Jung B, et al. Effect of hyperoxia on myocardial oxygenation and function in patients with stable multivessel coronary artery disease. J Am Heart Assoc. (2020) 9:e014739. doi: 10.1161/JAHA.119.014739
50. Smit B, Smulders YM, van der Wouden JC. Oudemans-van Straaten HM, Spoelstra-de Man AME. Hemodynamic effects of acute hyperoxia: systematic review and meta-analysis. Crit Care. (2018) 22:45. doi: 10.1186/s13054-018-1968-2
51. Broten TP, Feigl EO. Role of myocardial oxygen and carbon dioxide in coronary autoregulation. Am J Physiol Heart Circ Physiol. (1992) 262:H1231–7. doi: 10.1152/ajpheart.1992.262.4.H1231
52. Crystal GJ. Carbon dioxide and the heart: physiology and clinical implications. Anesth Analg. (2015) 121:610–23. doi: 10.1213/ANE.0000000000000820
53. Foster GE, Sheel AW. The human diving response, its function, and its control. Scand J Med Sci Spor. (2005) 15:3–12. doi: 10.1111/j.1600-0838.2005.00440.x
54. Sasse SA, Berry RB, Nguyen TK, Light RW, Mahutte CK. Arterial blood gas changes during breath-holding from functional residual capacity. Chest. (1996) 110:958–64. doi: 10.1378/chest.110.4.958
55. Farquhar H, Weatherall M, Wijesinghe M, Perrin K, Ranchord A, Simmonds M, et al. Systematic review of studies of the effect of hyperoxia on coronary blood flow. Am Heart J. (2009) 158:371–7. doi: 10.1016/j.ahj.2009.05.037
56. Wyss CA, Koepfli P, Fretz G, Seebauer M, Schirlo C, Kaufmann PA. Influence of altitude exposure on coronary flow reserve. Circulation. (2003) 108:1202–7. doi: 10.1161/01.CIR.0000087432.63671.2E
Keywords: coronary blood flow, blood gases, hyperoxia, oxygen, carbon dioxide, animal model
Citation: Fischer K, Guensch DP, Shie N, Nadeshalingham G, Lebel J and Friedrich MG (2022) Altered blood gas tensions of oxygen and carbon dioxide confound coronary reactivity to apnea. Front. Anesthesiol. 1:997836. doi: 10.3389/fanes.2022.997836
Received: 19 July 2022; Accepted: 09 September 2022;
Published: 30 November 2022.
Edited by:
Giovanni Landoni, San Raffaele Hospital (IRCCS), ItalyReviewed by:
Gianluca Paternoster, Department of Cardiothoracic Anesthesia and ICU San Carlo Hospital Potenza Italy, ItalySoumen De, University of Calcutta, India
Jun Hong Park, Korea Institute of Science and Technology, South Korea
Copyright © 2022 Fischer, Guensch, Shie, Nadeshalingham, Lebel and Friedrich. This is an open-access article distributed under the terms of the Creative Commons Attribution License (CC BY). The use, distribution or reproduction in other forums is permitted, provided the original author(s) and the copyright owner(s) are credited and that the original publication in this journal is cited, in accordance with accepted academic practice. No use, distribution or reproduction is permitted which does not comply with these terms.
*Correspondence: Kady Fischer, kady.fischer@insel.ch