Seasonal drivers of productivity and calcification in the coral Platygyra carnosa in a subtropical reef
- 1State Key Laboratory of Marine Pollution, City University of Hong Kong, Kowloon, Hong Kong SAR, China
- 2Department of Biomedical Sciences, City University of Hong Kong, Kowloon, Hong Kong SAR, China
- 3Department of Biology, Hong Kong Baptist University, Kowloon, Hong Kong SAR, China
- 4Marine Science Laboratory, Chinese University of Hong Kong, New Territories, Hong Kong SAR, China
- 5Southern Marine Science and Engineering Guangdong Laboratory, Guangzhou, China
- 6The Swire Institute of Marine Science, School of Biological Sciences, University of Hong Kong, Hong Kong, Hong Kong SAR, China
- 7School of Marine Sciences, University of Maine, Orono, ME, United States
- 8State Key Laboratory of Satellite Ocean Environment Dynamics, Second Institute of Oceanography, Hangzhou, China
Scleractinian corals are increasingly subjected to local stressors combined with global changes. In subtropical areas, corals exhibit metabolic plasticity and resilience in response to variability and extremes in local temperature, salinity, and light; however, the physiological mechanisms by which corals acclimate or adapt to these changing conditions remain disputed. We assessed the physiological status of the coral Platygyra carnosa during a two-year in situ monitoring survey. To obtain metabolic rates (respiration and photosynthesis), photochemical efficiency (Fv / Fm), and biocalcification measurements, non-invasive techniques such as underwater respirometry, Pulse Amplitude Modulated (PAM) fluorometry, total alkalinity measurements, and digital photography were used. Our findings show clear seasonality in water quality parameters, which affected coral health. Elevated temperatures during the summer were below the maximum monthly mean < 31°C) but reduced the energetic productivity of corals (-44% relative to winter). Fluctuations in salinity (25–38 ppt) and pH (7.65–8.44) were linked to rainfall and reduced calcification rates. The conditions during the spring were favorable for coral metabolism and calcification (+20% relative to summer). Overall, our research demonstrates that the metabolic plasticity of P. carnosa in response to shifts in seawater quality allows this species to survive ongoing environmental change. Our in situ observations provide fundamental insights into coral response mechanisms under changing environmental conditions and contribute to projections of coral health under future scenarios of global change.
1. Introduction
Global climate change is causing oceans to become warmer and with reduced seawater pH which is having a major impact on marine ecosystems (Keppel et al., 2012). These changes are especially pronounced in tropical reefs, which experience annual thermal perturbations with compounding effects from increasing acidification (Hoegh-Guldberg et al., 2007; Hoegh-Guldberg et al. 2017). Subtropical reefs have evolved under fluctuating pH (Yuan et al., 2019), and thermal conditions (Brown et al., 2000; Lee et al., 2020). However, reefs located across a wide range of latitudes are believed to be at their physiological threshold limit for reef development, recruitment success, and coral survival (Kleypas et al., 1999; Beger et al., 2014; Chui et al., 2016; Camp et al., 2018).
Extreme temperature events are increasing in frequency and severity (Hobday et al., 2016; Wyatt et al., 2020). A metabolic threshold limit has been observed for corals when seawater temperature exceeds the mean monthly maximum temperature by 1°C (Dias et al., 2019; Genevier et al., 2019; Silbiger et al., 2019; Dellisanti et al., 2020a), although it is species- and location-specific. Seasonal changes in coral metabolism are related to lower daily energetic productivity and reduced coral growth in summertime compared to winter (Roik et al., 2015; Vajed Samiei et al., 2015). In addition, the impacts of ocean acidification resulting from increased dissolution of anthropogenic CO2 in seawater (Hoegh-Guldberg et al., 2007; Kroeker et al., 2010) leads to depleted availability of , essential for the calcification process (Dove et al., 2013; Anthony, 2016; Comeau et al., 2019). However, modest increase in pCO2 levels can have the contrasting effect of stimulating greater primary production and calcification rates in corals (Castillo et al., 2014; Noonan et al., 2018; Biscéré et al., 2019), increase the oxidative stress of endosymbionts (Soriano-Santiago et al., 2013), or mitigate warming effects (Pitts et al., 2020). In parallel to abrupt changes of global climate conditions expected by 2030 (Trisos et al., 2020), increasing local stressors, such as sedimentation (Browne et al., 2014), algal proliferations, and nutrient loads (Wooldridge and Done, 2009; Browne et al., 2015), can have combined effects on coral communities.
Coral communities inhabiting subtropical coastal areas continue to thrive in the face of multiple stressors, including extreme seasonal fluctuations of temperature, salinity, light intensity, sedimentation rates, and nutrient loading (Courtney et al., 2017; Cybulski et al., 2020). Nevertheless, multiple stressors may affect the species distributions of in-shore reefs and the relative delivery of ecosystem services (Costanza et al., 2014; Loiola et al., 2019; Jones et al., 2020), with consequences for metabolic adaptation (Jones et al., 2020; Roberty et al., 2020). In particular, the ability of the coral individuals to adjust to environmental changes is one of the key features of stress-resistant corals affected by human activities (Heery et al., 2018; Ng et al., 2020).
Many species in the subtropical region of the South China Sea (SCS) are considered to be stress-tolerant, typically being influenced by strong seasonal changes in environmental conditions between summer (wet season) and winter (dry season) including temperature and salinity (Ang et al., 2005). Our study focuses on in situ observations of the physiological status of a stress-tolerant coral living in Hong Kong, Platygyra carnosa (Veron, 2000), and its responses to environmental changes. Although an overall decline in marine diversity has been documented (Wong et al., 2018), P. carnosa remains abundant in the subtropical region of the SCS (Yeung et al., 2021). Here, we apply a novel assessment approach based on non-invasive in situ techniques combining an innovative respirometry system, fluorescence measurements, and photographic analysis to assess the metabolic response and energetics of P. carnosa under environmental fluctuations. We hypothesized that P. carnosa is able to modulate its metabolic rates according to seasonal conditions, with elevated temperature and reduced salinity acting as the main drivers of physiological stress. In particular, we expected reduced energetic productivity and biocalcification during the summer. Overall, our results provided new insights into the physiological status of this species and its responses to the current and future climate scenarios.
2. Materials and methods
2.1. Survey site and coral monitoring
Hong Kong is located in the tropical area of the South China Sea, with a subtropical seasonal monsoon-influenced climate characterized by a clear wet season (summer, July-September) and a dry season (winter, January-March) with transitional months in between (spring and autumn; Yin, 2003; Lee et al., 2006). A shallow (2-4 m depth) region was selected in Mirs Bay, Port Island (Hong Kong, 22.502° N – 114.356° E; Figure 1A), where P. carnosa colonies are abundant compared to other local species, although this site has a relatively low coral cover (14%; Yeung et al., 2021). Repeated measurements of coral health were conducted in situ on apparently healthy coral colonies randomly selected (n = 5 per month) using SCUBA surveys conducted between April 2018 and March 2020, as described in the subsequent sections. Physical, chemical, and coral metabolic data were collected on the same day and location at monthly intervals at mid-day (between 10:30 am and 1:30 pm).
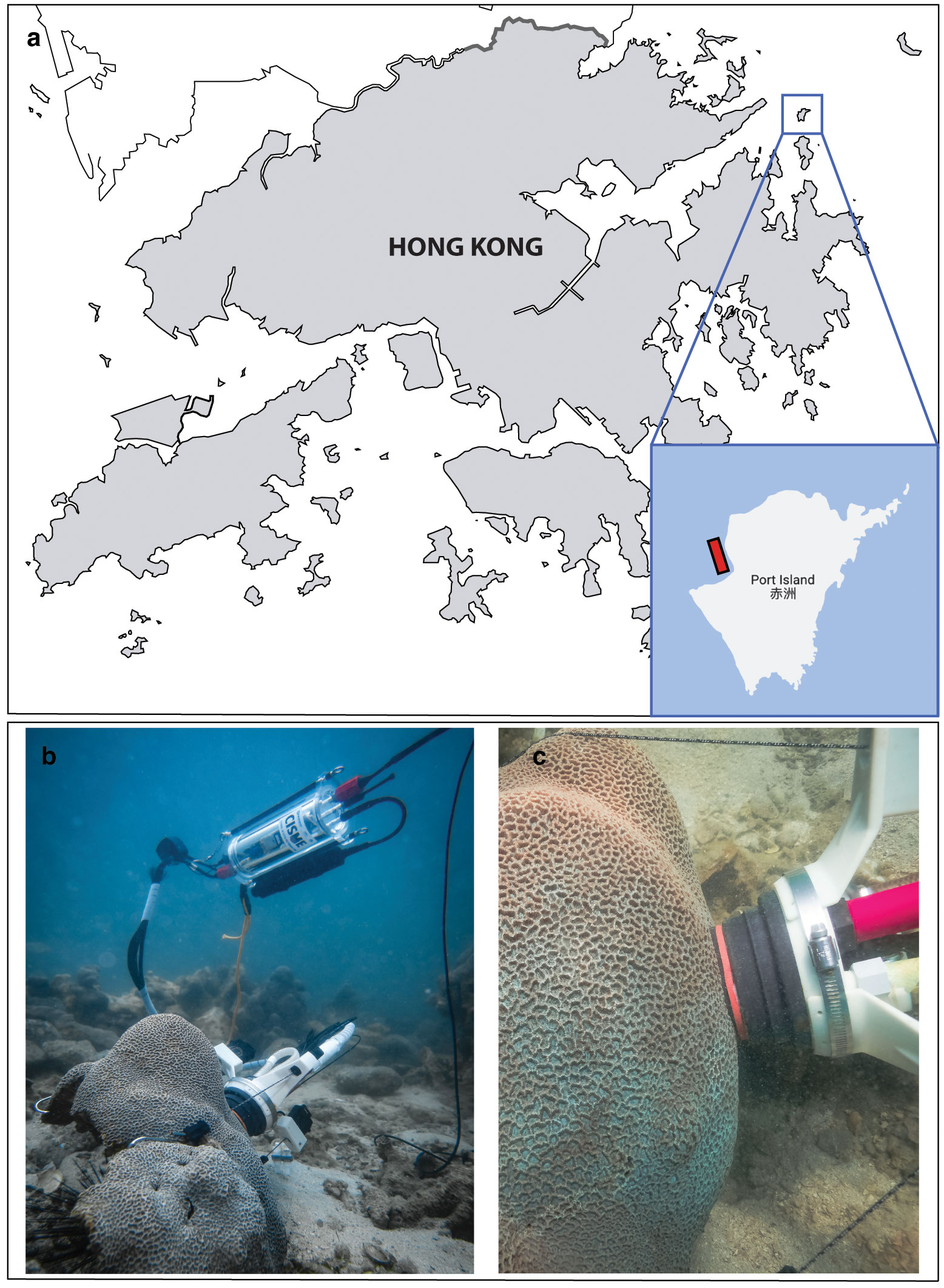
Figure 1 Location of the survey site in Mirs Bay, Port Island (Hong Kong). (A) The area where P. carnosa corals were found is highlighted in red; (B, C) show detailed images of the CISME underwater respirometer deployed on P. carnosa corals.
2.2. Physical and chemical parameters
Water column oceanographic parameters (temperature, salinity, pH, dissolved oxygen, chlorophyll-a, and turbidity) were monitored with a multi-parameter sonde (YSI EXO2 Water Quality Sonde) calibrated in the laboratory prior to each sampling day (Figure 2). pH was calibrated with National Bureau of Standards (NBS) buffers (pH 4.01, 7.00, and 10.06, Xylem Ltd.) at 25°C (Supplementary Information file).
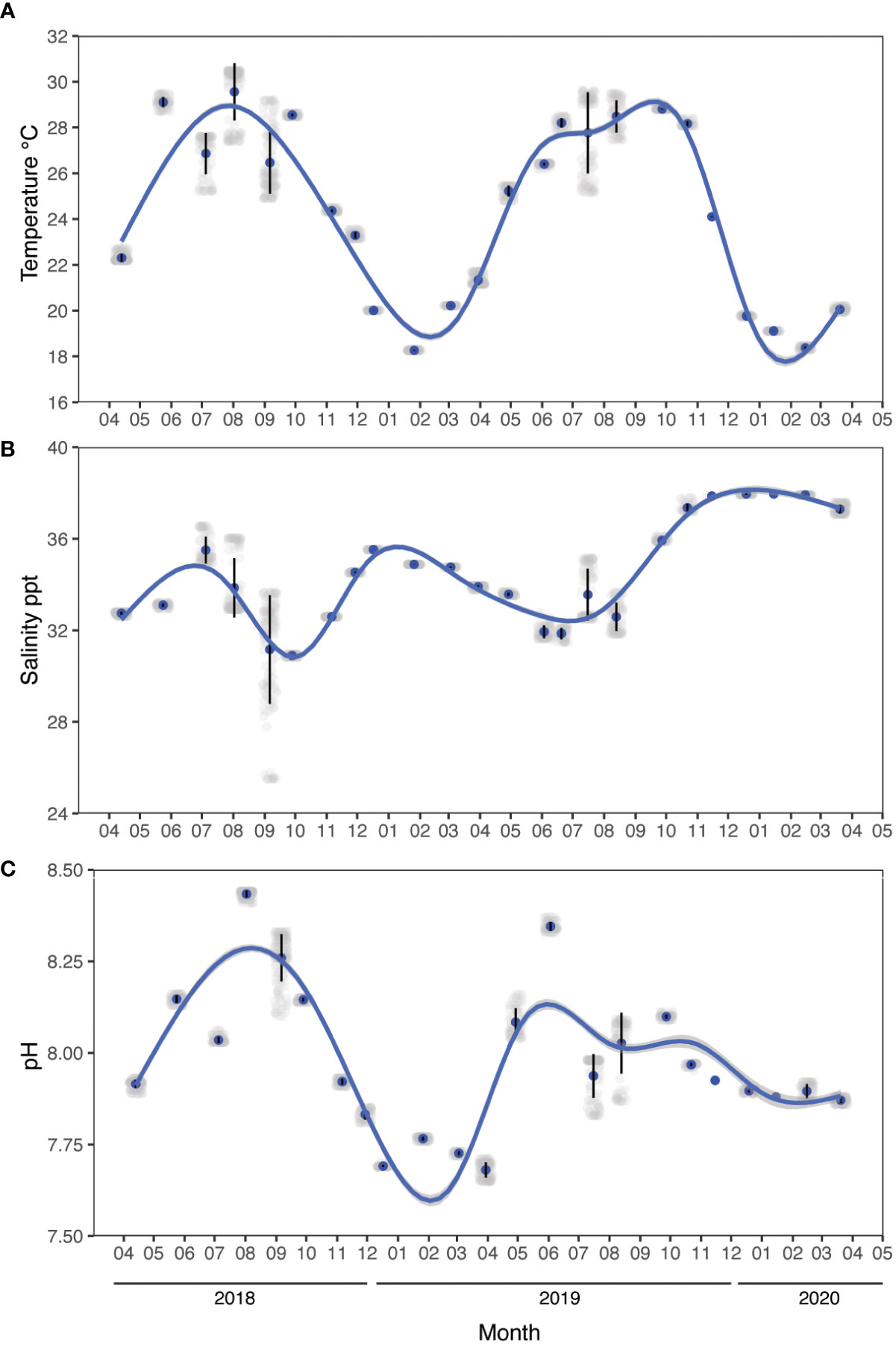
Figure 2 Environmental parameters measured in situ between April 2018 and March 2020. (A) Temperature, (B) salinity, and (C) pH. Data were recorded every month using a YSI multi-parameter sonde during daytime hours (between 10:30 am to 1:30 pm). Noise is added (jittering) for data visualization, the vertical lines indicate the standard error, and the blue line indicates a generalized additive model fit (ggplot2).
In situ light intensity, rainfall data (from the Tap Mun station), clouds cover, and evaporation (from the King’s Park station) were obtained from the Hong Kong Observatory of the Government of Hong Kong Special Administrative Region, China (www.hko.gov.hk; Supplementary Table 1).
Discrete seawater samples (500 mL, n = 5 per month) were collected at the same depth (2-4 m) beside the corals for the determination of total alkalinity (At) (Dickson et al., 2007). Samples were immediately treated with a saturated HgCl2 solution (0.08% final concentration) after collection and stored in the dark (+4°C) until the analysis.
2.3. In situ coral physiological measurements
Physiological parameters of five randomly selected P. carnosa (Figure 1B, C) were measured using the same protocols at each deployment survey, giving repeated observations of health status under natural conditions. Respiration (R) and net photosynthesis (Pn) rates were measured at the coral surfaces using an underwater respirometer (CISME Instruments LLC) following the measurement and calibration protocols as previously described by Dellisanti et al. (2020b). Similar settings were used to quantify the rate of oxygen consumption during dark incubation (5 min) and the subsequent rate of oxygen production by the photosynthetic endosymbionts under simulated natural light (10 min) in a continuously recirculating water chamber. The LED intensity in the chamber was adjusted to match the mid-day irradiance as measured at the coral-collection site (~460 μmol photons m−2 s−1). Gross photosynthesis (Pg) was calculated by adding the absolute value of R to Pn, quantified as the oxygen flux during the incubations. The holobiont productivity was quantified as the ratio of oxygen production through gross photosynthesis (Pg) to consumption through respiration (R), i.e., Pg/R (Figure 3A).
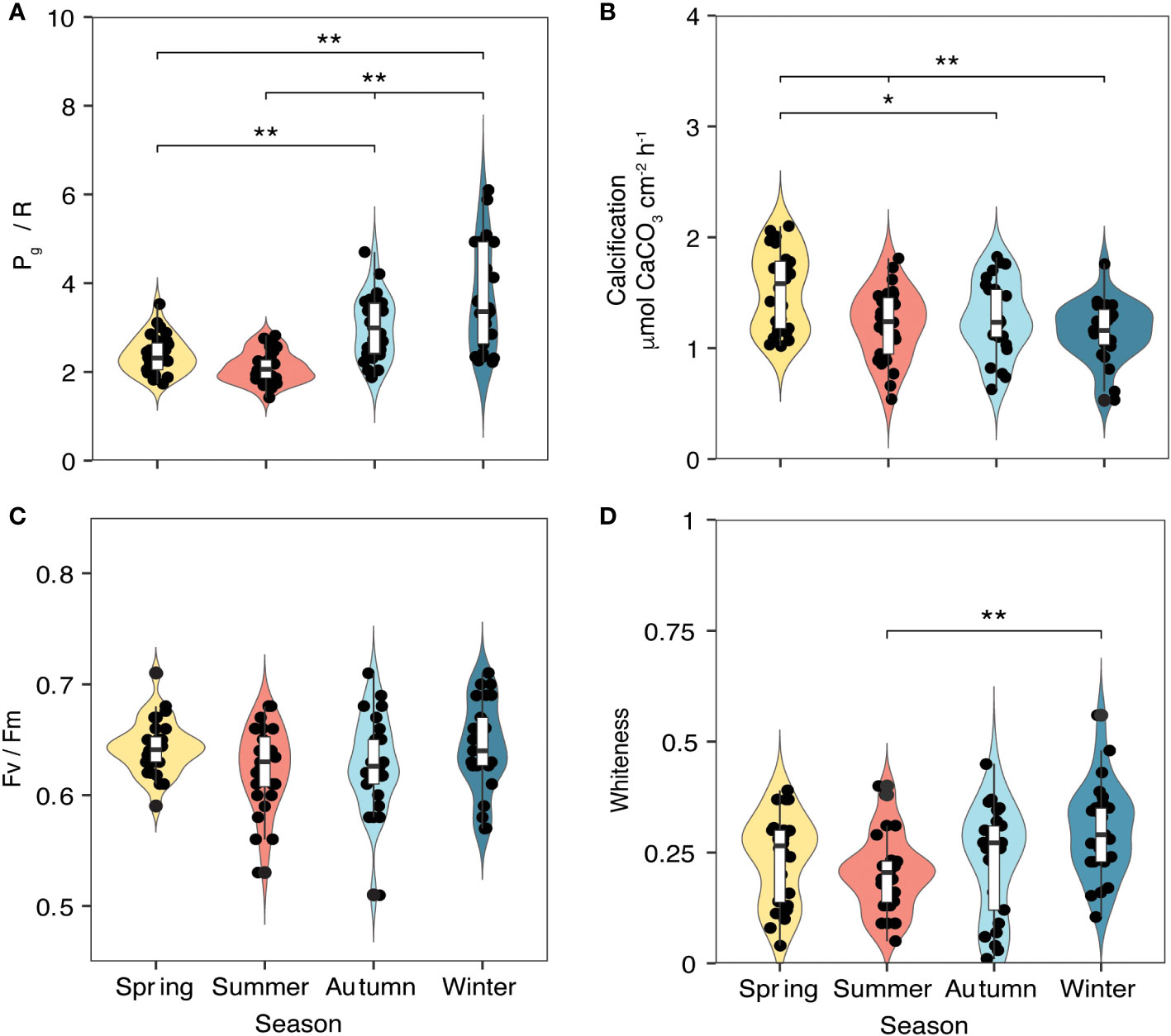
Figure 3 Metabolic parameters of P. carnosa corals (n = 5 per month) measured between April 2018 and March 2020 during daytime hours (between 10:30 am and 1:30 pm). (A) Pg/R ratio and (B) calcification (CA) were measured using a CISME device; (C) photosynthetic efficiency (Fv/Fm) was measured in the same area of the coral using a diving Pulse Amplitude Modulated (PAM) fluorometer; and (D) whiteness was calculated based on photographic analysis. Significance codes: ** p< 0.01; * p< 0.05; non-significant effects are not shown.
At the end of each light incubation period, a sample of the recirculated chamber water (20 mL) was collected for the measurement of At. Rates of coral calcification (CA; Figure 3B) were determined using the alkalinity anomaly technique (Dickson et al., 2007) as follows:
where ΔAt (μmol kg-1) is the difference in At between seawater and the recirculation loop, V is the total volume of the chamber (0.088 L), r is the seawater density (~1.023 g cm-3), t is the incubation time (15 min), and S is the coral surface area investigated (24.5 cm2).
The photosynthetic capacity of the coral symbionts was measured as the maximum quantum yield (Fv/Fm) using a Pulse Amplitude Modulated (PAM) fluorometer (diving-PAM, Heinz Walz, Effeltrich, Germany) equipped with a standard glass-fiber optic probe (Ralph et al., 1999). The diving-PAM settings for “Measuring Light Intensity” were set to 7, “Damping” to 3, “Gain” to 12, “Saturation-Pulse Intensity” to 12, and “Saturating width” to 2.0 s (Dellisanti et al., 2020a). PAM measurements were obtained from randomly selected polyps (n = 6) in the same area of the coral where the CISME device was deployed, after 15 min dark acclimation (Figure 3C).
Finally, digital photographs were taken of the same coral areas for colorimetric analysis to quantify whiteness levels as a measure of discoloration/bleaching (Chow et al., 2016). The whiteness values were obtained as the dissimilarity percentage in color composition using the SIMPER tool of Primer 6.0 software (Primer-E Ltd, Figure 3D).
2.4. Data analysis
All data were grouped into the following four groups: spring (April to June), summer (July to September), autumn (October to December), and winter (January to March; Yin, 2003; Lee et al., 2006). Data transformation (square-root) and normalization was performed prior to the multivariate analysis. Seasonal differences in coral physiology were evaluated using a one-way analysis of variance (ANOVA) with a post-hoc Tukey HSD t-test between seasons. The environmental parameters were compared using a two-way permutational multivariate analysis of variance (PERMANOVA) with repeated measures based on Euclidean distances and visualized using a principal coordinate analysis (PCoA). To evaluate the effect of environmental variables measured in situ on coral physiological measurements, a distance-based linear model (DistLM) was used. The correlation between coral physiology and environmental variables was then explored using Spearman’s rank correlation, visualized with using a correlogram (two-tailed significance = 95%). Thermal performance curves (TPCs) were modeled against the average seawater temperature as measured during the data-collection period. For each physiological trait, we applied four biologically relevant functions (Gaussian, exponentially modified Gaussian, Weibull, and lognormal; Angilletta, 2006) and a linear model, and determined the best fit with the corrected Akaike Information Criterion (AICc). Results were considered significant where p< 0.05. All analyses were performed with RStudio v.1.3.1093 (2020), and PRIMER v.6 with PERMANOVA+ (Anderson et al., 2008).
3. Results
3.1. In situ environmental conditions
The water conditions measured in the water column at the study site exhibited clear fluctuations in physical and chemical parameters between seasons (Figure 2, Table 1). Mean seawater temperature ranged from 28.29 ± 1.08°C in summer to 19.55 ± 1.19°C in winter (Figure 2A), with a maximum of 30.5°C recorded in August 2018 at the sea surface level and a minimum of 18.25°C in January 2019 below a depth of 3 m. Salinity ranged from 32.99 ± 1.87 ppt in summer to 36.12 ± 1.8 ppt in winter, with a peak of 38.01 ppt observed in December 2019 at 5.5 m depth and a minimum of 25.51 ppt in August 2018 at the sea surface level (Figure 2B). Seawater pH ranged from 8.09 ± 0.11 in spring to 7.81 ± 0.09 in winter, with a maximum of 8.44 in August 2018 at a depth of 0.5 – 4.8 meters depth and a minimum of 7.65 in March 2019 at the sea surface level (Figure 2C). Additional data on seawater quality provided in the Supplementary Information file.
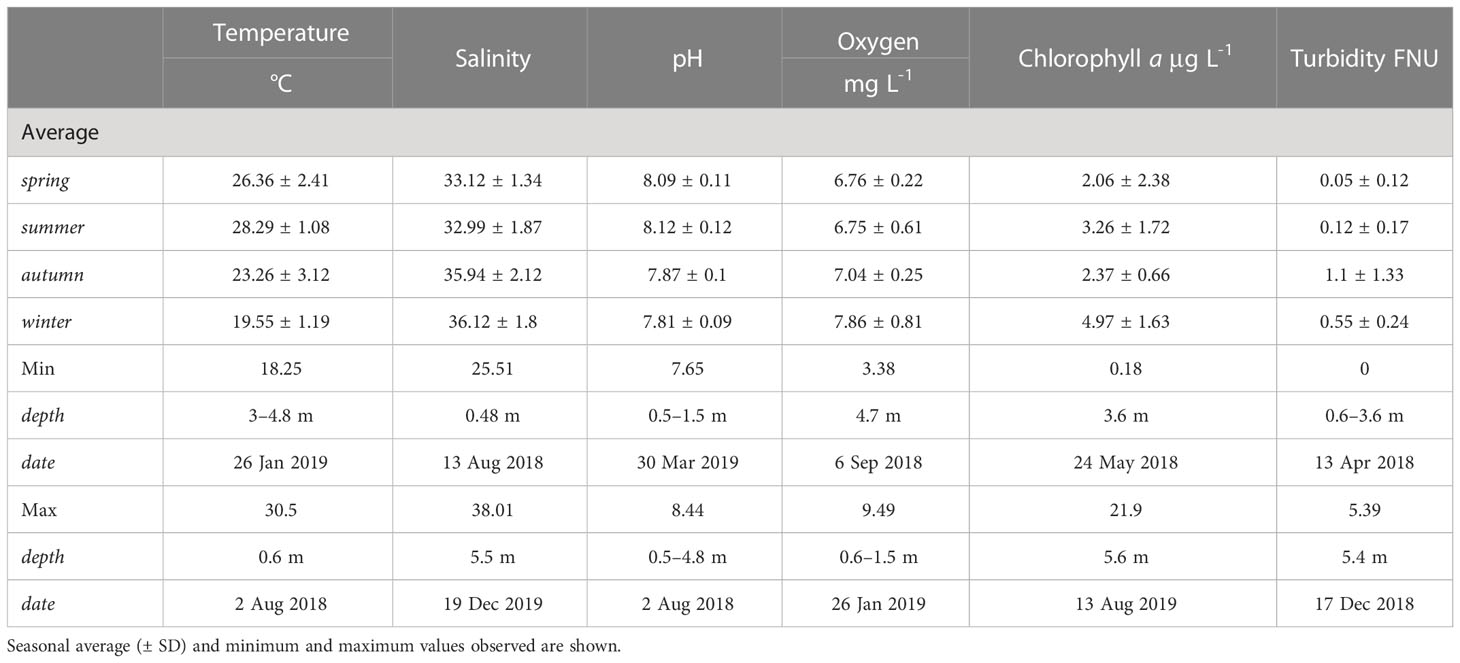
Table 1 Environmental parameters (temperature, salinity, pH, oxygen, chlorophyll-a, and turbidity) measured using a YSI EXO2 device within the water column at the study site in Mirs Bay, Port Island, Hong Kong.
3.2. Coral physiological measurements
The marked seasonal fluctuations in the environmental parameters influenced the coral physiological status during the period of study (Figure 3, Table 2). Pg/R varied significantly (ANOVA, F = 22.9, p< 0.01), with higher values during the winter (3.77 ± 1.27) and lower values during the summer (2.11 ± 0.17; Figure 3A). Calcification rates also showed significant differences among seasons (ANOVA, F = 6.93, p< 0.01), with lower rates in winter (1.16 ± 0.24 μmol CaCO3 cm-2 h-1) than in spring (1.53 ± 0.35 μmol CaCO3 cm-2 h-1, p< 0.01; Figure 3B). No significant seasonal differences in photosynthetic efficiency (Fv/Fm) were observed (Figure 3C). The whiteness values of the corals also varied significantly between seasons (ANOVA, F = 4.021, p< 0.01), with a summer value 0.21 ± 0.03%, a winter value of 0.29 ± 0.07% (Figure 3D). The ANOVA results for the coral metabolic parameters and the associated Tukey HSD test between the seasons are provided in Supplementary Table 2.
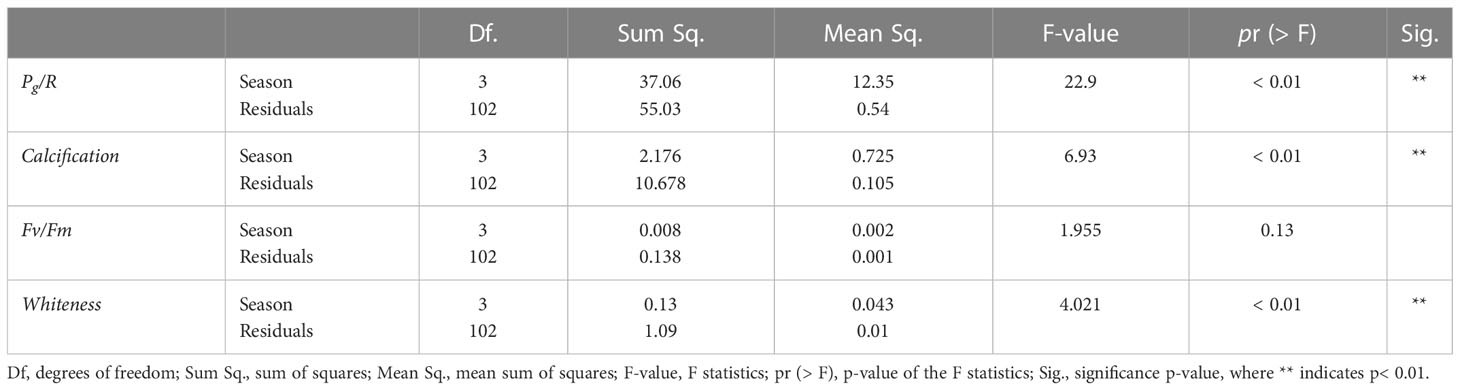
Table 2 Analysis of variance (ANOVA) for coral metabolic parameters and a Tukey HSD test between the means of the “Season” groups.
3.3. Multivariate analysis of environmental conditions on coral metabolism
The results of the PERMANOVA (permutations n = 9,999) indicated a clear seasonality in water-quality conditions (Figure 4; p< 0.01), although no differences were observed between spring and summer (Table 3). Thus, we investigated the influence of environmental conditions on coral metabolism to determine those factors that had the greatest effect on the health status of P. carnosa. Among all the variables analyzed, temperature was significantly associated with the health of P. carnosa during the study period (p< 0.01, proportion of variance = 19.5%; Table 4).
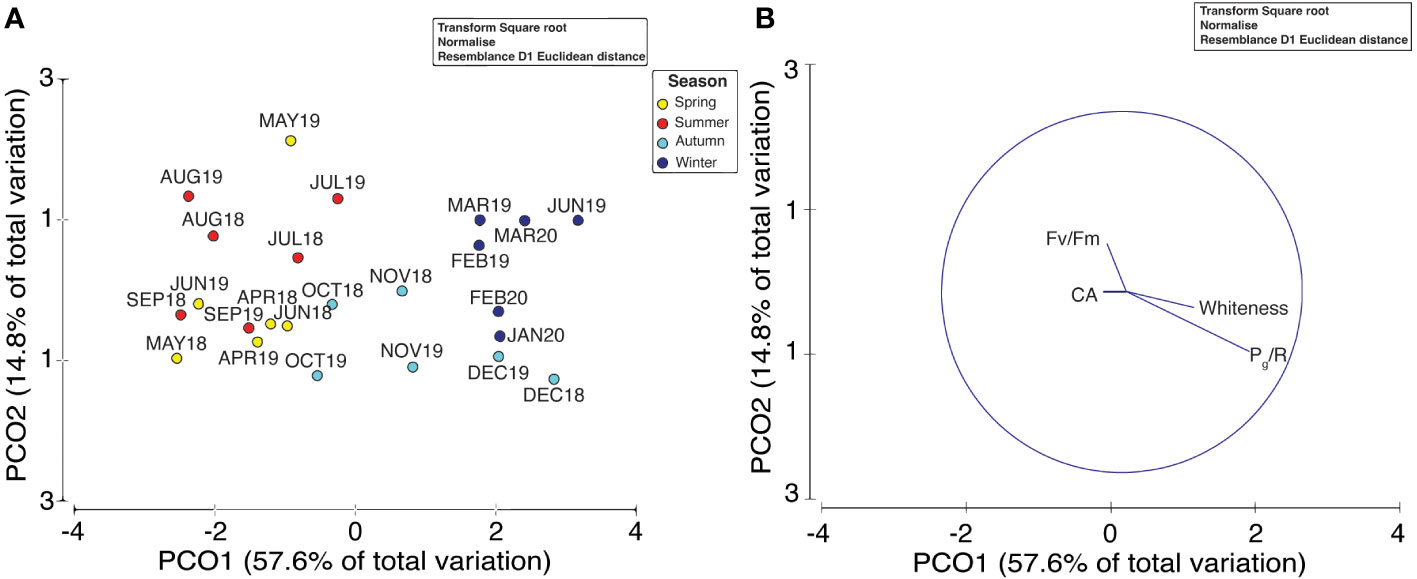
Figure 4 Principal coordinates analysis (PCoA) of multivariate environmental data (A) and vectors of coral metabolic parameters (B) collected during the two-year survey. Distances between each point are based on Euclidean distances with “Season” as the factor.
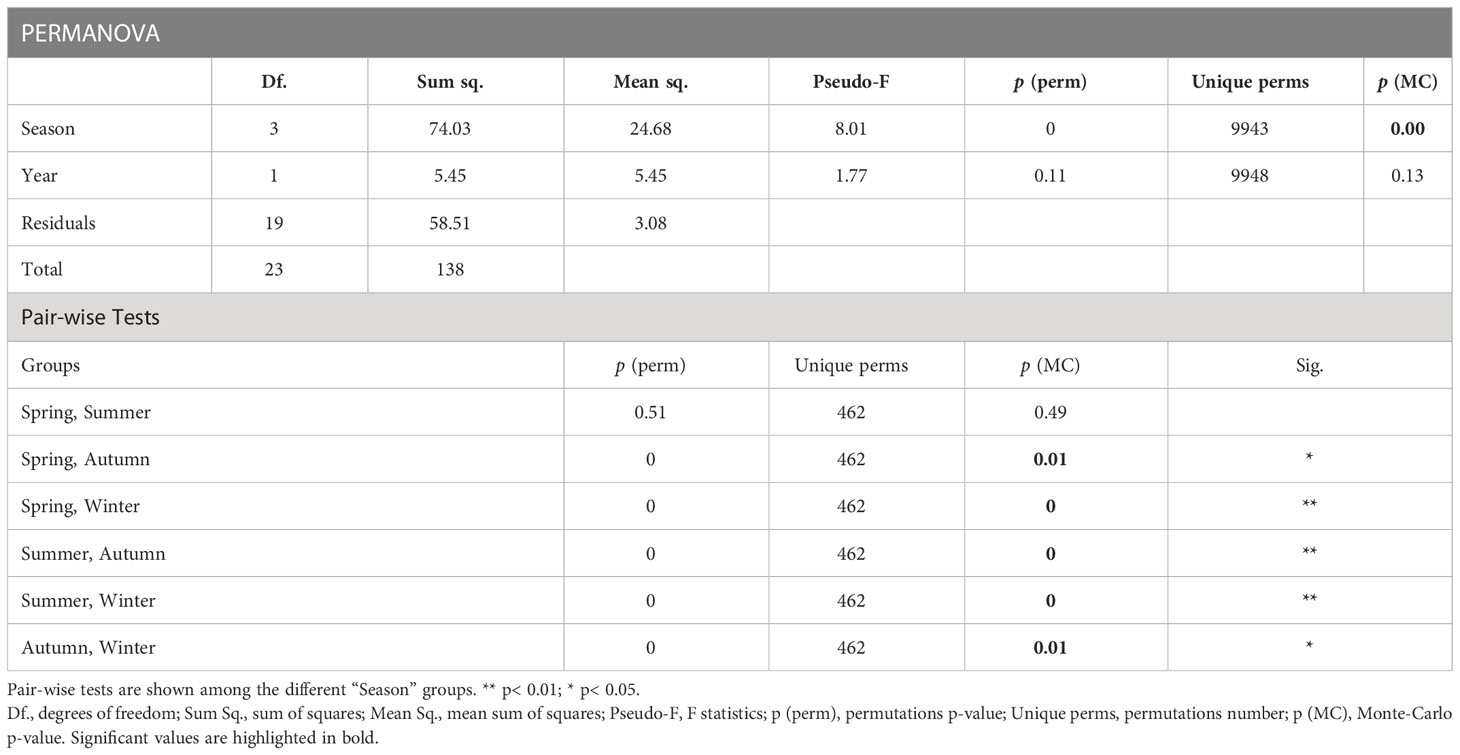
Table 3 Permutational multivariate analysis of variance (PERMANOVA) with repeated measures on Euclidean distances for environmental parameters analyzed.
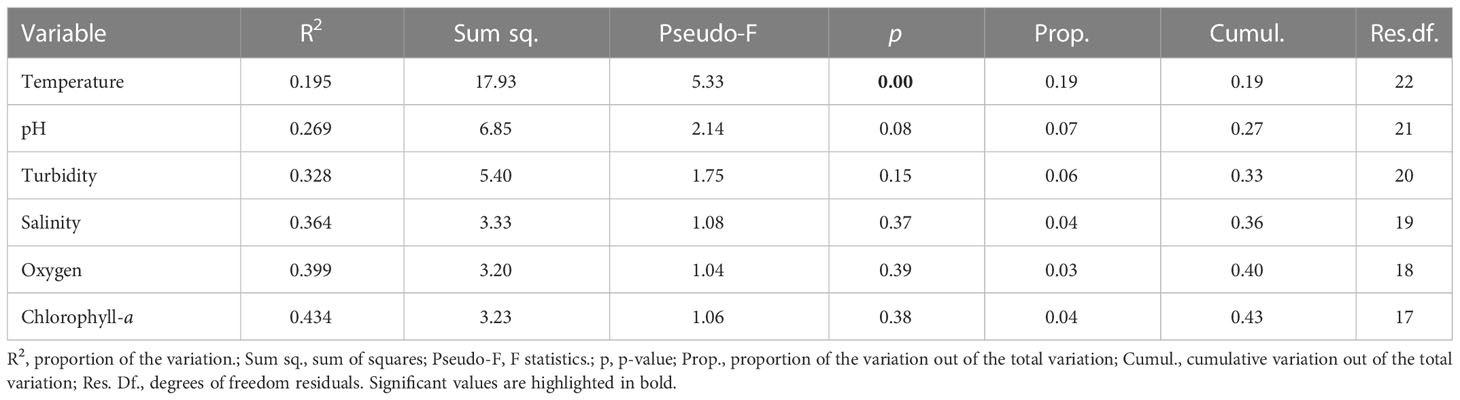
Table 4 Results of a distance-based linear model (DistLM) of the effect of environmental variables on coral metabolic parameters.
A correlogram of all environmental and coral metabolic variables based on Spearman’s rank correlation coefficients is shown in Figure 5. The temperature variation was negatively correlated with Pg/R (r = - 0.74, p< 0.05). Moreover, the pH was negatively associated with both Pg/R and whiteness levels (r = - 0.48, p< 0.05; r = - 0.55, p< 0.05, respectively).
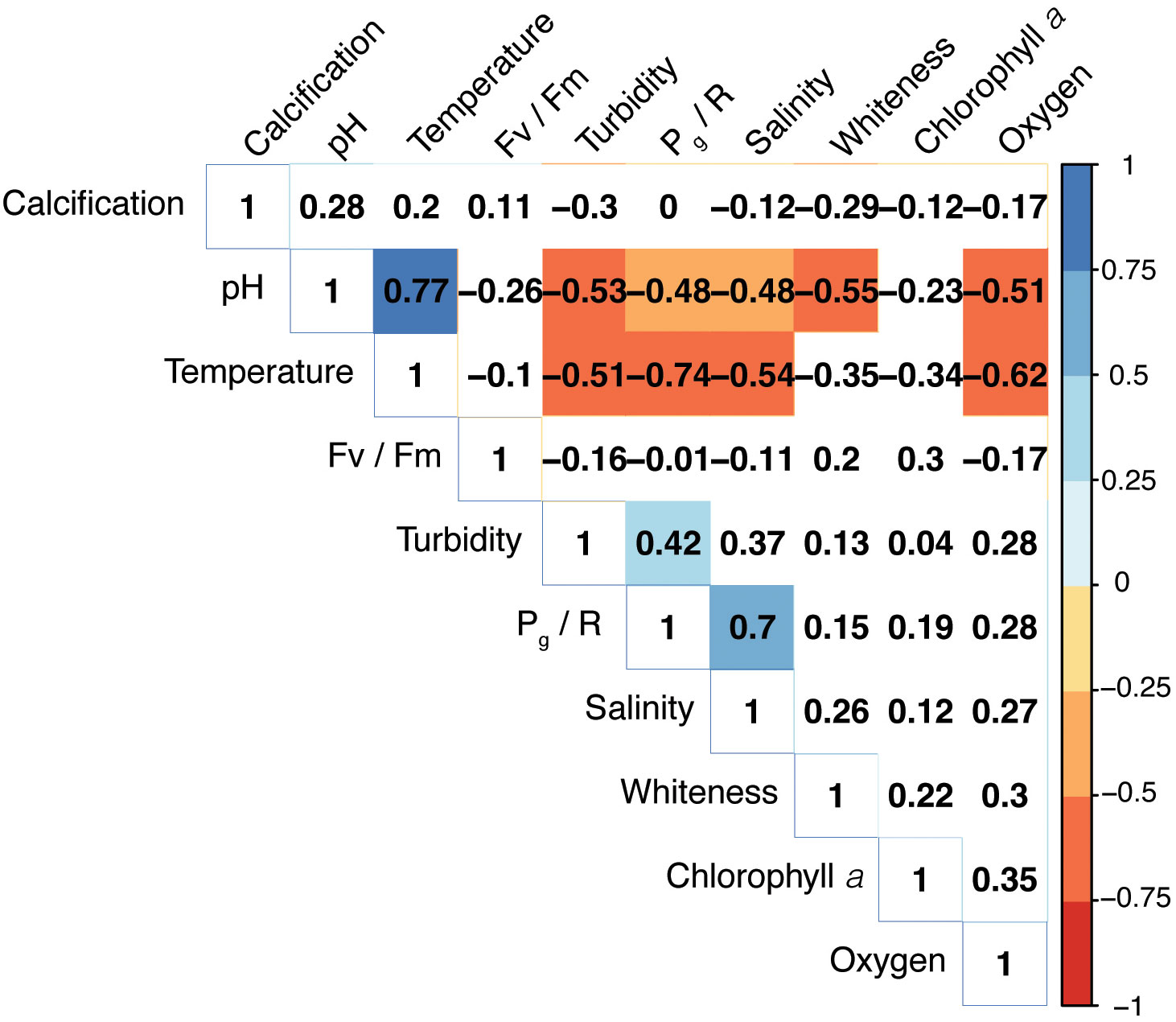
Figure 5 Correlogram of environmental variables and coral metabolic parameters based on Spearman’s correlation coefficients. Color intensity is proportional to the strength of the correlation. Only significant correlations (p< 0.05) are highlighted. The matrix was reordered using a hierarchical clustering.
Our two-year dataset of coral metabolic variables was best fitted using unimodal (Gaussian, lognormal or linear) models (Figure 6), as indicated by the AIC in Table 5, enabling an assessment of the distribution of each variable under different temperatures. Holobiont productivity (Pg/R) followed a lognormal distribution, with the highest values at 18-20°C (winter) and a decline at higher temperatures. The calcification rates followed a Gaussian distribution, reaching maximum rates at 26°C (spring). Photosynthetic efficiency (Fv/Fm) followed lognormal distribution, with maximum values at 21°C (winter). Finally, the coral whiteness levels followed a linear distribution, with higher values at lower temperatures (winter) and decreasing at higher temperatures.
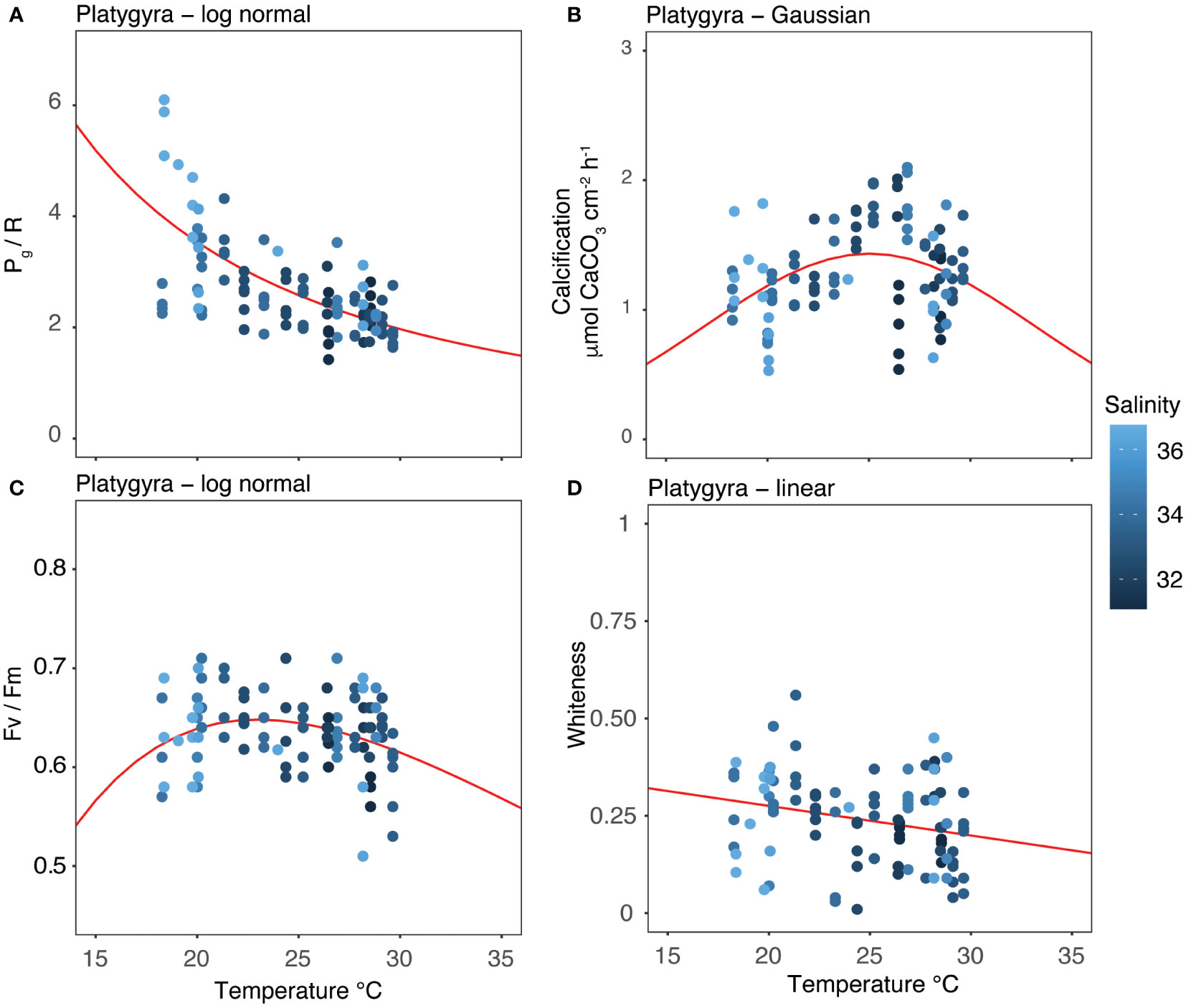
Figure 6 Thermal performance curves of each coral metabolic parameters: (A) Pg/R; (B) calcification; (C) Fv/Fm; and (D) whiteness. Points show descrete measurements collected every month between April 2018 and March 2020. Lines show the best-fitting models as determined by Akaike Information Criterion (AICc). The color gradient of the points represents the salinity level at the time of data collection.
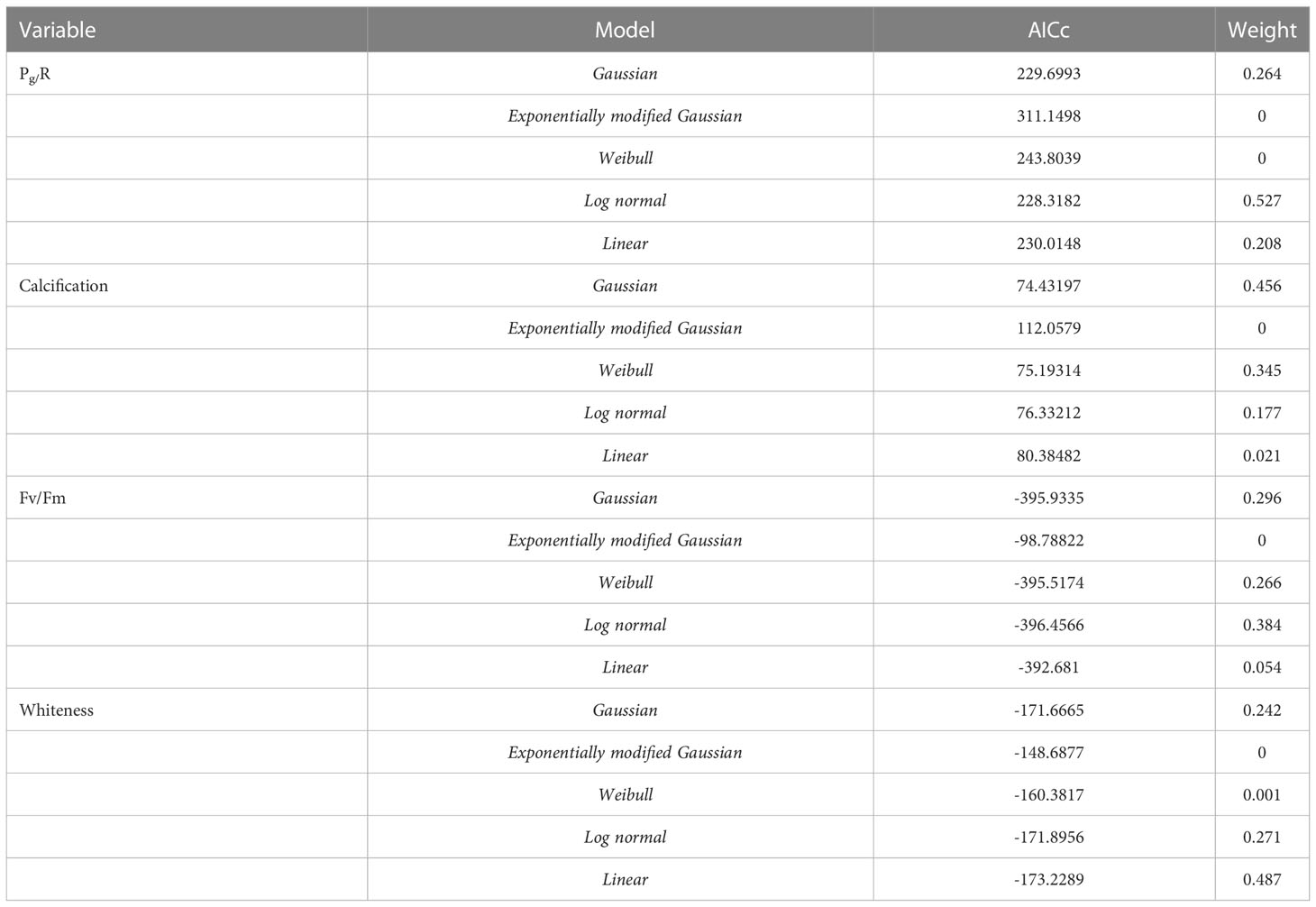
Table 5 Results of best-fit models for each metabolic parameter and temperature determined using the Akaike Information Criterion (AICc).
4. Discussion
The capacity of corals to modulate their physiology during thermal stress and environmental change is key to understanding which species are able to cope with current and future environmental conditions. Our examination of the stress-tolerant coral, P. carnosa, within a subtropical habitat, demonstrated its capacity to modulate energetic productivity (Pg/R) and key biological processes (biocalcification) in response to changing seasonal conditions. The physiological acclimation capabilities of subtropical corals under a particularly wide range of environmental conditions provide a unique and critical insight for understanding the response of corals to both global and local stressors.
Temperature was the main factor affecting the physiology of subtropical P. carnosa, with decreasing salinity likely a contributing factor. The metabolic response of P. carnosa was a pronounced reduction in its daily energetic productivity (Pg/R) during the summer (-44%) relative to winter (Figure 3A, Supplementary Table 2). A similar seasonal trend was also observed in the photosynthetic responses of acroporid corals between summer and winter by Vajed Samiei et al. (2015). The negative impacts of elevated temperature on coral metabolism are widely documented, especially in the case of tropical reefs where corals live nearer to their upper thermal limits year-round (McNeil et al., 2004; Oakley and Davy, 2018; Roth et al., 2021). Our results confirm that seasonal variations elicit a similar summertime response for subtropical corals, as observed in situ, adding new information on the modulation of energetics and threshold limits of corals. During winter, P. carnosa displayed higher productivity rates, with lognormal TPC curves indicating metabolic adaptability of this coral at low temperature and high salinity (Figure 6A), which contrasts with the productivity characteristics of other subtropical corals (McIlroy et al., 2019).
Temperature variations are also a key factor in the modulation of the internal carbonate system of corals and the biocalcification process. For example, although outcomes can be species-specific, elevated temperatures can reduce the concentration of dissolved inorganic carbon (DIC) in the calcifying fluids in non-visibly bleached corals (Schoepf et al., 2021) as well as net calcification rates under high-temperature and low-pH conditions combined (Guillermic et al., 2021). We observed a significant decrease in calcification rates during the transition from spring to summer (-20%), and from autumn to winter (-25%; Figure 3B). In contrast to adaptation mechanisms observed in the subtropics (Ross et al., 2018), these results suggest that P. carnosa living in subtropical areas may not have the ability to modulate the calcifying fluids to counter-balance seasonal changes in seawater carbonate chemistry, temperature, and light. The calcification maxima observed in spring (Figure 3B, 6B) were supported by the general increase in seawater temperature following the cold season (rising from 19.55 to 26.36°C, Table 1) combined with low chlorophyll-a and turbidity, and high solar intensities. High calcification rates have also been observed during the spring in the Red Sea, indicating that summertime temperature exceeded the optimum conditions for coral growth (Roik et al., 2015). In the subtropics, a negative correlation between high temperature and calcification rate is observed (Ross et al., 2018; Silbiger et al., 2019), and, as found in our study, coral growth is not maximized at warmer temperatures (i.e., summer). We recognize, however, that these patterns can be species- and location-specific responses driven by the local evolution in the subtropics (Cybulski et al., 2020). Based on our data, the increase in temperatures across the winter period was linked to a 2% increase in calcification rate for every 1°C of warming. Nevertheless, much more research is needed to estimate coral growth rates under future scenarios of ocean warming.
Interestingly, the photosynthetic efficiency (Fv/Fm) remained relatively stable during our study (Figure 3C, 6C), which is similar to reports of other coral species in the SCS (McIlroy et al., 2019). This indicates that the endosymbionts of P. carnosa, belonging to Cladocopium (formerly Symbiodinium Clade C; LaJeunesse et al., 2018), are capable of acclimation under natural conditions (Wong et al., 2016; Cai et al., 2018; Zhang et al., 2019). On the other hand, the whiteness level of the corals was higher during the winter (+0.1%, Figure 3D), when reduced temperatures, high salinity (Figure 6D), and low pH had a whitening effect on P. carnosa (ANOVA, F = 7.639, p< 0.01). This is related to a reduced density of endosymbionts in the coral tissue (Chow et al., 2016), which likely corresponds to the lower calcification rates during the winter relative to the spring (Supplementary Table 2). Importantly, this whitening effect remained within the threshold limits of P. carnosa, and no bleaching event was observed. In previous studies, P. carnosa whitening resulted from both low temperatures in the field (Chow et al., 2016), and low pH in the laboratory (Dellisanti et al., 2020a), although this coral species exhibits co-tolerance and long-life adaptations to multiple natural stressors (Yeung et al., 2021; Ip et al., 2022).
Regionally, we confirmed a strong environmental influence of the seasonal monsoon and typhoons (Ang et al., 2005; Figure 2), which characterize a unique marginal environment for coral adaptation and survival (Goodkin et al., 2011; Duprey et al., 2016). We were able to distinguish transitional seasonal conditions occurring in spring and autumn (Yin, 2003; Lee et al., 2006; Figure 4) with notably drastic changes during the winter-spring seasons. For example, in the eight-week period between March and May 2019, the seawater temperature in the study area increased by 5°C, salinity dropped by 1.9, and pH increased by 0.56 (Figure 2). Combined with high sunlight intensities, such changes can coincide with a proliferation of microalgae when combined with high sunlight intensity during spring (Supplementary Table 1). Indeed, algal blooms are well-studied in Hong Kong waters (Lu and Hodgkiss, 2004) and may negatively affect the metabolism of coral communities (Bauman et al., 2010; D’Angelo and Wiedenmann, 2014). Moreover, a combination of nutrient loading, rainfall, and temperature can stimulate localized eutrophication events (Duprey et al., 2020). Although no seasonal algal proliferation was observed during our study, major events occurred in July 2019 and March 2020, with chlorophyll-a concentrations reaching 19.5 and 13.5 μg/L, respectively (Table 1). This may have stimulated heterotrophy in the corals, which can mitigate the stress from other factors, as evidenced by the relatively low R rates and high Pg/R ratios during the winter (Figure 3A; Houlbrèque and Ferrier-Pagès, 2009; Courtney et al., 2017).
Within a subtropical reef environment, changing water conditions, such as low salinity and low pH, co-occur with elevated temperature, having interactive effects on the metabolisms of P. carnosa. Although the multivariate analysis showed that temperature was the main driver of the observed metabolic responses in P. carnosa, the Spearman’s correlation indicated that other factors, such as pH (r = - 0.48), salinity (r = 0.7), and turbidity (r = 0.42) also modulated coral productivity (Figure 5). Nevertheless, despite of large variations, the recorded extremes in temperature, salinity, and pH remained well within the tolerance limits of corals in the subtropical region of the SCS (Yuan et al., 2019; Dellisanti et al., 2020a). It is noteworthy, however, that low salinity, and hence At, can lead to lower rates of biocalcification (Dellisanti et al., 2020b). In summer, higher pigmentation (e.g., low whiteness) was observed, indicating the seasonal adaptability of photosynthetic symbionts in association with increases in endosymbiont density and chlorophyll concentrations (Chow et al., 2016).
Although our observations confirm the resilience of P. carnosa under fluctuating environmental conditions, we recognize that our measurements were limited to daytime hours, representing only a limited period (a few hours) of metabolic activity each day. Indeed, single monthly measurements of water parameters and coral metabolic rates are not inclusive of daily and weekly fluctuation in seawater and atmospheric conditions, especially during the summer, which is characterized by short-term monsoons or typhoons over periods of a few days. Therefore, longer-term and continuous measurements (over weeks or months) of seawater parameters-in particular temperature-are now needed to fully understand their effects on coral physiology in the SCS.
Overall, our findings demonstrate the metabolic changes that occur in stress-tolerant corals in response to seasonal changes in environmental conditions in a subtropical habitat. Energetic productivity and biocalcification were reduced at elevated temperatures, while P. carnosa demonstrated the capability to modulate its metabolism under variable salinity, pH, and turbidity conditions. Indeed, these corals are currently thriving under variable natural conditions, with no evidence of stress. This strongly suggests that P. carnosa exists in alternative states of development rather than in disturbed or restricted states (Perry and Larcombe, 2003).
Although our study highlights the resilience of P. carnosa physiology in response to seasonal changes in the SCS, other stressors must also be considered including land reclamation and increased sedimentation (Wong et al., 2018; Yeung et al., 2021). Importantly, corals are currently thriving in subtropical reefs subject to highly variable environmental conditions, which may help partially mitigate broader-scale declines in corals associated with global climate change. As such, the seasonal drivers of coral productivity and biocalcification in the subtropical coral species reported here highlight the importance of identifying the full range of factors affecting coral health at both local and global scales.
Data availability statement
The datasets presented in this study can be found in online repositories. The names of the repository/repositories and accession number(s) can be found below: Data are available online in the Pangaea repository system https://doi.pangaea.de/10.1594/PANGAEA.937210 and https://doi.pangaea.de/10.1594/PANGAEA.937214.
Author contributions
WD, JW and LC conceived the ideas and designed methodology; WD, JC, SY, and RT collected the data; WD and RT analysed the data; WD led the writing of the manuscript. All authors contributed to the article and approved the submitted version.
Funding
This study was supported by the Southern Marine Science and Engineering Guangdong Laboratory (Guangzhou) (SMSEGL20SC02); the Collaborative Research Fund (C7013-19G) of the Hong Kong Research Grants Council; the National Natural Science Foundation of China (41641047) and the SKLMP Seed Collaborative Research Fund (SCRF/0027).
Acknowledgments
The authors thank all the collaborators, SCUBA divers, boat captains involved in this project, and the constructive comments received by reviewers.
Conflict of interest
The authors declare that the research was conducted in the absence of any commercial or financial relationships that could be construed as a potential conflict of interest.
Publisher’s note
All claims expressed in this article are solely those of the authors and do not necessarily represent those of their affiliated organizations, or those of the publisher, the editors and the reviewers. Any product that may be evaluated in this article, or claim that may be made by its manufacturer, is not guaranteed or endorsed by the publisher.
Supplementary material
The Supplementary Material for this article can be found online at: https://www.frontiersin.org/articles/10.3389/fmars.2023.994591/full#supplementary-material
References
Anderson M. J., Gorley R. N., Clarke K. R. (2008). PERMANOVA+ for primer: Guide to software and statistical methods (Plymouth: PRIMER-E, Ltd).
Ang P. O. Jr, Choi L. S., Choi M. M., Cornish A., Fung H. L., Lee M. W., et al. (2005). Hong Kong Japan Wildlife research center, ministry of the environment, government of Japan. Status Coral Reefs East Asian Seas Region: 2004 (Tokyo), 121–152.
Angilletta M. J. (2006). Estimating and comparing thermal performance curves. J. Therm. Biol. 31, 541–545. doi: 10.1016/j.jtherbio.2006.06.002
Anthony K. R. N. (2016). Coral reefs under climate change and ocean acidification: Challenges and opportunities for management and policy. Annu. Rev. Environ. Resour. 41, 59–81. doi: 10.1146/annurev-environ-110615-085610
Bauman A. G., Burt J. A., Feary D. A., Marquis E., Usseglio P. (2010). Tropical harmful algal blooms: An emerging threat to coral reef communities? Mar. Poll. Bull. 60, 11. doi: 10.1016/j.marpolbul.2010.08.015
Beger M., Sommer B., Harrison P. L., Smith S. D. A., Pandolfi J. M. (2014). Conserving potential coral reef refuges at high latitudes. Divers. Distrib. 20, 245–257. doi: 10.1111/ddi.12140
Biscéré T., Zampighi M., Lorrain A., Jurriaans S., Foggo A., Houlbrèque F., et al. (2019). High pCO2 promotes coral primary production. Biol. Lett. 15, 20180777. doi: 10.1098/rsbl.2018.0777
Brown B. E., Dunne R. P., Goodson M. S., Douglas A. E. (2000). Bleaching patterns in reef corals. Nature 404, 142–143. doi: 10.1038/35004657
Browne N., Precht E., Last K., Todd P. (2014). Photo-physiological costs associated with acute sediment stress events in three near-shore turbid water corals. Mar. Ecol. Prog. Ser. 502, 129–143. doi: 10.3354/meps10714
Browne N. K., Tay J. K. L., Low J., Larson O., Todd P. A. (2015). Fluctuations in coral health of four common inshore reef corals in response to seasonal and anthropogenic changes in water quality. Mar. Environ. Res. 105, 39–52. doi: 10.1016/j.marenvres.2015.02.002
Cai L., Zhou G., Tong H., Tian R.-M., Zhang W., Ding W., et al. (2018). Season structures prokaryotic partners but not algal symbionts in subtropical hard corals. Appl. Microbiol. Biotechnol. 102, 4963–4973. doi: 10.1007/S00253-018-8909-5
Camp E. F., Schoepf V., Mumby P. J., Hardtke L. A., Rodolfo-Metalpa R., Smith D. J., et al. (2018). The future of coral reefs subject to rapid climate change: Lessons from natural extreme environments. Front. Mar. Sci. 5. doi: 10.3389/fmars.2018.00004
Castillo K. D., Ries J. B., Bruno J. F., Westfield I. T. (2014). The reef-building coral Sidastrea siderea exhibits parabolic responses to ocean acidification and warming. Proc. R. Soc B. 281, 20141856. doi: 10.1098/rspb.2014.185
Chow M. H., Tsang R. H. L., Lam E. K. Y., Ang P. (2016). Quantifying the degree of coral bleaching using digital photographic technique. J. Exp. Mar. Bio. Ecol. 479, 60–68. doi: 10.1016/j.jembe.2016.03.003
Chui A. P. Y., Yeung C. W., Tsang R. H. L., Leung Y. H., Ng T. Y., Chai K. H., et al. (2016). Lowered temperature and reduced salinity retarded development of early life history stages of Acropora valida from the marginal environment. Reg. Stud. Mar. Sci. 8, 430–438. doi: 10.1016/j.rsma.2016.04.004
Comeau S., Cornwall C. E., Pupier C. A., DeCarlo T. M., Alessi C., Trehern R., et al. (2019). Flow-driven micro-scale pH variability affects the physiology of corals and coralline algae under ocean acidification. Sci. Rep. 9, 12829. doi: 10.1038/s41598-019-49044-w
Costanza R., de Groot R., Sutton P., van der Ploeg S., Anderson S. J., Kubiszewski I., et al. (2014). Changes in the global value of ecosystem services. Glob. Environ. Change 26, 152–158. doi: 10.1016/j.gloenvcha.2014.04.002
Courtney T. A., Lebrato M., Bates N. R., Collins A., De Putron S. J., Garley R., et al. (2017). Environmental controls on modern scleractinian coral and reef-scale calcification. Sci. Adv. 3 (11), e1701356. doi: 10.1126/sciadv.1701356
Cybulski J. D., Husa S. M., Duprey N. N., Mamo B. L., Tsang T. P. N., Yasuhara M., et al. (2020). Coral reef diversity losses in china’s greater bay area were driven by regional stressors. Sci. Adv. 6, eabb1046. doi: 10.1126/sciadv.abb1046
D’Angelo C., Wiedenmann J. (2014). Impacts of nutrient enrichment on coral reefs: New perspectives and implications for coastal management and reef survival. Curr. Opin. Environ. Sustain. 7, 82–93. doi: 10.1016/j.cosust.2013.11.029
Dellisanti W., Tsang R. H. L., Ang P., Wu J., Wells M. L., Chan L. L. (2020a). Metabolic performance and thermal and salinity tolerance of the coral platygyra carnosa in Hong Kong waters. Mar. pollut. Bull. 153, 111005. doi: 10.1016/j.marpolbul.2020.111005
Dellisanti W., Tsang R. H. L., Ang P., Wu J., Wells M. L., Chan L. L. (2020b). A diver-portable respirometry system for in-situ short-term measurements of coral metabolic health and rates of calcification. Front. Mar. Sci. 7. doi: 10.3389/fmars.2020.571451
Dias M., Madeira C., Jogee N., Ferreira A., Gouveia R., Cabral H., et al. (2019). Oxidative stress on scleractinian coral fragments following exposure to high temperature and low salinity. Ecol. Indic. 107, 105586. doi: 10.1016/j.ecolind.2019.105586
Dickson A. G., Sabine C. L., Christian J. R. (2007). Guide to best practices for ocean CO2 measurement (Sidney: North Pacific Marine Science Organization).
Dove S. G., Kline D. I., Pantos O., Angly F. E., Tyson G. W., Hoegh-Guldberg O. (2013). Future reef decalcification under a business-as-usual CO2 emission scenario. Proc. Natl. Acad. Sci. U. S. A. 110, 15342–15347. doi: 10.1073/pnas.1302701110
Duprey N. N., Wang T. X., Kim T., Cybulski J. D., Vonhof H. B., Crutzen P. J., et al. (2020). Megacity development and the demise of coastal coral communities: evidence from coral skeleton δ15N records in the pearl river eastuary. Glob. Change Biol. 263, 1338–1353. doi: 10.1111/gcb.14923
Duprey N. N., Yasuhara M., Baker D. M. (2016). Reefs of tomorrow: eutrophication reduces coral biodiversity in an urbanized seascape. Glob. Change Biol. 22, 3550–3565. doi: 10.1111/gcb.13432
Genevier L. G. C., Jamil T., Raitsos D. E., Krokos G., Hoteit I. (2019). Marine heatwaves reveal coral reef zones susceptible to bleaching in the red Sea. Glob. Change Biol. 25, gcb.14652. doi: 10.1111/gcb.14652
Goodkin N. F., Switzer A. D., McCorry D., Devantier L., True J. D., Hughen K., et al. (2011). Coral communities of Hong Kong: long-lived corals in a marginal reef environment. Mar. Ecol. Prog. Ser. 426, 185–196.
Guillermic M., Cameron L. P., De Corte I., Misra S., Bijma J., De Beer D., et al. (2021). Thermal stress reduces pocilloporid coral resilience to ocean acidification by impairing control over calcifying fluid chemistry. Sci. Adv. 7, eaba9958. doi: 10.1126/sciadv.aba9958
Heery E. C., Hoeksema B. W., Browne N. K., Reimer J. D., Ang P. O., Huang D., et al. (2018). Urban coral reefs: Degradation and resilience of hard coral assemblages in coastal cities of East and southeast Asia. Mar. pollut. Bull. 135, 654–681. doi: 10.1016/j.marpolbul.2018.07.041
Hobday A. J., Alexander L. V., Perkins S. E., Smale D. A., Straub S. C., Oliver E. C. J., et al. (2016). A hierarchical approach to defining marine heatwaves. Prog. Oceanogr. 141, 227–238. doi: 10.1016/j.pocean.2015.12.014
Hoegh-Guldberg O., Mumby P. J., Hooten A. J., Steneck R. S., Greenfield P., Gomez E., et al. (2007). Coral reefs under rapid climate change and ocean acidification. Science 318, 1737–1742. doi: 10.1126/science.1152509
Hoegh-Guldberg O., Poloczanska E. S., Skirving W., Dove S. (2017). Coral reef ecosystems under climate change and ocean acidification. Fron. Mar. Sci. 4. doi: 10.3389/fmars.2017.00158
Houlbrèque F., Ferrier-Pagès C. (2009). Heterotrophy in tropical scleractinian corals. Biol. Rev. 84 (1), 1–17. doi: 10.1111/j.1469-185X.2008.00058.x
Ip J. C.-H., Zhang Y., Xie J. Y., Yeung Y. H., Qiu J.-W. (2022). Comparative transcriptomics of two coral holobionts collected during the 2017 El niño heat wave reveal differential stress response mechanisms. Mar. Poll. Bull. 182, 114017. doi: 10.1016/j.marpolbul.2022.114017
Jones R., Giofre N., Luter H. M., Neoh T. L., Fisher R., Duckworth A. (2020). Responses of corals to chronic turbidity. Sci. Rep. 10, 1–13. doi: 10.1038/s41598-020-61712-w
Keppel G., Van Niel K. P., Wardell-Johnson G. W., Yates C. J., Byrne M., Mucina L., et al. (2012). Refugia: Identifying and understanding safe havens for biodiversity under climate change. Glob. Ecol. Biogeogr. 21, 393–404. doi: 10.1111/j.1466-8238.2011.00686.x
Kleypas J. A., McManus J. W., Menez L. A. A. (1999). Environmental limits to coral reef development: Where do we draw the line? Am. Zool. 39, 146–159. doi: 10.1093/icb/39.1.146
Kroeker K. J., Kordas R. L., Crim R. N., Singh G. G. (2010). Meta-analysis reveals negative yet variable effects of ocean acidification on marine organisms. Ecol. Lett. 13, 1419–1434. doi: 10.1111/j.1461-0248.2010.01518.x
LaJeunesse T. C., Parkinson J. E., Gabrielson P. W., Jeong H. J., Reimer J. D., Voolstra C. R., et al. (2018). Systematic revision of symbiodiniaceae highlights the antiquity and diversity of coral rndosymbionts. Curr. Biol. 28, 2570.e6–2580.e6. doi: 10.1016/j.cub.2018.07.008
Lee I. H., Fan T. Y., Fu K. H., Ko D. S. (2020). Temporal variation in daily temperature minima in coral reefs of nanwan bay, southern Taiwan. Sci. Rep. 10, 1–10. doi: 10.1038/s41598-020-65194-8
Lee J. H. W., Harrison P. J., Kuang C., Yin K. (2006). “Eutrophication dynamics in Hong Kong coastal waters: physical and biological interactions,” in The environment in Asia pacific harbours. Ed. Wolanski E. (Dordrecht: Springer). doi: 10.1007/1-4020-3655-8_13
Loiola M., Cruz I. C. S., Lisboa D. S., Mariano-Neto E., Leão Z. M. A. N., Oliveira M. D. M., et al. (2019). Structure of marginal coral reef assemblages under different turbidity regime. Mar. Environ. Res. 147, 138–148. doi: 10.1016/j.marenvres.2019.03.013
Lu S., Hodgkiss I. J. (2004). Harmful algal bloom causative collected from Hong Kong waters. Hydrobiologia 512:1-3 pp, 231–238.
McIlroy S. E., Thompson P. D., Yuan F. L., Bonebrake T. C., Baker D. M. (2019). Subtropical thermal variation supports persistence of corals but limits productivity of coral reefs. Proc. R. Soc B Biol. Sci. 286, 20190882. doi: 10.1098/rspb.2019.0882
McNeil B. I., Matear R. J., Barnes D. J. (2004). Coral reef calcification and climate change: the effect of ocean warming. Geophys. Res. Lett. 31, 1–4. doi: 10.1029/2004GL021541
Ng C. S. L., Huang D., Toh K., Ben, Sam S. Q., Kikuzawa Y. P., et al. (2020). Responses of urban reef corals during the 2016 mass bleaching event. Mar. pollut. Bull. 154, 111111. doi: 10.1016/j.marpolbul.2020.111111
Noonan S. H. C., Kluibenschedl A., Fabricius K. E. (2018). Ocean acidification alters early successional coral reef communities and their rates of community metabolism. PloS One 13, e0197130. doi: 10.1371/journal.pone.0197130
Oakley C. A., Davy S. K. (2018). “Cell biology of coral bleaching,” in Coral bleaching. ecological studies, vol. 233 . Eds. van Oppen M., Lough J. (Cham: Springer). doi: 10.1007/978-3-319-75393-5_8
Pitts K. A., Campbell J. E., Figueiredo J., Fogarty N. D. (2020). Ocean acidification partially mitigates the negative effects of warming on the recruitment of the coral, Orbicella faveolata. Coral Reefs 39, 281–292. doi: 10.1007/s00338-019-01888-4
Ralph P., Gademann R., Larkum A., Schreiber U. (1999). In situ underwater measurements of photosynthetic activity of coral zooxanthellae and other reef-dwelling dinoflagellate endosymbionts. Mar. Ecol. Prog. Ser. 180, 139–147. doi: 10.3354/meps180139
Roberty S., Béraud E., Grover R., Ferrier-Pagès C. (2020). Coral productivity is co-limited by bicarbonate and ammonium availability. Microorganisms 8, 640. doi: 10.3390/microorganisms8050640
Roik A., Roder C., Röthig T., Voolstra C. R. (2016). Spatial and seasonal reef calcification in corals and calcareous crusts in the central red Sea. Coral Reefs 35, 681–693. doi: 10.1007/s00338-015-1383-y
Ross C. L., Schoepf V., DeCarlo T. M., McCulloch M. T. (2018). Mechanisms and seasonal drivers of calcification in the temperate coral Turbinaria reniformis at its latitudinal limits. Proc. R. Soc B: Biol. Sci. 285 (1879), 20180215. doi: 10.1098/rspb.2018.0215
Roth F., RAdecker N., Carvalho S., Duarte C. M., Saderne V., Anton A., et al. (2021). High summer temperatures amplify functional differences between coral- and algae-dominated reef communities. Ecology 102, e03226. doi: 10.1002/ecy.3226
Schoepf V., D’Olivo J. P., Rigal C., Jung E. M. U., McCulloch M. T. (2021). Heat stress differentially impacts key calcification mechanisms in reef-building corals. Coral Reefs 40, 459–471. doi: 10.1007/s00338-020-02038-x
Silbiger N. J., Goodbody-Gringley G., Bruno J. F., Putnam H. M. (2019). Comparative thermal performance of the reef-building coral Orbicella franksi at its latitudinal range limits. Mar. Biol. 166, 126. doi: 10.1007/s00227-019-3573-6
Soriano-Santiago O. S., Liñán-Cabello M. A., Delgadillo-Nuño M. A., Ortega-Ortiz C., Cuevas-Venegas S. (2013). Physiological responses to oxidative stress associated with pH variations in host tissue and zooxanthellae of hermatypic coral Pocillopora capitata. Mar. Freshw. Behav. Physiol. 46, 275–286. doi: 10.1080/10236244.2013.827877
Trisos C. H., Merow C., Pigot A. L. (2020). The projected timing of abrupt ecological disruption from climate change. Nature 580, 496–501. doi: 10.1038/s41586-020-2189-9
Vajed Samiei J., Saleh A., Mehdinia A., Shirvani A., Kayal M. (2015). Photosynthetic response of Persian gulf acroporid corals to summer versus winter temperature deviations. PeerJ 3, e1062. doi: 10.7717/peerj.1062
Veron J. E. N. (2000). Corals of the world. vols. 1-3 AIMS and CRR, Queensland, Australia rev. Biol. Trop. 49:3-4, 1289–1290.
Wong K. T., Chui A. P. Y., Lam E. K. Y., Ang P. (2018). A 30-year monitoring of changes in coral community structure following anthropogenic disturbances in tolo harbour and channel, Hong Kong. Mar. pollut. Bull. 133, 900–910. doi: 10.1016/j.marpolbul.2018.06.049
Wong J. C. Y., Thompson P., Xie J. Y., Qiu J. W., Baker D. M. (2016). Symbiodinium clade c generality among common scleractinian corals in subtropical Hong Kong. Reg. Stud. Mar. Sci. 8, 439–444. doi: 10.1016/J.RSMA.2016.02.005
Wooldridge S. A., Done T. J. (2009). Improved water quality can ameliorate effects of climate change on corals. Ecol. Appl. 19, 1492–1499. doi: 10.1890/08-0963.1
Wyatt A. S. J., Leichter J. J., Toth L. T., Miyajima T., Aronson R. B., Nagata T. (2020). Heat accumulation on coral reefs mitigated by internal waves. Nat. Geosci. 13, 28–34. doi: 10.1038/s41561-019-0486-4
Yeung Y. H., Xie J. Y., Kwok C. K., Kei K., Ang P., Chan L. L., et al. (2021). Hong Kong’s subtropical scleractinian coral communities: Baseline, environmental drivers and management implications. J. W vMar. pollut. Bull. 167, 112289. doi: 10.1016/j.marpolbul.2021.112289
Yin K. D. (2003). Influence of monsoons and oceanographic processes on red tides in Hong Kong waters. Mar. Ecol. Prog. Ser. 262, 27–41. doi: 10.3354/meps262027
Yuan X., Guo Y., Cai W.j., Huang H., Zhou W., Liu S. (2019). Coral responses to ocean warming and acidification: Implications for future distribution of coral reefs in the south China Sea. Mar. pollut. Bull. 138, 241–248. doi: 10.1016/j.marpolbul.2018.11.053
Keywords: coral physiology, metabolic performance, metabolic rates, phenotypic plasticity, subtropical corals
Citation: Dellisanti W, Chung JTH, Yiu SKF, Tsang RHL, Ang P Jr., Yeung YH, Qiu J-W, McIlroy SE, Wells ML, Wu J and Chan LL (2023) Seasonal drivers of productivity and calcification in the coral Platygyra carnosa in a subtropical reef. Front. Mar. Sci. 10:994591. doi: 10.3389/fmars.2023.994591
Received: 15 July 2022; Accepted: 11 January 2023;
Published: 30 January 2023.
Edited by:
Verena Schoepf, University of Amsterdam, NetherlandsReviewed by:
Jessica Reichert, University of Giessen, GermanyStephen Levas, University of Wisconsin–Whitewater, United States
Copyright © 2023 Dellisanti, Chung, Yiu, Tsang, Ang, Yeung, Qiu, McIlroy, Wells, Wu and Chan. This is an open-access article distributed under the terms of the Creative Commons Attribution License (CC BY). The use, distribution or reproduction in other forums is permitted, provided the original author(s) and the copyright owner(s) are credited and that the original publication in this journal is cited, in accordance with accepted academic practice. No use, distribution or reproduction is permitted which does not comply with these terms.
*Correspondence: Leo Lei Chan, leochan@cityu.edu.hk