Abstract
Owing to its thermoresponsive and photocrosslinking characteristics, gelatin methacryloyl (GelMA)-based biomaterials have gained widespread usage as a novel and promising bioink for three-dimensional bioprinting and diverse biomedical applications. However, the flow behaviors of GelMA during the sol-gel transition, which are dependent on time and temperature, present significant challenges in printing thick scaffolds while maintaining high printability and cell viability. Moreover, the tunable properties and photocrosslinking capabilities of GelMA underscore its potential for localized drug delivery applications. Previous research has demonstrated the successful incorporation of minocycline (MH) into GelMA scaffolds for therapeutic applications. However, achieving a prolonged and sustained release of concentrated MH remains a challenge, primarily due to its small molecular size. The primary aim of this study is to investigate an optimal extrusion printing method for GelMA bioink in extrusion bioprinting, emphasizing its flow behaviors that are influenced by time and temperature. Additionally, this research seeks to explore the potential of GelMA bioink as a carrier for the sustained release of MH, specifically targeting cellular protection against oxidative stress. The material properties of GelMA were assessed and further optimization of the printing process was conducted considering both printability and cell survival. To achieve sustained drug release within GelMA, the study employed a mechanism using metal ion mediation to facilitate the interaction between MH, dextran sulfate (DS), and magnesium, leading to the formation of nanoparticle complexes (MH-DS). Furthermore, a GelMA-based in vitro model was developed in order to investigate the cellular protective properties of MH against oxidative stress. The experimental results revealed that the printability and cell viability of GelMA are significantly influenced by the printing duration, nozzle temperature, and GelMA concentrations. Optimal printing conditions were identified based on a thorough assessment of both printability and cell viability. Scaffolds printed under these optimal conditions exhibited exceptional printability and sustained high cell viability. Notably, it was found that lower GelMA concentrations reduced the initial burst release of MH from the MH-dextran sulfate (MH-DS) complexes, thus favoring more controlled, sustained release profiles. Additionally, MH released under these conditions significantly enhanced fibroblast viability in an in vitro model simulating oxidative stress.
Export citation and abstract BibTeX RIS

Original content from this work may be used under the terms of the Creative Commons Attribution 4.0 license. Any further distribution of this work must maintain attribution to the author(s) and the title of the work, journal citation and DOI.
1. Introduction
Reactive oxygen species (ROS) are chemically reactive molecules that contain oxygen free radicals. These compounds are naturally produced during normal cellular metabolism activities [1]. Nevertheless, under conditions of environmental stress, the levels of ROS may undergo a substantial rise, inflicting significant damage on cellular structures [2, 3]. Minocycline (MH), a semi-synthetic derivative of tetracycline, is renowned for its efficacy against both gram-positive and gram-negative bacteria by impeding bacterial protein biosynthesis [4]. Beyond its antimicrobial prowess, MH exhibits antioxidative, anti-inflammatory, and anti-apoptotic capabilities, making it a versatile agent in combating infections, inflammation, and neurodegenerative diseases [5]. MH has been shown to effectively reduce oxidative stress through multiple mechanisms. These include inhibiting the production of ROS from microglia, impeding the activation of nicotinamide adenine dinucleotide phosphate (NADPH) oxidase triggered by thrombin and Zinc, and serving as a direct scavenger of ROS [6]. Systemic delivery of MH carries risks such as hypersensitivity reactions, gastrointestinal intolerance, and antibiotic resistance. Its role in managing periodontal diseases is controversial, primarily due to the necessitation of high dosages, which inadvertently escalates the risk of increased morbidity and hepatotoxicity in animal models [7]. Therefore, it is crucial to consider alternative local delivery methods and treatment strategies. Hydrogels have been widely used to encapsulate drugs or drug-loading carriers for sustained local delivery owing to is high water content and tunable material properties. To achieve curative potential of drug, sustained release of high concentration for an extended time period is often needed [8]. Hydrogels often serve as scaffolding materials to provide support for tissue regeneration and healing. Additionally, for topical wound dressings or drug-loaded implants, customization of shape and size may be necessary to accommodate the lesion geometries and unique needs of each patient.
Three-dimensional (3D) bioprinting is an emerging interdisciplinary technology aimed at building 3D biomimetic and functional tissue constructs. In a typical bioprinting process, 'bioinks', comprising living cells, biomaterials, and bioactive factors (e.g. growth factors), are systematically deposited in a layer-by-layer manner to form 3D constructs with precise spatial control [9–11]. Distinct from traditional tissue engineering methodologies, bioprinting possesses the unique capability to reproduce the intricate microenvironments of native tissues or organs. This advanced approach facilitates the creation of heterogeneous, biomimetic tissue constructs with customized shape and internal structure (e.g. porosity) [12]. 3D bioprinting has been widely used in a variety of applications such as regeneration and transplantation of tissues and organs [13–15], high throughput drug screening and testing [16, 17], and in vitro disease modeling [18–20]. Over the past two decades, bioprinting has undergone a remarkable evolution, transitioning from the printing of biocompatible materials to the printing of living cells and organoid-based bioinks for tissue regeneration and personalized medicine. The need for structurally complex, high-resolution, and biocompatible bioprinted constructs has catalyzed intensive research into innovative bioink formulations and bioprinting techniques [10].
Among various types of bioinks, GelMA bioinks stand out due to their exceptional cytocompatibility and highly tunable physical properties. Gelatin methacryloyl (GelMA) possesses favorable biochemical characteristics derived from gelatin and undergoes fast covalent crosslinking upon light irradiation, allowing exceptional temporal and spatial control. Gelatin methacryloyl (GelMA) exhibits biodegradability, biocompatibility, and cell attachment-promoting properties due to its origin from collagen, an extracellular matrix (ECM) component. It naturally contains metalloprotease (MMP) digestion sites and arginine-glycine-aspartic acid (RGD) sequences [21]. However, directly bioprinting of cell-laden GelMA bioinks is still challenging due to its relatively low printability. GelMA has low viscosity and processibility at physiological temperature and this often results in low print resolution and inconsistent printing quality. Furthermore, GelMA's flow behavior is dependent on both temperature and time, necessitating thorough material characterization, temperature control, along with the holding and printing times throughout the printing process, to ensure the production of filaments with uniform quality. Numerous studies have explored using GelMA in extrusion bioprinting. Liu et al pioneered an approach in which they effectively bioprinted cell-laden GelMA structures. Their method involved an initial cooling phase for the bioink precursor, inducing the formation of physical gels prior to the printing process [22]. Demonstrating remarkable characteristics, the bioinks used exhibited self-healing and shear-thinning properties, which are crucial for maintaining the shape and structural integrity of the constructs post-printing. The team achieved successful bioprinting with a concentration as low as 3% GelMA, without compromising cell viability and function, underscoring the material's potential for efficiency and effectiveness in tissue engineering applications. However, bioprinting GelMA has limitations in size and shape due to its time and temperature dependent rheological property. Printing larger scaffolds requires longer time and brings inconsistency to bioink properties [23]. In the pursuit of enhanced performance, researchers have formulated various GelMA composite bioink by blending GelMA with nanomaterials [24–27], other natural based polymers such as alginate [28], and hyaluronic acid [29]. Nonetheless, introducing other components into GelMA adds complexity to crosslinking strategies and bioprinting procedures. Additionally, the printability window of GelMA has not been exhaustively investigated. Previous studies have reported the printability and cell viability characterization for GelMA printing. For example, Yin et al developed the printability window for the optimal composition ratio of GelMA/gelatin based bioink while at fixed temperature condition [30]. Liu et al presented a strategy to cool the low concentration GelMA bioink at 4 °C and then print at 21 °C. Although the study investigated time-dependent rheology at 21 °C following a 5min cooling period at 4 °C, it did not consider these factors in relation to extending the printable time during the printing process. Therefore, only low aspect ratio and thin scaffolds can be printed using their strategy [22]. There have also been studies evaluating the cell damage during extrusion printing process [31–33] but rarely any studies incorporated both printability and cell viability to yield a merged process map, thus constraining its potential for wider biomedical and bioprinting applications.
In this study, our primary objective was to establish a standardized protocol for GelMA bioprinting, considering its rheological properties that are time and temperature-sensitive. Following the establishment of this protocol, we proceeded to explore the capabilities of GelMA-based wound dressing scaffolds that are incorporated with MH-loaded nanoparticles. These scaffolds are specifically engineered to ensure sustained release of drugs and protection of cells in the presence of oxidative stress. Specifically, we first performed an extensive evaluation of GelMA as a standalone bioink component for extrusion-based bioprinting, characterizing its chemical structure, degree of substitution, swelling property, degradation profiles, mechanical properties, and rheological properties. We analyzed GelMA's printability by studying the effects of concentration, holding temperature, and time. Furthermore, we assessed the impact of holding time and temperature on cell viability. Based on these factors, we defined optimized printing zones that balance printability and cell viability. Subsequently, we engineered nanoparticle complexes (MH-DS) to encapsulate and preserve MH using a metal ion mediated interaction mechanism involving MH, dextran sulfate (DS), and Mg2+ ions, as previously reported [8]. These nanoparticles were then integrated into GelMA for controlled release. Building on groundwork on optimized cell printing and sustained release, an in vitro oxidative stress model with fibroblasts was employed to evaluate the anti-oxidative and anti-apoptotic properties of the released MH.
2. Materials and methods
2.1. Materials
Gelatin (type A from porcine skin), methacrylic anhydride (MAA), collagenase type II, sodium carbonate, sodium hydroxide, hydrogen chloride, sodium bicarbonate, sodium dodecyl sulfate, MH, DS, magnesium chloride hexahydrate, Paraformaldehyde (PFA), Fluoroshield™ with DAPI, 2,4,6-trinitrobenzene sulfonic acid (TNBS) were purchased from Sigma-Aldrich (St. Louis, USA). LIVE/DEAD cell viability kit, alamarBlue cell viability assay, Dulbecco's phosphate buffered saline (DPBS), AlexaFluor™ 488 phalloidin were purchased from ThermoFisher (Waltham, MA, USA). Lithium phenyl-2,4,6-trimethylbenzoylphosphinate (LAP) was purchased from SunP Biotech LLC (Cherry Hill, NJ, USA).
2.2. Cell culture
All cells were cultured in a humidified incubator maintained at 37 °C and 5% CO2. L929 fibroblasts were grown in Dulbecco's Modified Eagle Medium (DMEM), supplemented with 10% (v/v) Fetal Bovine Serum (FBS, ATCC) and 1% (v/v) penicillin-streptomycin (PS, Thermo Fisher). The fibroblasts were kept in tissue culture flasks (Corning) for expansion. The culture medium was changed twice a week, and cells were sub-cultured upon reaching 80% confluency.
2.3. GelMA synthesis and hydrogel/bioink preparation
GelMA was synthesized based on a protocol previously reported [34]. Briefly, gelatin type A was dissolved into CB buffer (0.25 M) at 10% (w/v) under 50 °C. pH was adjusted to 9 by adding 5 M NaOH. Then MAA at the feeding ratio of 0.1 ml g−1 (MAA/gelatin) was added to gelatin solution in dropwise followed by 1 h reaction at 50 °C Then reaction was terminated by adjusting pH to 7.4 using 6 M HCl. The solution was transferred to dialysis tube (12–14 kDa) and dialysis against distilled water for 7 d and then lyophilize for 7 d. GelMA is collected and stored in the dark at 4 °C. Methacrylation was verified by 1H NMR and degree of substitution was determined by TNBS assay (figure S1). 5, 10 and 20% (w/v) acellular GelMA hydrogel were prepared by adding photoinitiator (LAP) to the DPBS solution at 60 °C to achieve a final concentration of 0.5% (w/v) with rigorous stirring until fully dissolved. Then freeze dried GelMA macromer was added into solution and maintained at 40 °C for 1 h to get homogeneous solution. Cell-laden GelMA bioinks were prepared by replacing the DPBS with conditioned DMEM. Then the solution was filtered through 0.22 µm PES membrane. Finally, cells were added to the solution to achieve a certain density. In this paper, GelMA hydrogel refers to acellular bioink while GelMA bioink refers to cell-laden bioink. All GelMA concentration percentages that appeared in this study refer to weight/volume (w/v).
2.4. Time and temperature dependent flow behaviors of GelMA hydrogel
Rheological properties of 5, 10, 20% (w/v) GelMA hydrogel were tested using R/S-CPS+ rheometer (Brookfield, MA) equipped with temperature control unit. Parallel plate geometry with 1 mm gap was used for all measurement. Firstly, 2 ml GelMA hydrogel precursor samples were incubated in 37 °C and then placed on the platform to fill the gap. The dynamic viscosities were recorded in temperature sweep mode from 27 °C to 10 °C at fixed shear rate (1 1 s−1). Then the shear thinning properties were investigated at the 21 °C for all GelMA samples with shear rate ramping from 0 to 100 1 s−1. Time dependent flow behaviors and dynamic viscosities of GelMA were recorded and for 5%, 10%, and 20% GelMA for up to 40 min at shear rate 5 1 s−1.
2.5. Mechanical characterization of GelMA hydrogel
The mechanical properties of crosslinked GelMA hydrogel were characterized by compressive tensile test using a dynamic mechanical analysis tester (Electroforce 3200, TA Instruments, DE). Briefly, GelMA hydrogel precursors were crosslinked and punched into disks (height = 4 mm, diameter = 6 mm). Samples were compressed between two parallel plates at the rate of 1 mm min−1. Stress-strain curves were recorded and the compressive modulus was calculated in the 10%–25% strain linear region.
2.6. In vitro degradation
Three concentrations of GelMA hydrogel precursors (5, 10 and 20% (w/v)) were crosslinked by UV light (395 nm–405 nm, 5 W) into disks (height = 4 mm, diameter = 9 mm) using cylindrical molds following swelling to equilibrium in PBS for 2 h. One group of swollen GelMA disks were immersed in 2 U ml−1 collagenase PBS solution and the other group in PBS (control) for 1, 3, 7, 14, 21,28,42, 49 and 56 d. The hydrogel disks were taken out and weighted at each data point and the percentage mass remaining was calculated. Three trials were performed for each disk sample.
2.7. GelMA biocompatibilities
Fibroblasts, at a concentration of 3 million ml−1, were encapsulated in GelMA hydrogel precursors at concentrations of 5, 10, and 20% (w/v). This was followed by UV crosslinking for 1 min. The cell-laden GelMA hydrogels were then punched into disks (6 mm in diameter and 1 mm in height) and transferred to a 96-well plate containing cell culture medium. The samples were subsequently placed in an incubator. The culture media were refreshed daily. To evaluate the metabolic activity and proliferation of L929 fibroblasts in the GelMA hydrogels, the alamarBlue cell metabolic activity assay was employed on Days 1, 3, and 7. Additionally, the cell morphologies were characterized on Days 1, 3, and 7 using cytoskeleton and nuclei staining with Phalloidin and DAPI, respectively.
2.8. Bioprinting GelMA constructs
A commercial 3D bioprinter BioMaker (SunP Biotech, USA) was used to deposit GelMA hydrogel/bioinks. The extrusion nozzle is a 26-gauge metal needle (McMaster-Carr, USA). Extrusion speed is 1 mm3 s−1 and print speed is 10 mm s−1 for all prints. For the printability study, GelMA hydrogel was printed into a two-layer construct with size 5 × 5 × 0.4 mm and filament distance of 1 mm. For cell-laden GelMA printing, a three-layer 10 × 10 × 0.6 mm construct was printed with filament distance of 1 mm. Certain temperature and holding time were applied before the GelMA bioinks reached printable physical state. The printed constructs were always immediately cured by UV light for 1 min (395–405 nm, 5 W). Cell-laden construct was placed into 6-well plates and was immersed in supplemented DMEM medium. Then the plates were moved to incubator for culturing.
2.9. Hydrogel printability and cell survivability analysis
A semi-quantitative method reported previously [35] was used to evaluate the printability of GelMA hydrogel. Briefly, the pores generated by intersecting filaments were used to measure the printability index (Pr) based on the equation:
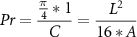
where C is the circumference of the circle, L is the perimeter of the pore and A is the enclosed pore area. Fiji software was used to process and analysis the microscopic image of the constructs. Three random pores were selected from each construct for measurement.
Cell survival was determined by evaluating the cell death induced by the shear stress during extrusion process. L929 fibroblasts at 5 million ml−1 density were encapsulated inside GelMA bioink and then were loaded in the syringe for extrusion at different temperature and time settings. GelMA precursor was treated with trypan blue dye for 1 min and then transferred to cell counting slides without crosslinking. Live/Dead cell counting was done by Countless II automated cell counter (ThermoFisher) and survivability was calculated based on the percentage of live cells.
2.10. Synthesis of MH-DS nanoparticle complexes and encapsulation in GelMA hydrogel
The fabrication method of MH-DS particle complexes was adapted from a previously published procedure [5, 8]. Briefly, solutions of DS and MH are prepared separately. DPBS without Mg2+ or Ca2+ were used as dissolving medium. DS was dissolved at 2.4 mg ml−1 in 1X DPBS buffer with Mg2+ at 14.4 mM, while MH is dissolved at 2 mg ml−1 in 1X DPBS. Equal amount of MH and DS (with Mg2+) is mixed and vigorously vortexed for 5 min. Then the particle solutions at 1 mg ml−1 MH loading concentration were prepared and ready for the next step. The solution was then used to dissolve GelMA and LAP photoinitiator (0.2% (w/v)), followed by photocrosslinking under 405 nm for 1 min (figures 5(A) and (B)).
2.11. Scanning electron microscopy (SEM)
SEM (Zeiss Supra 50VP) was utilized to characterize and visualize the internal microstructure, pores of GelMA hydrogel and the MH-DS nanoparticle complexes inside GelMA networks. Swollen GelMA samples were rapidly frozen in liquid nitrogen and then followed by lyophilization. Free-dried samples were sputter coated with Pt/Pd and observed in SEM at EHT voltage at 5 kV. ImageJ software was used to quantitatively measure the pore size distrubutions and the size of nanoparticles.
2.12. Sustained release of MH from MH-DS complexes in GelMA
Daily and accumulative release of MH from MH-DS complexes in GelMA was measured using microplate reader (BioTek Synergy HT, CA). MH-DS complexes with 1 mg ml−1 MH loading concentration was mixed with different batches of GelMA precursor and then were printed into 50 µl 4-layered constructs. The constructs were then placed in 96 well plate and filled with 100 µl fresh DPBS. 100 µl release medium were taken out and replaced every 24 h. The concentration of released MH was calculated by reading the absorbance at 380 nm and compared with a standard curve.
2.13. Study anti-oxidative properties of the released MH for cellular protection
An in vitro 2-layer oxidative stress model was printed using a dual nozzle bioprinter (Biomaker, SunP Biotech). 5% GelMA with 5 million ml−1 L929 fibroblasts were printed into 8 mm*8 mm*0.5 mm scaffold as the bottom layer. 10% (w/v) GelMA encapsulated with MH-DS complexes at the MH loading concertation of 0.15, 0.275, 0.375, 0.5 mg ml−1 were printed as 0.6 mm*6 mm*0.5 mm scaffolds respectively as topical dressing layer on top. Groups with only bottom layer scaffolds without H2O2 addition were used as the negative control group while the groups with H2O2 addition were used as positive control. All samples were then moved to 48-well plates with culturing medium supplemented with 1000 mM hydrogen peroxide to induce the oxidative stress. After culturing for 16 h overnight, the cell viability of the bottom layer constructs was measured using alarmaBlue assay.
2.14. Cell staining and fluorescence study
Fluorescent live/dead staining was used to determine the cell viability of printed construct based on the manufacturer's protocol (ThermoFisher). 2 µl Calcein AM (Component A, 4 mM) and 2 µl Ethidium homodimer-1 (Component B, 2 mM) were added to 1 ml DPBS as staining solution. 1 ml staining solution was applied on one printed construct and then the construct was incubated for 20 min. Live cells were stained with green and dead cells showed red. The construct was observed and imaged using a fluorescence microscope (Revolve, ECHO, USA). Cell morphological staining in bioprinted scaffold was characterized by cytoskeleton staining (Phalloidin) and nuclei staining (DAPI). At different stages of the culturing process, constructs were removed from the culturing medium and washed 3 times with PBS. Cells were fixed by incubation in 3.7% (v/v) PFA for 20 min at room temperature, followed by washing with PBS. Then constructs were treated with 0.5% (v/v) Triton X-100 at room temperature for 10 min, followed by washing with PBS. Phalloidin 488 was used to stain the cytoskeletons based on manufacture's protocol. Finally, constructs were mounted with mounting medium with DAPI for 5 min. Samples were then examined under a fluorescence microscope.
2.15. Statistical analysis
All the data is presented as the mean ± standard deviation. Differences between the two groups were tested by student t-test and one-way analysis of variance (ANOVA), followed by post hoc test (Dunnett's multiple comparisons test). GraphPad Prism 8.0 was used to calculate statistics. Statistical significance was determined at p < 0.05.
3. Results and discussion
3.1. Flow behavior, swelling, degradation, mechanical properties and microstructural of GelMA hydrogel
The flow behaviors and apparent viscosities were measured at different concentration and temperature conditions. Figure 1(A) showed the temperature dependent viscosities of GelMA. 5%, 10%, 20% GelMA all showed sol-gel transition induced viscosity increase when temperature decreased from 25 °C to 15 °C. GelMA with higher concentration had a more significant and faster thermo response compared with low concentration ones. Shear rate scanning of GelMA was used to evaluate shear thinning behaviors of GelMA hydrogel, which is an important property for extrusion-based bioprinting. All groups showed prominent shear thinning properties as the viscosities decrease at increasing shear rate (figure 1(B)). Figure 1(C) showed the increased viscosities of GelMA with respect to time at 21 °C. It was observed that the viscosities from all groups kept increasing until 40 min, indicating the time-dependent thermoresponsive process. Temperature-induced sol-gel transition is a time dependent process as the GelMA network transforms from random coil to triple-helix when the temperature went below sol-gel transition temperature. The transition from sol to gel in GelMA is not instantaneous while occurs over a period of time. Factors that affect he kinetics of such process include the concentration of GelMA, additives existence, and cooling rate. The time taken for complete gelation can vary from minutes to hours depending on these factors. Swelling behaviors of GelMA were characterized in figure 1(D). 5%, 10%, and 20% GelMA had a mass swelling ratio of 1523.93 ± 87.43%, 760.25 ± 59.21%, and 403.73 ± 20.34%. Because of the loosely crosslinked network, GelMA at lower concentrations tends to have higher swelling ratio, indicating better water absorption, nutrient transport, and drug delivery abilities.
Figure 1. Material characterization of 5%, 10%, 20% GelMA hydrogels. (A) Temperature dependent dynamic viscosities of GelMA. (B) Shear thinning properties of GelMA at shear ramping from 1 to 100 1 s−1 . (C) Time dependent dynamic viscosities of GelMA. (D) Swelling ratio of GelMA hydrogel. Degradation of crosslinked GelMA in PBS (E) and collagenase (F). (H) Morphology of GelMA network and pore size distributions of (I) 5%, (J) 10%, (K) 20% GelMA. Scale bar = 10 µm (500×) and 200 nm (20 000×).
Download figure:
Standard image High-resolution imageIn the fields of drug delivery and tissue engineering, the biodegradability of hydrogel materials has become increasingly important. Specifically, GelMA hydrogels exhibit enzymatic degradation characteristics, akin to their precursor substances, gelatin and collagen. GelMA contains enzyme-sensitive sequences, notably proline-X-glycine-proline, where 'X' represents a neutral amino acid. This study evaluated GelMA's stability under physiological conditions by studying its degradation in PBS and collagenase. The results showed that both 10% and 20% GelMA maintained excellent stability for up to 60 d, retaining over 90% of their mass. In contrast, 5% GelMA displayed a significant mass loss, with a half-life of approximately 45 d (figure 1(E)). Furthermore, GelMA's degradation in collagenase was rapid, with lower concentrations degrading more quickly. Specifically, 5% GelMA completely degraded by day 2, whereas 10% and 20% GelMA required 7 d and 11 d, respectively, to fully degrade.
Unconfined compression tests were conducted to evaluate the GelMA hydrogel at different concentrations and crosslinking times (figure 1(G)). The compressive modulus was calculated from the stress-strain curve between 10%–25% strain linear region. It was observed that 5% and 10% GelMA reached complete photocrosslinking in less than 60 s as the compressive modulus reached plateau, while 20% GelMA reached maximum modulus at around 90 s. The compressive modulus of 5% GelMA reached 35.65 ± 3.13 kPa, and 116.11 ± 5.23 kPa for 10%, and 370.18 ± 7.04 kPa for 20%. To further explore the microstructures of GelMA hydrogel, SEM images of GelMA were acquired at high (500×) and low (200×) magnification (figures 1(H) and (I)). Average pore size for 20%, 10% and 5% GelMA were 25.75 ± 5.67 µm, 51.91 ± 11.60 µm, and 110.33 ± 20.43 µm, respectively. Lower concentrations had a larger pore size, indicating higher crosslinking density and stiffer network. Nonetheless, the actual pores of freeze-dried GelMA sample visualized under SEM can vary from the freezing temperature and may not reflect the actual pore size of GelMA hydrogel at wet state.
3.2. Biocompatibilities of GelMA
L929 fibroblasts were encapsulated GelMA hydrogels (5%, 10%, 20%) at the density of 3 million ml−1 for 3D culturing. Cellular metabolism activities were recorded for a period of 7 d. Based on the alarma blue assay, cellular metabolism in 5%, and 10% groups steadily increase over a period of 7 d. While the activities were significantly higher at 5% GelMA, compared with 10% and 20%. Cellular activities in 20% GelMA were extremely low, indicating incompatible for 3D culturing (figure 2(A)). The cellular morphologies on day 1, 3 and 7 were visualized by DAPI/Actin staining. Results were shown in figure 2(B). At day 3 of 5% and 10% GelMA group, many cells have transitioned from a spherical form, indicating initial attachment, to a more complex morphology characterized by the emergence of branches (indicated by red arrows), suggesting the initiation of dynamic cellular processes and adaptation to the culture environment (figure 2(C)). On day 7, there are less branched and more elongated cells, suggesting maturation of fibroblasts (figure 2(D)). Fibroblasts in 20% GelMA showed a significant decrease in number and spherical morphologies compared with lower concentrations, indicating inhibited proliferation and functionality. The ability of cells to live and function in hydrogels is a basic requirement for subsequent tissue regeneration. GelMA provides a supportive 3D environment that can mimic the natural ECM, providing a favorable environment for the growth and differentiation of various cells. The bioactive motifs in GelMA (due to its origin from collagen) can also support cell adhesion and proliferation [21, 22].
Figure 2. Biocompatibility study of GelMA hydrogels with L929 fibroblasts. Red arrows indicate branched morphologies of L929 in GelMA (A) Biocompatibility study of GelMA hydrogels with L929 fibroblasts. (A) L929 cellular metabolic activities in 5%, 10%, and 20% GelMA cultured for 1, 3 and 7 d (***, p < 0.001, *, p < 0.05 compared with 5% group). (B) Actin filament and nuclei staining using DAPI (blue) and phalloidin (green) to evaluate the L929 fibroblast morphology. Scale bar = 70 µm. Branched L929 morphologies that were observed in 5% GelMA on (C) Day 3 (Scale car = 70 µm) and (D) Day 7 (Scale bar = 30 µm).
Download figure:
Standard image High-resolution image3.3. Printability of novel GelMA bioinks
Due to the thermoresponsive and photocrosslinking abilities of GelMA, a two-step crosslinking strategy was adopted in this research (figure 3). GelMA bioinks were physically crosslinked first to form extrudable gels. Based on the sol-gel transition state of physically crosslinked GelMA, the extruded GelMA bioink demonstrates uncontrollable, continuous and controllable, irregular or unextrudable filaments, corresponding to under-, proper- and over- physical gelation. Printed scaffolds were then photocrosslinked upon UV irradiation to form permanent structure. A semi-quantitative method was adopted to evaluate its ability to maintain structure fidelity [35]. While other criteria like filament fusion, sagging, and filament uniformity are also relevant for assessing printability [9], this study primarily focused on the 'pore factor' of the printed constructs to simplify the construction of an optimized process. In this context, 'Pr' scores below 0.9 were indicative of under-gelation, scores above 1.1 suggested over-gelation, and scores within the 0.9–1.1 range signified proper gelation. Additionally, cell viability was measured immediately post-printing to evaluate potential cellular damage incurred during the extrusion process, with viability defined as the percentage of surviving cells relative to the total encapsulated cells.
Figure 3. Diagrammatic representation of the two-step crosslinking process in GelMA bioprinting, accompanied by criteria for visual and semi-quantitative evaluation, and methodology for assessing process-induced cell viability.
Download figure:
Standard image High-resolution imageThe testing range of time and temperature parameters for printing with GelMA hydrogels were meticulously determined. The holding/printing time range of 0–40 min was set based on the rheological behavior of 5% and 10% GelMA hydrogels. Specifically, their rheology profiles indicated a plateau phase occurring approximately between 35 to 40 min. Furthermore, cell viability studies using the GelMA bioinks revealed a reduction in cell viability after 30 min, as demonstrated by trypan blue assay results (refer to figure S3). Besides, previous studies have reported that extrusion processes in most applications typically occur within 40 min [22, 35]. The temperature range was determined based on GelMA's viscosity-temperature profile. The upper limit was set slightly above the sol-gel transition temperature, while the lower limit was established at 10 °C, which is the operational minimum of our SunP BioMaker printer.
3.3.1. GelMA printability at difference concentration with respect to printing time and temperature
Due to its lack of biocompatibility, 20% GelMA was not further optimized in terms of printability and cell viability. Instead, printability tests were focused on 5% and 10% GelMA concentrations, under various temperature and holding time conditions. In this context, 'temperature' refers to the setting of the printing nozzle, while 'holding time' denotes the duration for which the bioink remains in the nozzle. Holding time is also a critical factor in determining the total printing duration the bioink can withstand before undergoing over-gelation. This aspect is particularly crucial for printing constructs with substantial thickness and high aspect ratios. The Pr index was plotted in a 3D space using MATLAB's surf () function. The x and y axis represent temperature and time, respectively. The results can be seen from figure 4. Two hyperplanes were plotted on the surface plot to use as boundaries for the good printability range (0.9 < Pr < 1.1). For both 5% and 10% GelMA hydrogels, as the nozzle temperature decreases from above sol-gel transition temperature to below, the printability index (Pr) increases from below 0.9 to greater than 1.1, which indicates the GelMA hydrogel experience from under-gelation, proper gelation and to over gelation conditions. Similar trends were found in the effect of time. With longer holding time of GelMA bioink inside the printing nozzle, the printed scaffolds have higher Pr (figures 4(A) and (G)). For 5% GelMA, the bioinks are extrudable at all the temperature ranges (10 °C–21 °C) and time range (5–40 min) and fail to form physical gels above 22 °C at any holding time (figure 4(B)). For 10% GelMA, the bioinks become unextrudable when held for 5 min at 15 °C. Additionally, at 24 °C, they fail to form physical gels regardless of the holding time (figure 4(H)). The temperature and time dependent printability index of GelMA bioink is owing to its the sol-gel transition properties. GelMA polymer chains start to transform from random coil to triple helix when the temperature decreases blow its sol-gel transition temperature, leading to physical gelation into extrudable hydrogels [36]. However, the thermogelation of GelMA is a time-dependent process, extended gelation time may lead to over-gelation (Pr > 1.1) of GelMA and yield low printability scaffold. Similarly, lower temperature may speed up the gelation process of GelMA and lead to over-gelation.
Figure 4. Construction of optimal printing zones based on printability and cell viability. 3D printability index as a function of time and temperature for (A) 5% GelMA and (G) 10% GelMA. 2D projection of printability of (B) 5% GelMA and (H) 10% GelMA onto temperature and time space. Yellow color represents over-gelation (Pr > 1.1), blue represents proper gelation (0.9 < Pr < 1.1) and green represents over-gelation (Pr < 0.9). 3D cell viability index as a function of time and temperature for (C) 5% GelMA and (I) 10% GelMA. (D) 2D projection of cell viability index of 5% GelMA onto temperature and time plane. Gray area represents viability greater than 80%. (J) 2D projection of cell viability index of 10% GelMA onto temperature and time plane. Blue area represents viability greater than 80%. Merged optimized printing zones of (E) 5% and (K) 10% GelMA. Live/Dead staining of (F) 5%, and (L) 10% bioprinted GelMA scaffold using the parameters selected from shaded merged area (I) and (J) (black dots). Scale car = 670 µm.
Download figure:
Standard image High-resolution image3.3.2. Cell survivability at different concentrations with respect to holding time and temperature
Cell viability right after extrusion printing process was evaluated for 5% and 10% GelMA under the same time and temperature conditions as printability test. The results are shown in figures 4(C), (D), (I) and (J). Cell viability was immediately measured with trypan blue assay without photocrosslinking into hydrogel, excluding the effect of UV light and free radicals on the cell damage. For all test groups, cell viabilities decreased with longer printing times and lower nozzle temperatures. This trend aligns with the time and temperature-dependent rheological properties of GelMA. As indicated in figures 1(A) and (C), GelMA exhibits higher apparent viscosities at longer gelation times and lower gelation temperatures, which may contribute to the observed decrease in cell viability. During extrusion-based bioprinting, cells are subjected to various mechanical forces. Inside the nozzle, shear stress is generated in the viscous bioink, which is considered as the primary contributor to potential disruption of cell membranes or cell damage and death (figure 3). Shear stress is dependent on several factors including extrusion pressure, speed, cell density and the viscosities of bioinks [12]. Notably, a highly viscous bioink, while favorable for generating high-quality printability filaments, can induce elevated shear stress during extrusion. This study specifically investigated the impact of two principal factors influencing GelMA bioinks' viscosity, and their consequent effects on cell survival during extrusion bioprinting.
80% viability threshold planes were constructed to map the appropriate printing range in which cells have appropriate viability for postprinting usage. Then based on the printability map (figures 4(B) and (H)) and cell survival map (figures 4(D) and (J)), we merged them and yielded the optimal printing parameter range for 5% (figure 4(E)) and 10% GelMA (figure 4(K)). Therefore, we were able to select from the hatched optimal area and yield the cell-laden scaffold with good structural printability (0.9 < Pr < 1.1) as well as cell viability (>80%). We randomly picked a previously untested datapoint in the hatched area from the optimized map of 5% (20.8 °C, 24 min) and 10% (21.5 °C, 10 min), and then conducted cell printing of a two-layer scaffold. Based on the LIVE/DEAD staining, the scaffold showed good cell viability (∼90%) and good structure fidelity as shown in figures 4(K) and (J).
3.4. Controlled release of MH from MH-DS nanocomplexes in GelMA
As previously reported [5], DS exhibits a high binding affinity for divalent metal ions. At the same time, MH is capable of chelating these ions, thereby facilitating an interaction between DS and MH through metal ion binding. During complex formation, which occurs at a pH range of 5.5–6.5, MH adopts a zwitterionic form due to the deprotonation of its hydroxyl group and the presence of an ammonium group. This zwitterionic state enables MH to engage in electrostatic interactions with DS's negatively charged sulfate groups, as well as potential hydrogen bonds with DS's hydroxyl, carbonyl, and amide groups [8]. MH-DS complexes were encapsulated within GelMA, and the resulting crosslinked printing constructs are depicted in figure 5(B). Subsequently, GelMA loaded with MH-DS particles underwent lyophilization to prepare for (SEM) imaging, as shown in figure 5(C). The SEM images revealed that the nano-sized MH-DS particles aggregated into clusters, which were adhered to the crosslinked GelMA polymer network. The size of these nanoparticles ranged from 50 to 120 nm.
MH-DS complexes encapsulated in different concentrations of GelMA were explored, with MH directly mixed into GelMA serving as a control (figures 5(C) and (D)). Our observations indicated that MH, when incorporated as MH-DS complexes, showed a significantly reduced burst release within the first 24 h. This was followed by a prolonged release period of up to 22 d across all tested GelMA concentrations. In contrast, MH incorporated directly within GelMA exhibited a release lasting merely 3–4 d. Quantitatively, the burst release percentages for the directly integrated MH ranged from 38.1% to 43.2%, depending on the GelMA concentration. For the MH-DS complexes, this range was significantly reduced to 13.5%–17.3%. Notably, MH-DS complexes demonstrated reduced burst releases in lower GelMA concentrations, a trend that was inversely observed with direct MH mixtures. This observed behavior could be attributed to the interactions between MH-DS complexes and the charged networks within the GelMA. At higher GelMA concentrations, the MH-DS complexes likely experience increased interactions with both negatively and positively charged components of the GelMA network [37]. Such enhanced interaction between MH-DS and GelMA network may facilitate the dissociation of the nsnoparticle complexes, accelerating the release of MH from MH-DS. The release kinetics of MH-DS encapsulated in 5% and 10% GelMA concentrations were observed to follow similar profiles throughout the study period. Notably, MH-DS complexes in these concentrations almost achieved a complete cumulative release, with 99.84 ± 4.25% for 5% GelMA and 99.60 ± 5.53% for 10% GelMA. In contrast, the 20% GelMA formulation showed a lower cumulative release of 84.99 ± 9.41%. These figures stand in contrast to the directly incorporated MH formulations, which demonstrated significantly lower cumulative releases: 54.31 ± 4.32% in 5% GelMA, 47.55 ± 3.61% in 10% GelMA, and 41.69 ± 4.66% in 20% GelMA.
Figure 5. MH-DS preparation, characterization, and release behavior study in GelMA hydrogels. (A) Preparation of MH-DS particle complexes and encapsulation in GelMA hydrogel. Scale bar = 1350 µm. (B) SEM images of MH-DS particles in 5%, 10%, and 20% GelMA hydrogel. Scale bar = 20 µm (500×) and 200 nm (20 000×). (C). Daily release of 5%, 10%, and 20% GelMA for MH-DS and direct mixing. (D) Accumulative release of MH in 5%, 10%, and 20% GelMA for MH-DS and direct mixing.
Download figure:
Standard image High-resolution imageLiterature relating to using GelMA to deliver MH has been mostly on direct mixing [38, 39], our findings indicate that this approach results in high burst release and lacks sustained-release properties. In addition, the significantly reduced accumulative release from direct mixing groups may account for the oxidation from free radicals residuals of GelMA photocrosslinking. In other words, MH in the MH-DS complexes form was protected from the attack of free radicals during chemical crosslinking, which is supported by the observation that the unencapsulated MH in GelMA started getting oxidized and turned black on day 2 of incubation (figure S4).
3.5. Evaluating the in vitro cellular protection properties of MH for L929 under oxidative stress
The oxidative stress-induced apoptotic model was constructed using the method detailed in section 2.13. Employing the optimal printing conditions identified in previous sections, we achieved a cell viability of 96.12 ± 3.23% in the bottom layer of the model, which served as the negative control. The results were shown in figure 6. After 16 h of incubation with oxidative stress induced by 1000 mM hydrogen peroxide, the viability of L929 cells was found to be 44.93 ± 10.9% (positive control). Notably, the group with a MH loading concentration of 0.375 mg ml−1 exhibited the highest cell viability, reaching 71.6 ± 9.8% (p = 0.0107), representing approximately a 60% increase compared to the positive control group. In contrast, cell viability at MH loading concentrations of 0.5 mg ml−1, 0.275 mg ml−1, and 0.15 mg ml−1 were 32.45 ± 6.16%, 56.90 ± 2.29%, and 47.03 ± 8.96% respectively (figures 6(C) and (D)). These values did not differ significantly from the positive control group, according to the results of the post hoc multi-comparison test (p-values of 0.3496, 0.3845, and 0.9983, respectively). H2O2 is one of the cytotoxic ROS that can increase dramatically at times of environmental stress such as injury and inflammation. MH has been shown to directly scavenge ROS, neutralizing them before they can cause cellular damage [4, 6], which may account for the increasing cell viability when the MH-DS loading concentration increases from 0.15 mg ml−1 to 0.375 mg ml−1 . However, an increase in loading to 0.5 mg ml−1 results in decreased cell viability, which is not significantly different from the positive control group. This reduction in viability at higher concentrations can likely be attributed to the cytotoxic properties of MH [29]. Specifically, when the concentration of released MH exceeds a certain threshold, it can become cytotoxic and lead to cell death under these experimental conditions and with this cell type.
Figure 6. In vitro model to evaluate MH to protect cells under oxidative stress. (A) Diagram of in vitro model printed using dual-nozzle extrusion bioprinter. The top layer was printed with MH-DS complexes in GelMA, bottom layer consisting of fibroblasts at 5 million ml−1 . Oxidative stress (1000 mM H2O2) was introduced at the start of model incubation. (B) CAD and slicing of model design, and images of the printed dual layer model. Scale bar = 1870 mm. (C) Cell survival rate after incubation at different conditions. (NS p > 0.05, * p < 0.05, ** p < 0.01, compared with positive control). (D) Live staining of bottom layer cell-laden scaffold after incubation period. Scale bar = 500 µm (4×), 130 µm (10×).
Download figure:
Standard image High-resolution image4. Conclusion
In this study, we have comprehensively characterized GelMA's time and temperature-dependent flow behaviors, examining their effects on printability and cell survivability in extrusion bioprinting. We successfully established an optimized printing window for GelMA bioink by leveraging its time dependent thermoresponsive properties, with selected parameters from this zone yielding high-fidelity scaffolds and enhanced cell viability. Moreover, we demonstrated the effective incorporation of MH-DS nanoparticle complexes into GelMA, facilitating controlled and sustained drug release in bioprinted scaffolds. This resulted in the released MH providing significant protection to fibroblasts under oxidative stress. Our findings underscore the versatility of GelMA bioinks in various applications, such as 3D printed wound dressings, implantable scaffolds, and in vitro disease models, showcasing its potential as a multifaceted platform in biomedical engineering.
Acknowledgments
We acknowledge the financial support from SunP Biotech company research Grant Drexel—260676.
Data availability statement
All data that support the findings of this study are included within the article (and any supplementary files).
Conflict of interest
Authors declare that they have no competing interests.
CRediT authorship contribution statement
Zhouquan Fu: Methodology, Investigation, Writing—original draft, Writing—review & editing, Visualization. Nan Hai: Investigation. Yinghui Zhong: Conceptualization, Writing—Review & Editing. Wei Sun: Conceptualization, Funding acquisition, Writing—Review & Editing.
Supplementary data (1.3 MB DOCX)