Abstract
We present a dynamically tunable metamaterial graphene absorber (MGA) in the terahertz regime. The unit cell of the proposed MGA consists of metal wire and graphene sheet over the grounded dielectric absorber. The MGA achieves frequency tunable characteristics via changing the chemical potential. In order to understand the absorption mechanism of this absorber, a simple equivalent circuit method has been proposed. Because the coupling between wire-based metamaterial and graphene is complicated and cannot be neglected an equivalent surface impedance was introduced and extracted for simplification. In addition to the chemical potential of graphene, the constitutive parameters of metal wire are also discussed in detail to completely understand how these factors affect the absorption properties. It is believed that this study may be useful for providing valuable guidance in the development of more advanced MGAs.
Export citation and abstract BibTeX RIS
1. Introduction
Recently, extensive research has been carried out on the design and fabrication of cloaks from microwave to optical frequency due to their potential applications in stealth technology [1–5]. Metamaterial absorbers (MAs), as a kind of cloaking device, have provoked extensive interest, and a variety of metamaterial structures have been designed [6–9]. With these kinds of novel absorbers, theoretical unity absorptivity can be achieved by matching the impedance of MA to the free space [10, 11]. However, once the structure of MA is fabricated, the position and magnitude of the absorption peak is fixed. Therefore, more and more attention has been focused on the design of tunable MAs. Graphene, a single atom carbon layer arranged in a honeycomb lattice, has attracted a great deal of attention due to its intriguing properties in photonics and optoelectronics [12]. Meanwhile, because of its high electron mobility, unique doping capabilities, and tunable conductivity by bias voltage, the absorbers based on graphene can be tunable in the THz and optical regime [13, 14], which are important properties in some applications. However, the general principles of selecting structure parameters for the desired MGAs have not been discussed. For engineers, it is more important to understand the relationship between the chemical potential, structure parameters, and the absorption properties. More recently, an ultra-broadband multilayered graphene absorber has been studied in [15]. However, there is a lack of general strategy to analyze the absorption frequency of the ultra-broadband from its transmission line model.
Wire-based metamaterial or graphene ribbon absorber, as simple but versatile artificial structures, have been widely investigated in previous literature (e.g. [16–21]). At the same time, some theoretical approaches, such as effective surface conductivities through a sheet retrieval method [17], transmission line method [20, 22], accurate analytical model [23], effective medium theory [24] and simulation analysis method [25, 26] have been exploited to explain the physics mechanism. When the metamaterial is in close proximity to a graphene sheet, however, to the best of the authors' knowledge, little attention has been paid on discussing the equivalent impedance of this whole in previous literature.
In this paper, a wire-based metamaterial and graphene absorber is proposed in which a periodic array of metal wire is placed on a graphene sheet. The absorption spectra can be shifted dynamically by modulating the chemical potential of graphene. We use the retrieved equivalent impedance of wire-based metamaterial and graphene to analyze the mechanism. Meanwhile, an equivalent circuit model is applied to further reveal the influences of the constitutive parameters on the absorption properties for this proposed MGA.
2. Design and simulation
The schematic of the unit cell of the studied MGA is illustrated in figure 1. The metal wire is separated from the bottom gold plate by an ultrathin graphene and insulator dielectric substrate. The gold is made of lossy metal with an electric conductivity of σ = 4.56 × 107 S m−1 and the thickness is 0.1 µm. The real part of the dielectric constant of the dielectric spacer is 11.9, and the thickness is t = 3 µm. The other dimension parameters are fixed to be p = 10 µm, l = 9 µm and w = 1.2 µm. All of these geometrical sizes are carefully designed to acquire the optimal effect.
Figure 1. Schematic diagram and geometric parameters of the MGA unit cell with polarization and propagation direction of the incident wave.
Download figure:
Standard image High-resolution imageFor a graphene sheet, the surface conductivity of graphene σg can be given by the Kubo formula [27]. When there is no external magnetic field present, the graphene exhibits isotropy. In this case, the surface conductivity can be approximated as follows for kBT μc,
:
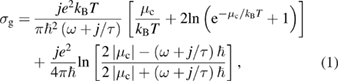
where kB is the Boltzmann constant, e is the electron charge, ℏ is the reduced Planck constant, T is the temperature, μc denotes the chemical potential, ω is the angular frequency, and τ is the relaxation time, respectively. All simulations are performed in T = 300 K, the thickness of graphene sheet is 1 nm and τ = 0.1 ps throughout this study. The surface impedance of a graphene sheet can be expressed by Zg = 1/σg.
Performance simulations of this MGA were demonstrated using the full-wave electromagnetic simulation software CST Microwave Studio. The incident electric field vector is parallel to the metal wire, and the magnetic field vector is perpendicular to the metal wire. Because the bottom gold plate prevents any transmission of terahertz radiation, the absorption properties of this MGA are characterized by reflection coefficients in this work.
The frequency tenability of graphene absorber is an important characteristic in practical application. In order to explore the tunable effects of this proposed MGA, we first merely vary the chemical potential of graphene sheet. Reflection coefficient spectra for different chemical potential are represented in figure 2. At normal incidence. At figure 2(a), one can observe a progressive reduction and blue shift of the reflection peaks with increasing μc from 0 to 0.4 ev. The peak positions shift from 6.91–8.1 THz, and the reflection peaks continuously decrease from −39.7 dB to −7.24 dB. To clearly display these relationships, figure 2(b) shows the dependence of the relationship among chemical potential, reflection coefficient and absorption frequency. The reason for this behavior will be elucidated in the next section. From these results, we can see that graphene plays an important role in achieving the tunable function for this MGA. Meanwhile, the properties of the proposed MGA structure can be modulated conveniently by controlling the graphene's chemical potential.
Figure 2. (a) Reflection coefficient at μc = 0, 0.1, 0.2, 0.3 and 0.4 ev, (b) absorption frequency and chemical potential μc as function of reflection coefficient.
Download figure:
Standard image High-resolution image3. Results and discussions
We first use a transmission line model to investigate this proposed absorber. As show in figure 3(a), Zt is the characteristic impedance of the dielectric substrate. Zmg presents the effective impedance of the metamaterial-graphene (MG) film. The gold ground plane is considered as a short circuit in the transmission line model analysis due to its zero transmission. (Although it is exact only for perfect electric conductors, it is accurate enough in the terahertz regime as long as the metal backplane is sufficiently thick.) The impedance of the grounded substrate can be expressed as [28–30]:

where Z0 is the wave impedance in the free-space and is equal to 376.7 Ω, is the free space propagation constant, t is the thickness of the dielectric,
and
are real and imaginary parts of the relative dielectric constant of the dielectric material. The effective surface impedance of the ground substrate is depicted in figure 3(b). It can be seen that at 7.22 THz the imaginary part of Z1 is zero, indicating resonance occurring at near this frequency when there only exists gold-backed silicon dielectric substrate.
Figure 3. (a) Transmission line model of this absorber, (b) effective surface impedance of the ground dielectric.
Download figure:
Standard image High-resolution imageIf Zmg is calculated, the input impedance of the absorbing structure Zin is equal to the parallel connection between the MG impedance Zmg and the complex surface impedance of the grounded dielectric slab Z1 [31],

Finally, the reflection coefficient can be expressed as:

So in the following section, we should first calculate the value of Zmg.
4. Mechanism analysis
To better understand the physics mechanism of the reflection and explain quantitatively the relationship between the frequency shift and chemical potential, the effective impendence of the MG sheet must be established first. Theoretically calculating the impedance of MG sheet is much more difficult since the mutual coupling between the metal wire and graphene sheet. There are several methods to determine the effective surface resistance of the thin layer [24, 32, 33]. Here, we retrieved the effective impendence of the MG sheet Zmg from the simulated reflection coefficient S11 [32]:

where the admittance of free space, Y1 is the intrinsic admittance of the dielectric spacer, and n is the refractive index of the dielectric spacer.
The retrieved effective surface impedances Zmg from S-parameters are plotted in figures 4(a) and (b). Referring to figure 4(a), it can be seen that the magnitude of the real part of Zmg decreases at first and then increases with the augment of . Figure 4(c) shows this relationship intuitively. By comparing figures 2(b) and 4(c), one can find that the absorption frequency of MGA shows a similar exponential increase with the increase of μc. However, comparing the reflection coefficient to the impedance Zmg of MG sheet, it can also find that when the real part of Zmg decreases from 717.77 Ω to 213.4 Ω, the reflection coefficient increases from −38 dB to −7.29 dB. Therefore, we can confirm that the absorption frequency and absorptivity were determined by the effective impendence Zmg, which was tunable by the chemical potential.
Figure 4. The real (a) and imaginary (b) parts of the retrieved effective surface impendence Zmg, and (c) resonance frequency and chemical potential μc as function of real Zmg.
Download figure:
Standard image High-resolution imageIn order to explain how the constitutive structure parameters affect the reflection properties, an equivalent circuit model (shown in figure 5(a)) was utilized, which is equivalent to figure 3(a). The inductance L is determined primarily by the parallel wire, capacitance C is created between the wire and the bottom gold plate. In addition, Rm, Rg and Rt were caused by the metal wire, graphene sheet and dielectric losses, respectively [16]. Compared with the thickness of the substrate, the thickness of graphene sheet is ultrathin. For simplicity, Rm and Rg can be considered as a whole, represented by Rmg. The inductance L and capacitance C are presented by and
(shown in figure 5(b)). As a matter of fact, it is difficult to obtain the accurate analytical formulations of inductance
and capacitance
.
Figure 5. (a) Equivalent circuit model of the MGA, and (b) simplified circuit model.
Download figure:
Standard image High-resolution imageFrom this equivalent circuit model, the absorption frequency can be expressed as [16]:

Due to , so the term of
can be neglected in equation (6). Furthermore, the inductance
can be approximated by the formula of a parallel capacitor as
, and the inductance L as
[16, 34, 35], so the absorption frequency from equation (6) can be given by:

From relation (7), we can find that the absorption frequency is related to the length l, width w, permittivity , loss Rmg and thickness t. In this study, the influence of spacer permittivity
, loss Rmg and thickness t were not discussed. We first investigated the relationship between length l and absorption frequency. Figure 6(a) plots the reflection coefficient for various wire lengths when chemical potential is set at 0 ev and w, t,
at the optimized value. It is clear that the absorption peak shows the trend of first decreasing and then increasing. Meanwhile, as the wire length l decreases, the absorption frequency experiences a blue shift, displaying an inversely proportional relationship as indicated by equation (7). Figure 6(b) shows the dependence of the absorption frequency on the length of wire clearly.
Figure 6. (a) Simulated reflection coefficient of the MGA at l = 5, 6, 7, 8, 9 µm. (b) The first and second absorption frequencies as a function of the wire length l. Dashed line denotes the dependence of the wire length for the absorption frequency.
Download figure:
Standard image High-resolution imageFinally, the influence of the width on the absorption frequency was also investigated at normal incident case and the results are presented in figure 7(a). Here, we have varied the width w from 0.8 µm to 1.2 µm. It can be found that the absorption frequencies show continuously red shift with increasing the width w. These results agree well with the conclusion of equation (7). In order to clearly display this relationship, figure 7(b) shows this dependency.
Figure 7. (a) Simulated reflection coefficient of the MGA with various wire width. (b) The absorption frequencies as a function of the wire width w. (Dashed line denotes the dependence of the wire width for the absorption frequency.)
Download figure:
Standard image High-resolution image5. Conclusions
In conclusion, we have investigated a frequency-tunable terahertz absorber based on wire-based metamaterial and graphene. The influence of the MG surface resistance on the chemical potential and absorption frequency has been studied in detail. Meanwhile, the wire length and width were discussed by using an equivalent circuit model. With this transmission line model and equivalent circuit model, it can be easy to understand the physical mechanism. The most important thing is that this will provide valuable guidance on how to develop more advanced MGAs in the future.
It should be noted that only the proposed method was verified in this model. Therefore, in our future work, we will focus on the experimental verification of the acquired numerical results by measuring fabricated prototypes. In addition, the dependence of the absorption frequency on the structural parameters will also be explored in other absorbers.
Acknowledgments
This work was supported by the National Natural Science Foundation of China (Grant Nos. 61501067, 61661012), Foundation and Advanced Research Projects of Chongqing Municipal Science and Technology Commission (Grant Nos. Cstc2016jcyjA0377), and Opening Project of Guangxi College Key Laboratory of Microwave and Optical Wave Applications Technology (Grant Nos. MLLAB2016001).